ABSTRACT
Cu/Ta2O5/Pt and Cu/SiO2/Pt are two of the most promising resistance switches. From experimental observations, it is speculated that the presence of H2O in the amorphous Ta2O5 and SiO2 (a-Ta2O5 and a-SiO2) facilitates the rate-limiting step during the switching process. This rate-limiting step is essentially the diffusion of Cu ions along the nanopores of the amorphous. To better understand this behavior and obtain a detailed examination of the atomic structures, a first-principles simulation was conducted. In addition, we investigate the diffusion behaviors of Cu ions in bare a-Ta2O5 nanopore and in the one covered with H2O–together with those in a-SiO2 nanopore. Our work reveals that Ta and Si atoms on the sidewalls of bare a-Ta2O5 and a-SiO2 nanopores are in the unsaturated (TaO5) and saturated (SiO4) forms, respectively. Consequently, H2O molecules are adsorbed on the nanopore sidewall strongly in the case of a-Ta2O5, and weakly in a-SiO2, by forming O-Ta and H∙∙∙O bonds, respectively. This can explain the experimental observation that the desorption of H2O occurs only at high temperatures for a-Ta2O5 films, while it is observed for a-SiO2 even when the temperature is low. The calculated diffusion barrier of Cu ions in a-Ta2O5 nanopores covered with H2O is about 0.43 eV, which is much lower than that without H2O (~1.40 eV). In view of the similar chemical environments of O and the adsorbed Cu ions in a-SiO2 and a-Ta2O5 nanopores, it is expected that the diffusion of Cu ions in a-SiO2 nanopore without H2O is much more difficult than with H2O. This could be attributed to the strong and weak adsorption of Cu ions on the sidewall in the absence and presence of H2O, respectively, for both, a-Ta2O5 and a-SiO2. Our investigation provides a full atomic picture to understand the moisture effect on the diffusion of Cu ions in Cu/a-Ta2O5/Pt and Cu/a-SiO2/Pt resistance switches.
Graphical Abstract

1. Introduction
Cation-based resistance switches or electrochemical metallization memories (ECM) have attracted significant attention due to their high scalability, low power consumption and potential application in memory cells [Citation1,Citation2]. In general, such devices consist of an insulator (such as, HfOx [Citation3], SiOx [Citation4], TaOx [Citation5], etc.) layer sandwiched between an inert electrode (Pt or Au) and an oxidizable electrode (Cu or Ag). Their switching processes (from high resistance state to low resistance state) could be realized by changing the polarity of the applied voltage between the electrodes.
Among the ECM devices, amorphous Ta2O5 and SiO2 (a-Ta2O5 and a-SiO2)-based devices (i.e. Cu/a-Ta2O5/Pt and Cu/a-SiO2/Pt) have been extensively studied considering their high performances [Citation5,Citation6]. Composition analyses of a-Ta2O5 and a-SiO2 films before and after applying a voltage reveal that the switching phenomenon is mainly due to the formation/rupture of Cu filaments [Citation7,Citation8]. Recently, the moisture effects on the performance of a-Ta2O5- and a-SiO2-based ECM devices have been studied experimentally [Citation6,Citation9]. The following are the key outcomes: (i) none of the devices could be formed without the presence of H2O, (ii) the forming voltage drops dramatically with increase in the content of H2O in a-Ta2O5 and a-SiO2 films, (iii) the operation voltage of a-Ta2O5 (a-SiO2)-based ECM device rarely (strongly) depends on the ambient H2O pressure.
During the switching processes of Cu/a-Ta2O5/Pt and Cu/a-SiO2/Pt ECM devices, three rate-limiting steps have been proposed in the literature. These are (i) ionization of Cu electrode at the Cu/a-Ta2O5 and Cu/a-SiO2 interfaces, (ii) diffusion of Cu ions in the a-Ta2O5 and a-SiO2, and (iii) nucleation of Cu ions at the Pt electrode. These key factors determine the performance of ECM devices (such as, the forming and operation voltages, endurance and switching rates). Accordingly, the ‘moisture effect’ on the performance of ECM devices can be attributed to the influence of the above-mentioned rate-liming steps due to the presence of H2O [Citation6,Citation9–Citation11]. In particular, moisture has been found to be important in the processes of diffusion and resistive switching (e.g. the counter electrode reaction) [Citation10,Citation11]. Studies on moisture effect in such processes remain to be done. In the present work, we pay due attention on the ‘moisture effect’ on the diffusion of Cu ions in ECM devices. The following hypothesis is proposed on the basis of experimental observations: the nanoporous structures of the deposited a-Ta2O5 and a-SiO2 films have strong and weak moisture adsorption characteristics during ambient exposure [Citation12,Citation13], respectively. The Cu diffusion along the sidewalls of a-Ta2O5 and a-SiO2 nanopores becomes smoother when the nanopores are covered with H2O. The strong dependence of the operation voltage on the ambient H2O pressure in the case of a-SiO2 can be understood from weaker adsorption of H2O on the sidewall of a-SiO2. However, several issues need to be addressed: (i) what is the atomic structures of a-Ta2O5 and a-SiO2 nanopores, (ii) why is the adsorption behavior of H2O in a-Ta2O5 and a-SiO2 nanopores significantly different, (iii) how the Cu ions diffuse along the a-Ta2O5 and a-SiO2 nanopores with and without the presence of H2O. Accordingly, the atomic-scale simulations on the structures of a-Ta2O5 and a-SiO2 nanopores, and the Cu diffusion behaviors in these nanopores would be very helpful not only to understand the switching process of ECM devices, but also to design the devices for high performance.
In this work, by using the first-principles simulation, we examine the atomic structures and Cu ions diffusion behaviors in a-Ta2O5 and a-SiO2 nanopores with and without the presence of H2O. Our work shows that the different adsorption behaviors of H2O in a-Ta2O5 and a-SiO2 nanopores can be ascribed to the different features of the sidewalls of their nanopores. That is, the existence of unsaturated and saturated cations on a-Ta2O5 and a-SiO2, respectively. On the other hand, the presence of H2O is found to enhance the diffusion of Cu ions along the nanopores in both cases of a-Ta2O5 and a-SiO2. This can be attributed to the drastic weakening of the interaction between Cu ions and a-Ta2O5/a-SiO2 nanopores after H2O adsorption.
2. Theoretical method
All calculations are performed using the Vienna ab initio simulation package (VASP) [Citation14,Citation15]. A plane wave basis set with a cutoff energy of 400 eV is used. A projector augmented-wave (PAW) [Citation16] method and a generalized gradient approximation (PW91) [Citation17] are adopted to describe the electron-ion and electron-electron interactions, respectively. To construct the surface structures of amorphous Ta2O5, molecular dynamics (MD) simulations are carried out at room temperature using the NVT ensemble (constant number, volume, and temperature). The time steps are set at 3 fs and 1 fs for the systems without and with H2O, respectively. The structure is sampled up to 9 ps to obtain a sufficiently equilibrated structure. Considering the large cell size, only the Г point is used during the MD simulation for the Brillouin-zone integration. The structure optimization is performed with 2 × 2 × 1 k-points. The convergence criteria adopted here assumes that the maximum force acting on each atom is smaller than 0.05 eV/Å.
To investigate the diffusion behaviors of Cu ion on a-Ta2O5 surface, again MD simulation can be employed as have done in a recent study of the moisture effect on the diffusion of ions in a novel CO2 sorbent [Citation18]. In the present study, however, the climbing-image nudged elastic band (CI-NEB) method [Citation19] is employed using 2 × 2 × 1 k-points because realistic atomic structures of nanopores are difficult to obtain as mentioned in the next section. To examine the interaction strength between Cu or H2O and a-Ta2O5/a-SiO2 surface, the adsorption energy is defined as following: Eads = EMOx-Cu/H2O – ECu/H2O – EMOx, where EMOx-Cu/H2O, ECu/H2O, and EMOx denote the energies of a-Ta2O5/a-SiO2 surface with the adsorbed Cu or H2O, the pure Cu or H2O, and the pure a-Ta2O5/a-SiO2 surface, respectively.
3. Results and discussion
3.1 Atomic structure of a-Ta2O5 nanopore without water
To examine the diffusion behaviors of Cu ions in a-Ta2O5-based ECM device, first a structure model of a-Ta2O5 nanopore is constructed. Experimental studies suggest that the diameters of the nanopore structures in a-Ta2O5 are about several nm [Citation6,Citation20,Citation21], which are too large to be treated using first-principles simulation directly. Accordingly, we need to construct an approximate a-Ta2O5 nanopore model that can reproduce the actual Cu ion diffusion behaviors within available computational resources. Since the interaction between the two sidewalls several nm apart from each other can be negligible, the diffusion of Cu ions along the sidewall of a-Ta2O5 nanopore could be viewed as the diffusion on the surface of a-Ta2O5 as schematically shown in ). Therefore, we adopt a-Ta2O5 surface model to investigate the diffusion behavior of Cu ions in Cu/a-Ta2O5/Pt, instead of the a-Ta2O5 nanopore structure.
Figure 1. (a) Schematic of the diffusion of Cu in Ta2O5 nanopore, and the atomic structures of amorphous (b) Ta2O5 and (c) SiO2 surfaces, respectively. The atoms and bonds on the Ta2O5 and SiO2 surfaces are displayed by using ball and stick model with relatively large sizes as compared with those in the other region. The yellow, purple, and cyan balls in (b) represent the Ta atoms bonding with 6, 5, and 4 O atoms, respectively. The green regions in (b) and (c) represent the void spaces on the surfaces.

In our previous work, we constructed the a-Ta2O5 bulk model by using molecular dynamics (MD) simulation [Citation22]. The structural features of as-generated a-Ta2O5 is consistent with the experimental data. To construct the a-Ta2O5 surface model, the a-Ta2O5 bulk model is cleaved with O-termination, which has been proven to be more stable than the Ta-terminated one [Citation23]. Subsequently, structure optimization and MD simulation are performed at the room temperature. As shown in ), the as-generated a-Ta2O5 surface model (with the chemical composition of Ta40O100) possesses a relatively large surface area (14.64 × 14.86 Å2) with a slab thickness about 11.20 Å in average.
As discussed in our previous study [Citation22], most of the Ta atoms in the bulk a-Ta2O5 are saturated by bonding with six O atoms. In the case of the surface region, on the other hand, most of Ta atoms are in the unsaturated forms, for example, Ta2O5 (see )). This point is discussed in more detail below.
In the a-Ta2O5 bulk structure, most of the O atoms bond with two Ta atoms [Citation22]. As a result, in the freshly cleaved a-Ta2O5 surface, most of the O atoms are coordinated with only one Ta atom. During the structure relaxation, we have observed the following trends of structural change on the a-Ta2O5 surface: (i) two adjacent O atoms with single coordination tends to bond together to form O2, and leave away from the system; (ii) TaO6 components tend to change into TaO5 by breaking one Ta-O bond in the subsurface region. As a result, TaO5 components become predominant on the a-Ta2O5 surface. In the case of a-SiO2, on the other hand, the surface structure has been successfully constructed in a previous theoretical study [Citation24] as shown in ), where all the O atoms on the a-SiO2 surface are coordinated with more than one Si atoms. The Si atoms on the surface and in the bulk region are all in their saturated form (SiO4). In the present study, we adopt this model in our calculations.
3.2 The atomic structure of a-Ta2O5 surface covered with water
The experimental work suggests that a small amount of H2O molecules tend to adhere to the sidewalls of a-Ta2O5 and a-SiO2 nanopores. The desorption of H2O takes place only at high temperature (~350°C) on a-Ta2O5 surface, but at room temperature on a-SiO2 surface [Citation12,Citation13]. To understand this point better, we have examined the adsorption of a single H2O molecule on the surface of a-Ta2O5 and a-SiO2. In doing so, all the possible adsorption sites have been considered because various chemical environments must appear on the surface of amorphous material (for details, see Figures S1 and S2 in Supporting Information). Our calculations show that a H2O molecule prefers to adsorb on an unsaturated Ta atom on the surface of a-Ta2O5, and it forms an O-Ta bond as seen in ) and Figure S1. The calculated averaged adsorption energy for such adsorption structures is about −0.94 eV, which means that the interaction between H2O and a-Ta2O5 surface is strong. Thus, the desorption process can only occur at high temperatures. On the other hand, in the case of a-SiO2, we have found that a H2O molecule weakly adsorbs on the a-SiO2 surface by forming hydrogen bonds (H∙∙∙O) as shown in ) and Figure S2. The calculated adsorption energy for such adsorption structures is about −0.35 eV in average. As a result, facile desorption of H2O from a-SiO2 surface is expected.
Figure 2. The adsorption structures of a single H2O molecule on amorphous (a) Ta2O5 and (b) SiO2 surfaces, respectively. (c) The structure of amorphous Ta2O5 surface covered by a thin layer of H2O.
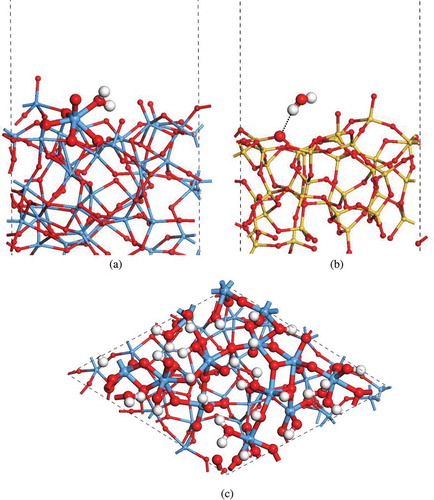
In the experiments, the sidewall of a-Ta2O5 nanopore is covered with a thin layer of H2O molecules [Citation6]. To model such a situation, 10 H2O molecules are initially placed on a surface of a-Ta2O5 so as to form O-Ta bonds. Then the system is relaxed (with the other surface fixed) by MD simulation for 6 ps at the room temperature, and structure optimization is done subsequently. It is found that the H2O molecules on a-Ta2O5 surface tend to split into OH groups and H atoms during the structure relaxation. The OH group tends to adsorb on the undercoordinated Ta atom, and the H atom tends to bond with the adjacent O atom by forming another OH group. This is consistent with the fact that a large amount of OH groups was detected in the previous experimental study [Citation6]. To achieve a full OH termination on a-Ta2O5 surface, another 8 H atoms are added to the isolated O atoms to form the OH groups. The structure relaxations (including MD simulation for 3 ps and optimization) are carried out subsequently. As shown in ), the as-generated a-Ta2O5-H2O surface model possesses a surface area of 13.89 × 14.26 Å2. The average slab thickness is 12.42 Å. It has a chemical composition of Ta40O110H28. Compared with the pure a-Ta2O5 surface, the O atoms on a-Ta2O5-H2O surface are stabilized by the formation of O-H×××O hydrogen bonds, and there are no O-O bonds. As a result, the Ta atoms on the a-Ta2O5-H2O surface are hydroxylated and possess one or two hydroxyl groups. The predominant chemical components of the Ta atoms on the surface region have changed from TaO5 (at pure a-Ta2O5 surface) into TaO6 (at a-Ta2O5-H2O surface). Accordingly, we can say that the adsorption of H2O molecules could further stabilize the surface structure of a-Ta2O5 by saturating the Ta and O atoms on the surface.
3.3 The diffusion of cu ions on a-Ta2O5 surface without and with water
Using the above a-Ta2O5 surface models, next we examine the diffusion behavior of Cu ion on the a-Ta2O5 surface. To do this, we first consider all the possible adsorption sites of a single Cu ion on the a-Ta2O5 surface without and with the presence of H2O. The possible diffusion pathways between the adjacent Cu adsorption sites are then calculated.
In the case of bare a-Ta2O5 surface, total 14 Cu adsorption sites have been identified as shown in ). We have found that a single Cu ion prefers to locate itself between the two O atoms in the void spaces of pure a-Ta2O5 surface (for details, see the supporting information (Figure S3)). The calculated average adsorption energy of Cu ion on the bare a-Ta2O5 surface is −1.88 eV, which indicates a strong interaction between the Cu and the surface. Then, we calculate the possible diffusion pathways between two adjacent Cu adsorption sites, and four paths across the whole a-Ta2O5 surface as identified in ). In each path, at least one of the diffusion steps between two adjacent Cu adsorption sites has the energy barrier higher than 1.40 eV, with the maximum value of about 2.20 eV. It is to be noted that one of the authors of this work and his collaborators recently examined the diffusion barriers of Cu ion in the bulk a-Ta2O5 (with the volume of 1447.46 Å3) using a neural network interatomic potential fitted to density functional theory calculation data. That work reported barrier values ranging from 0.68 eV to 1.79 eV [Citation25] for paths connecting a site in a supercell, and an equivalent one in the adjacent supercell. From calculations presented in [Citation25] and in this work, we conclude that the diffusion of Cu ions in pure a-Ta2O5 (both the bulk region and the nanopore of a-Ta2O5) is quite difficult. In fact, experimental study in [Citation26] has shown that the a-Ta2O5 could act as the barrier layer for the Cu ions diffusion within the temperature range of 450–600°C. It should be noted that this temperature range is higher than the desorption temperature of H2O from a-Ta2O5 (~350°C) [Citation6]. Thus, the barrier property in that experiment must be ascribed to that of the pure a-Ta2O5, which further confirms our findings. Another experimental study [Citation27] showed that the coordination number of a diffusing Cu with O atoms in a-Ta2O5 at 350°C is about 1.8 ~ 2.0. This is consistent with our theoretical result that the Cu ion prefers to bond with two O atoms on the bare surface of a-Ta2O5.
Figure 3. (a) The adsorption sites, the diffusion paths, and (b) the diffusion barriers of Cu on pure amorphous Ta2O5 surface.
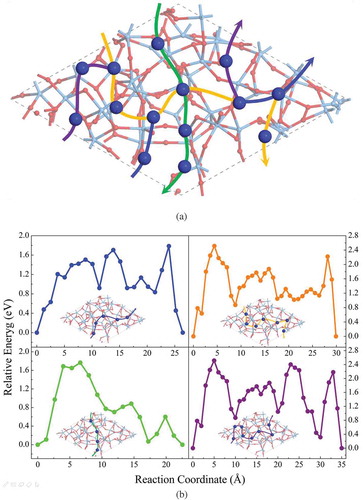
In the case of a-Ta2O5 surface covered with H2O molecules, 14 Cu adsorption sites have been identified as shown in ) and Figure S4. The calculated average adsorption energy is −0.69 eV, which is obviously lower than that of pure a-Ta2O5 surface (−1.88 eV). Four diffusion paths have been considered as shown in ). Here, the lowest diffusion barrier is estimated to be 0.43 eV, which agrees excellently with the value estimated in a recent experimental study (0.40 eV) [Citation27]. In addition, the estimated diffusion barrier (0.43 eV) of Cu ions on a-Ta2O5 surface covered with H2O is much lower than that in the case of bare a-Ta2O5 surface (>1.40 eV), which further confirms the experimental result that H2O molecules in a-Ta2O5 matrix could enhance Cu ion diffusion in Cu/a-Ta2O5/Pt device [Citation6]. Accordingly, the simulation results based on our constructed models can reproduce the experimental results well.
Figure 4. (a) The adsorption sites, the diffusion paths, and (b) the diffusion barriers of Cu on amorphous Ta2O5 surface covered by H2O. The green regions in (a) respect the Ta2O5 surface with low H2O coverage area.

Now, let us discuss the atomic details of the diffusion of Cu ion on a-Ta2O5 surface covered with H2O. After examining all the Cu adsorption structures, we have found that the adsorption energies and structures of Cu ion on a-Ta2O5 strongly depend on the H2O coverage on a-Ta2O5 surface. As shown in the supporting information (Figure S4), the Cu adsorption energies (−1.19 eV in average) on a-Ta2O5 surface with low H2O coverage area are lower than those on the surface with high H2O coverage (−0.43 eV in average). In the low H2O coverage area, Cu ions tend to be irreversibly trapped into the H2O layer, forming two Cu-O bonds. In this case, to escape from the trap, Cu ions need to overcome a high energy barrier (about 1.00 eV, see )). In the high H2O coverage area, on the other hand, Cu ions prefer to bond with only one O atom. Its location is on the top of H2O layer. As a result, the diffusion of Cu ion on the surface of H2O layer is much easier since only one Cu-O bond needs to be broken in each diffusion step.
It should be noted that, in the cases of high H2O contents, several more H2O layers will adsorb on the a-Ta2O5 surface, and the diffusion of Cu ions are still on the surface of topmost H2O layer. Therefore, it is expected that the diffusion behaviors of Cu ions on a-Ta2O5 surface with high H2O contents will be similar to the case with a single H2O layer. Actually, Tsuruoka and Valov et al.’s experimental studies [Citation6,Citation9] have shown that the operation voltages of the Cu/Ta2O5/Pt device, which are strongly related to the diffusion behaviors of Cu ions, are not changed by increasing the ambient H2O pressure. In addition, the diffusion of Cu and H and/or O atoms may coexists. This possibility would be worth considering. This would need significantly more computations, as there are variety of possible cases. This is left as a future task. It is also left as a future task to build a mathematical model to describe the vapor pressure dependence of diffusion behaviors, such as the one constructed for an anion exchange absorbent for CO2 capture [Citation28].
3.4 The diffusion of Cu ions on a-SiO2 surface without and with water
Finally, we would like to discuss the diffusion behaviors of Cu ions on a-SiO2 surface. As seen in ), there are several void spaces on the bare a-SiO2 surface due to the low atomic density of this material. Our results show that Cu ion prefers to locate itself in such a void space of a-SiO2 surface while forming two Cu-O bonds. The calculated adsorption energies for the cases of such spaces are lower than −3.16 eV. It is to be noted that, in spite of different characters of Ta and Si atoms on bare a-SiO2 and a-Ta2O5 surfaces, the chemical environments of O and the adsorbed Cu ion are similar on the two amorphous surfaces in the sense that the Cu has two Cu-O bonds. Thus, an irreversible trapping of Cu ion on the bare a-SiO2 surface is expected. With the presence of H2O, the void space of a-SiO2 surface will be filled with the H2O, and Cu ion will diffuse along such H2O layer. Similar to the case of a-Ta2O5, it is expected that the diffusion of Cu ion on a-SiO2 surface covered with H2O will be much easier than that of bare a-SiO2 surface.
4. Conclusions
In this work, the atomic structures and the diffusion behaviors of Cu ion on a-Ta2O5 and a-SiO2 nanopores with and without H2O are discussed by using the first-principles simulations. Our results reveal that the Ta and Si atoms on the sidewalls of a-Ta2O5 and a-SiO2 nanopores are in under- and full-coordination with O atoms, respectively. As a result, the H2O molecules tend to strongly adsorb in the a-Ta2O5 nanopore with forming O-Ta bonds, while the interaction is weak between H2O and a-SiO2 nanopore with the formation of H∙∙∙O hydrogen bonds. This result could well explain the experimental observation that the desorption of H2O from a-Ta2O5 could occur only at high temperatures, while such process could easily take place at lower temperatures in the case of a-SiO2. Without the presence of H2O, the Cu ions tend to get irreversibly trapped in the void spaces on the sidewalls of a-Ta2O5 and a-SiO2 nanopores by forming two Cu-O bonds. With the presence of H2O, the adsorption strength of Cu ions in a-Ta2O5 and a-SiO2 nanopores dramatically weaken, since the H2O fills in the void spaces in a-Ta2O5 and a-SiO2 nanopores. As a consequence, the diffusion of Cu ions could be enhanced by the presence of H2O in a-Ta2O5 and a-SiO2 nanopores. Our results would be helpful to understand the switching mechanisms of Cu/Ta2O5/Pt and Cu/SiO2/Pt resistance switches.
Supplemental Material
Download PDF (6.1 MB)Acknowledgments
S. W. thanks Prof. Shu Yamaguchi, Prof. Tsuyoshi Hasegawa, Dr. Tohru Tsuruoka, Dr. Toshi Sakamoto, and Dr. Naoki Banno for fruitful discussion. Part of the computations in this work was performed using the facilities of the Supercomputer Center, Institute for Solid State Physics, University of Tokyo.
Disclosure statement
No potential conflict of interest was reported by the authors.
Supplementary material
Supplemental data for this article can be accessed here.
Additional information
Funding
References
- Terabe K, Hasegawa T, Nakayama T, et al. Quantized conductance atomic switch. Nature. 2005;433:47–50.
- Wedig A, Luebben M, Cho DY, et al. Nanoscale Cation motion in TaOx, HfOx and TiOx memristive systems. Nat Nanotechnol. 2016;11:67–74.
- Yu SM, Chen HY, Gao B, et al. HfOx based vertical resistive switching random access memory suitable for bit-cost-effective three-dimensional cross-point architecture. ACS Nano. 2013;7:2320–2325.
- Mehonic A, Shluger AL, Gao D, et al. Silicon oxide (SiOx): a promising material for resistance switching? Adv Mater. 2018;30:1801187.
- Tsuruoka T, Valov L, Tappertzhofen S, et al. Redox reactions at Cu,Ag/Ta2O5 interfaces and the effects of Ta2O5 film density on the forming process in atomic switch structures. Adv Funct Mater. 2015;25:6374–6381.
- Tsuruoka K, Terabe K, Hasegawa T, et al. Effects of moisture on the switching characteristics of oxide-based, gapless-type atomic switches. Adv Funct Mater. 2012;22:70–77.
- Sakamoto T, Lister K, Banno N, et al. Electronic transport in Ta2O5 resistive switch. Appl Phys Lett. 2007;91:092110.
- Nandakumar SR, Minvielle M, Nagar S, et al. A 250 mV Cu/SiO2/W memristor with half-integer quantum conductance states. Nano Lett. 2016;16:1602–1608.
- Valov I, Tsuruoka T. Effects of moisture and redox reactions in VCM and ECM resistive switching memories. J Phys D: Appl Phys. 2018;51:413001.
- Tappertzhofen S, Waser R, Valov I. Impact of the counter-electrode material on redox processes in resistive switching memories. ChemElectroChem. 2014;1:1287.
- Lübben M, Menzel S, Park SG, et al. SET kinetics of electrochemical metallization cells: influence of counter-electrodes in SiO2/Ag based systems. Nanotechnology. 2017;28:135205.
- Oshio S, Yamamoto M, Kuwata J, et al. Interaction of reactively sputtered TaOx thin films with In-Sn-O thin films and properties of TaOx thin films. J Appl Phys. 1992;71:3471.
- Takeuchi M, Martra G, Coluccia S, et al. Evaluation of the adsorption states of H2O on oxide surfaces by vibrational absorption: near-and mid-infrared spectroscopy. J Near Infrared Spectrosc. 2009;17:373.
- Kresse G, Furthmüller J. Efficiency of ab-Initio total energy calculations for metals and semiconductors using a plane-wave basis set. Comput Mater Sci. 1996;6:15–50.
- Kresse G, Furthmüller J. Efficient iterative schemes for ab-Initio total-energy calculations using a plane-wave basis set. Phys Rev B. 1996;54:11169–11186.
- Kresse G, Joubert J. From ultrasoft pseudopotentials to the projector augmented-wave method. Phys Rev B. 1999;59:1758–1775.
- Wang Y, Perdew JP. Correlation hole of the spin-polarized electron gas, with exact small-wave-vector and high-density scaling. Phys Rev B. 1991;44:13298–13307.
- Shi XY, Xiao H, Liao XB, et al. Humidity Effect on Ion Behaviors of Moisture-Driven CO2 Sorbents. J Chem Phys. 2018;149:164708.
- Henkelman G, Jonsson H. Improved tangent estimate in the nudged elastic band method for finding minimum energy paths and saddle points. J Chem Phys. 2000;113:9978–9985.
- Ali R, Fan Y, King S, et al. Simulation of Cu Diffusion in Porous Low-k Dielectrics. ECS Trans. 2017;77:121–132.
- Sieber I, Hildebrand H, Friedrich A, et al. Initiation of tantalum oxide pores grown on tantalum by potentiodynamic anodic oxidation. J Electroceram. 2016;16:35–39.
- Xiao B, Gu TK, Tada T, et al. Conduction paths in Cu/amorphous-Ta2O5/Pt atomic switch: first-principles studies. J Appl Phys. 2014;115:034503. .
- Gu TK, Wang ZC, Tada T, et al. First-Principles simulations on bulk Ta2O5 and Cu/Ta2O5/Pt heterojunction: electronic structures and transport properties. J Appl Phys. 2009;106:103713.
- Comas-Vives A. Amorphous SiO2 Surface Models: energetics of the Dehydroxylation Process, Strain, Ab Initio Atomistic Thermodynamics and IR Spectroscopic Signatures. Phys Chem Chem Phys. 2016;18:7475–7482.
- Li WW, Ando Y, Watanabe S. Cu diffusion in amorphous Ta2O5 studied with a simplified neural network potential. J Phys Soc Jpn. 2017;86:104004.
- Alen P, Vehkamaki M, Ritala M, et al. Diffusion barrier properties of atomic layer deposited ultrathin Ta2O5 and TiO2 films. J Electrochem Soc. 2006;153:304–308.
- Shigeoka Y, Tsuruoka T, Hasegawa T. The rate limiting process and its activation energy in the formation process of a Cu/Ta2O5/Pt gapless-type atomic switch. Jpn J Appl Phys. 2018;57:035202.
- Shi XY, Li QB, Wang T, et al. Kinetic analysis of an anion exchange absorbent for CO2 capture from ambient air. PLoS ONE. 2017;12:e0179828.