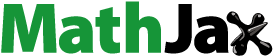
ABSTRACT
Structural color is a color derived from optical interaction between light and a microstructure and is often seen in nature. Natural melanin plays an important role in bright structural coloration. For example, the vivid colors of peacock feathers are due to structural colors. The periodic arrangement of melanin granules inside the feathers leads to light interference, and the black granules absorb scattered light well, resulting in bright structural color. In recent years, polydopamine (PDA) has attracted attention as a melanin mimetic material. This review article summarizes recent research on structural coloration using PDA-based artificial melanin materials. It also outlines possible applications using bright structural colors realized by artificial melanin materials and future perspectives.
Graphical abstract

1. Introduction
The structural color produced by the interaction of light and a microstructure does not fade as long as the microstructure is maintained, and light energy can be converted to color. Artificial fabrication of structural color materials is achieved by incorporating optical phenomena that generate structural colors, e.g. thin film interference, multilayer interference, diffraction, and scattering, into the material design to form a microstructure. The development of structural color materials that take advantage of the characteristics of each optical phenomenon is in progress [Citation1–5]. In particular, colloidal crystals generated by assembling colloidal particles have a wide range of designs, and many studies on these materials have been conducted [Citation6–10]. A structure in which monodisperse colloidal particles with a particle diameter of several hundred nm, close to the wavelength of light, are regularly arranged is called a colloidal crystal structure. The structural color produced by fcc (111) planes of the colloidal crystal structure is known to follow the Bragg-Snell EquationEquation (1)(1)
(1) shown below [Citation11].
where m is the diffraction order, λ is the wavelength of light, d is the interparticle distance, n is the effective refractive index of the colloidal crystal (particle and medium), and θ is the auxiliary angle of the incident light. Colloidal crystals produce highly reflective, angle-dependent structural colors due to the effects of Bragg diffraction (). In contrast, recent research has shown that when an amorphous structure with an intentionally disturbed arrangement structure is constructed, the scattering due to the particle size of the colloidal particles selectively enhanced, and angle-independent structural color can be obtained () [Citation12]. In the development of structural color materials, it is important to control the angle dependence of structural colors according to the application.
Figure 1. Two types of structure colors obtained by the assembly of colloidal particles: (a) colloidal crystals and (b) colloidal amorphous arrays
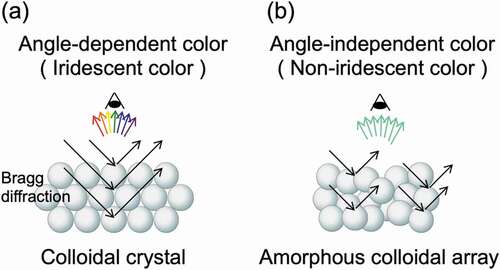
For structural colors produced by colloidal assemblies, the hue, saturation, and angle dependence of the color are controlled by parameters such as size, refractive index, blackness, and assembled structure of the particles used. Based on these parameters, the development of structural color materials is advancing from various perspectives [Citation13]. In designing colloidal assemblies, in addition to the method of creating the microstructures, the materials that make them up are important. Research on structural color materials from the viewpoint of biomimetics is being actively conducted [Citation14–17]. Another important challenge is the development of a method to generate highly visible structural colors from the assembly of colloidal particles. When light hits a submicron-sized microstructure that produces structural color, it appears white to the human eye due to multiple scattering of the light [Citation18]. One effective way to produce a bright structural color is to dope the assemblies of colloidal particles with black substances that absorb the extra scattered light. It has been reported that doping with black substances such as carbon black [Citation18–21], graphene [Citation22–24], iron oxide nanoparticles [Citation25,Citation26], and polypyrrole [Citation27] was effective in creating bright structural colors.
Structural colors are also found in the coloring of many organisms in nature, and melanin plays an important role in the generation of bright structural colors in these organisms. Over the past five years, research on structural color generation using polydopamine (PDA), which is a melanin-like substance inspired by structural coloration in nature, has rapidly progressed. This review article introduces the importance of melanin in the chromogenic mechanism of typical organisms with vivid structural colors and a recent topic on the development of structural colors with PDA-based artificial melanin materials.
2. The role of melanin in vivid structural colors in nature
Structural colors are often found in the vivid coloring of natural organisms such as insects and birds. The beautiful blue scales of the Morpho butterfly are attributed to structural color due to the hierarchical shelf structure [Citation28,Citation29]. Melanin is a dark brown to black substance that protects the skin from ultraviolet rays and is well known as a component of human hair [Citation30,Citation31]. In addition to the periodicity of the microstructure, the presence of melanin is essential for the structural color of organisms that are characterized by bright colors. In general, when light is applied to a submicron-sized microstructure, the light is scattered and appears white to the human eye. Since melanin can absorb a wide range of visible light, if part or all of a microstructure is made of melanin, scattered light will be absorbed, and the structural color will be conspicuous and appear bright to the human eye (). On the Morpho butterfly scale, the melanin layer is underneath the shelf structure, the scattered light is properly absorbed, and a bright blue structural color can be seen [Citation28,Citation29]. The shiny green color of the wings of jewel beetles, also known as tiger beetles, is also a typical structural color derived from multilayer interference (). The jewel beetle wing is composed of a multilayer film in which approximately 20 layers of melanin and cuticle are alternately stacked, and the structural color is clearly visible because the melanin layer in the microstructure properly absorbs scattered light [Citation32,Citation33]. The refractive index of melanin is also important in structural coloration. Because the melanin layer has a high refractive index, the reflectance enhanced at the interface between the melanin layer and the cuticle layer with a low refractive index [Citation34,Citation35]. Yabu and coworkers reported highly reflective structural color films, consisting of alternating multilayers of transparent poly(1,2-butadiene) (PB) and the black metal osmium (Os), which mimic the multilayered structure of jewel beetles [Citation36]. Since the refractive index of Os is much higher than that of PB, light was reflected at the interface between the two materials, causing strong reflection between multiple layers.
Figure 2. Photographs of (a) a Morpho butterfly, (b) a jewel beetle, (c) a peacock, and (d) an albino peacock. The stuffed peacocks shown in (c) and (d) are the property of the National Museum of Nature and Science
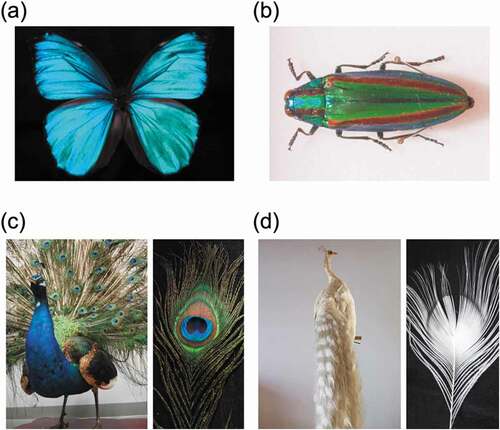
A famous example of structural coloration due to the microstructure formed by melanin itself is the color of peacock feathers (). Inside peacock feathers, rod-shaped melanin granules form a periodic microstructure that absorbs scattered light and creates a bright structural color [Citation37–39]. Organisms that cannot produce melanin in vivo are called albino. As shown in , albino peacock feathers, which lack the melanin microstructure, appear white [Citation40]. In natural vivid structural coloration, melanin plays an important role both in microstructure building and suppression of scattered light.
3. Characteristics of polydopamine as a melanin mimetic material
In vivo, melanin is produced from 3,4-dihydroxyphenylalanine (DOPA), an amino acid, and is classified into pheomelanin, eumelanin and neuromelanin depending on the composition () [Citation30,Citation31]. The complexity of the multistep enzymatic reaction makes it difficult to artificially synthesize microstructure-controlled melanin using the biosynthetic pathway. In 2007, Lee and coworkers reported that dopamine, a derivative of DOPA, was easily polymerized through self-oxidative polymerization to form PDA [Citation41]. Due to the excellent adhesion of PDA to the surfaces of various substrates, research into the use of PDA as a surface modifier has developed rapidly. In addition to surface coating methods that utilize the adhesiveness of PDA, advanced materials such as PDA-based gel materials, conductive materials, catalytic materials, self-healing materials, and biomaterials have been developed. Although this review does not include the history and applications of PDA, some recent review articles provide more details in these areas [Citation42–47].
Figure 3. Biosynthesis of natural melanin and artificial synthesis of melanin mimetic materials, i.e. PDA
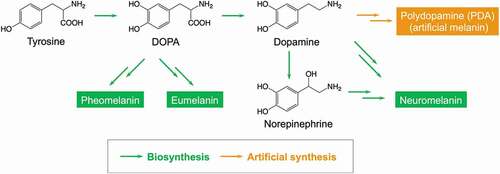
Since dopamine is a metabolite of DOPA in vivo, from a chemical structure perspective, PDA has almost the same composition as natural melanin and can be considered artificial melanin [Citation48]. From this viewpoint, research focusing on the structure-function relationship between natural melanin and PDA is progressing [Citation49]. Inspired by the functionality of natural melanin, PDA-based functional materials such as free radical scavengers [Citation50], UV absorbers [Citation51], metal ion probes [Citation52], catalyst [Citation53,Citation54], and antioxidants [Citation55] have been developed.
4. Polydopamine particle-based structural coloration
Our group has conducted research on colorless functionalized PDA thin films [Citation56–58] and morphology control of PDA thin films [Citation59]. In the course of these studies, it was found that by polymerizing dopamine in a water/methanol mixed solvent, monodisperse PDA particles can be obtained. In 2015, our group reported that a bright structural color was observed from the assembled structure obtained by concentrating an aqueous dispersion of monodisperse PDA particles that mimic melanin granules () [Citation60]. These colors were scattering-derived structural colors that result from the assembly of submicron-sized PDA particles, and the color tone was controlled by simply selecting the size of particles used. In addition, the color tone could be changed by attaching hairy polymer chains to the surface of PDA particles. When PDA particles grafted with a hydrophilic hair polymer were assembled, the tone of the structural color was controlled by the change in interparticle distance [Citation61]. Since PDA particles were well dispersed in water [Citation62], structural coloration by spray painting was also possible () [Citation60]. By coating PDA particles, which were well dispersed in an aqueous solvent, with a magnetic surfactant, the dispersibility in organic solvents was improved, and the color was changed by a magnetic field [Citation63]. The coloring mechanism in nature was reproduced, and bright structural coloration that suppressed light scattering was realized by constructing a microstructure with PDA particles of uniform size.
Figure 4. (a) SEM images of monodisperse PDA particles. (b) Scattering-derived structural colors from the assembly of submicron-sized PDA particles. (c) Structural colors achieved by spray coating of PDA particles. Reproduced with permission from [Citation60] (Copyright 2015, Royal Society of Chemistry). (d) Structural coloration based on thin-film interference using PDA particles. Reproduced with permission from [Citation64] (Copyright 2015, American Chemical Society). (e) Changes in structural color due to humidity. Reproduced with permission from [Citation65] (Copyright 2016, American Chemical Society). (f) Structural color achieved by placing a thin PDA film on top of a layer of PDA particles. Reproduced with permission from [Citation67] (Copyright 2015, American Chemical Society). (g) Structural coloration due to the hierarchical structure of the PDA thin film and PDA particles. Reproduced with permission from [Citation68] (Copyright 2017, Society of Polymer Science). (h) Non-iridescent structural color of a PDA coating on a silicon wafer. Reproduced with permission from [Citation71] (Copyright 2017, Royal Society of Chemistry)
![Figure 4. (a) SEM images of monodisperse PDA particles. (b) Scattering-derived structural colors from the assembly of submicron-sized PDA particles. (c) Structural colors achieved by spray coating of PDA particles. Reproduced with permission from [Citation60] (Copyright 2015, Royal Society of Chemistry). (d) Structural coloration based on thin-film interference using PDA particles. Reproduced with permission from [Citation64] (Copyright 2015, American Chemical Society). (e) Changes in structural color due to humidity. Reproduced with permission from [Citation65] (Copyright 2016, American Chemical Society). (f) Structural color achieved by placing a thin PDA film on top of a layer of PDA particles. Reproduced with permission from [Citation67] (Copyright 2015, American Chemical Society). (g) Structural coloration due to the hierarchical structure of the PDA thin film and PDA particles. Reproduced with permission from [Citation68] (Copyright 2017, Society of Polymer Science). (h) Non-iridescent structural color of a PDA coating on a silicon wafer. Reproduced with permission from [Citation71] (Copyright 2017, Royal Society of Chemistry)](/cms/asset/8528afa6-95f9-4f82-8a62-57e4da4caa06/tsta_a_1852057_f0004_oc.jpg)
Structural coloration based on thin film interference using PDA particles has also been reported. Xiao and coworkers reported that PDA particles with a size of approximately 184 nm were assembled to form a thin film, and the tone of the structural color changed depending on the film thickness () [Citation64]. In this case, it is possible to control the color tone by changing the film thickness by utilizing the hygroscopicity of PDA particles () [Citation65]. A high refractive index of 1.74 for PDA particles has been shown to be important for vivid structural coloration. Eom and coworkers reported that structural color was observed from a film in which natural melanin particles obtained from squid ink were deposited by the layer-by-layer (LBL) method [Citation66]. By controlling the film thickness with the LBL cycles, a wide range of iridescent colors were obtained. Structural coloration by thin film interference is possible, even if a PDA thin film prepared by directly polymerizing dopamine is used instead of a thin film in which PDA particles are assembled. Wu and coworkers obtained structural color by placing a thin PDA film on top of a layer of PDA particles that acted as a light absorbing layer () [Citation67]. Our group also demonstrated that when the polymerization of a dopamine-containing silane coupling agent was carried out under static conditions, structural color derived from the PDA thin film formed at the gas-liquid interface can be obtained () [Citation68]. At this time, the visibility of the structural color was enhanced by the PDA particles that were simultaneously generated on the liquid phase. Structural coloration due to the hierarchical structure of the PDA thin film and PDA particles can be regarded as a system that mimics the coloration of rock pigeon feathers [Citation69,Citation70]. Zang and coworkers reported that only coating a uniform PDA thin film on a silicon wafer yielded an angle-independent structural color () [Citation71]. They showed that large-scale and stimulus-responsive structural color films were synthesized by the co-deposition of PDA with poly(N-isopropylacrylamide). Vega and coworkers demonstrated structural color drawing by coating the surface of a silicon-silicon nitride wafer with a PDA film whose thickness varied depending on the patterned area [Citation72].
5. High-visibility structural coloration by core-shell-type artificial melanin particles
5.1. Particle design
Due to the extensive blackness of PDA particles, the structural color obtained by assembling PDA particles appears dark to the human eye. Thus, controlling the blackness of the particles is important for improving visibility. Our group reported high-visibility structural coloration by core-shell-type artificial melanin particles that can adjust the blackness, that is, the light absorption ability [Citation73]. Core-shell-type artificial melanin particles were designed using monodisperse polystyrene (PS) particles as the core material and PDA as the shell layer. The synthesized particles were named PS@PDA particles. By changing the feed concentration of the dopamine monomer, a PDA shell layer of any thickness can be provided, and the blackness of the particles can be easily controlled. The monodisperse core particles are important for building the microstructure, and the melanin-mimetic PDA shell layer is important for absorbing scattered light ().
Figure 5. (a) Design of core-shell type artificial melanin particles, i.e. PS@PDA particles. (b) Reflection spectra of pellet samples from PS particles (dotted line) and PS@PDA particles (solid line). The insets show photographs of the pellet samples. (c) Structural color pellets prepared from PS@PDA particles with different core diameters and PDA shell thicknesses. (d) Photographs and SEM images taken from different view angles of structural color pellets composed of PS@PDA particles. Reproduced with permission from [Citation73] (Copyright 2016, Nature Publishing Group)
![Figure 5. (a) Design of core-shell type artificial melanin particles, i.e. PS@PDA particles. (b) Reflection spectra of pellet samples from PS particles (dotted line) and PS@PDA particles (solid line). The insets show photographs of the pellet samples. (c) Structural color pellets prepared from PS@PDA particles with different core diameters and PDA shell thicknesses. (d) Photographs and SEM images taken from different view angles of structural color pellets composed of PS@PDA particles. Reproduced with permission from [Citation73] (Copyright 2016, Nature Publishing Group)](/cms/asset/3ae2cb5d-9f06-4b11-b731-6b00e16cc001/tsta_a_1852057_f0005_oc.jpg)
5.2. Highly-visibility structural color
Polymer particles such as PS particles are often used to form colloidal crystals. When the aqueous dispersion of PS particles was dropped on a silicone rubber plate, solid pellets were prepared by natural drying, and white pellets with an opal-like color were obtained. On the other hand, from the PS@PDA particles, highly visible structural color pellets were obtained. shows the results of the absolute reflectance measurement of the pellet surface, which was prepared from PS and PS@PDA particles. Although the reflectance of the peak at approximately 480 nm derived from blue structural color was higher for the pellets made of PS particles, the background due to light scattering was higher throughout the visible light range and appeared white to the human eye (dotted line in ). On the other hand, the background of pellets of PS@PDA particles was reduced overall (solid line in ). While the reflectance due to structural colors was also slightly reduced, the visibility to the human eye was dramatically improved, and highly visible structural coloration under normal ambient light was realized. This is because the light absorption layer composed of PDA appropriately absorbed the scattered light. By controlling the diameter of the core particles and the thickness of the PDA shell, it was possible to obtain nearly the full range of structural colors () [Citation73].
For practical use, the development of technologies for curing the colloidal crystal structure is important. Yi and coworkers reported the preparation of photonic crystals by co-assembly of PS@PDA particles and 3-aminopropyltriethoxysilane via a thermal-assisted self-assembly method [Citation74,Citation75]. Curing via a Schiff base reaction or Michael addition between the PDA shell layer and the silane coupling agent counteracts the tensile stress that is induced by latex shrinkage during drying, resulting in a crack-free photonic crystal that is resistant to wetting and impact. Chen and coworkers showed that co-assembly of polyurethane dispersions and PS@PDA particles resulted in structural color films with controlled angular dependence due to strong Brownian motion under heating conditions [Citation76]. Liu and coworkers reported that alkaline vapor treatment improved the adhesion and robustness of amorphous colloidal arrays prepared by spray coating PDA-coated silica (SiO2) particles, i.e. SiO2@PDA particles [Citation77]. This is because ammonia vapor provided a moist alkaline environment, which promoted further oxidative self-polymerization between PDA shells and cured samples. Shen and coworkers reported that the presence of chitosan during the natural drying of an aqueous dispersion of PS@PDA particles significantly suppressed the occurrence of cracks and improved the mechanical stability of the structural color coating [Citation78]. They showed that structural color coating was possible not only on rigid substrates but also on flexible cotton fabrics that offer robust mechanical stability.
5.3. Effect of assembled structure on color
There are two types of structural colors, i.e. iridescent and non-iridescent structural colors, depending on whether the color is angle dependent or not. The color of a peacock feather is iridescent structural color arising from the regularly assembled structure of rod-shaped melanin granules, and the hue of the structural color changes depending on the viewing angle (vide supra) [Citation37–39]. On the other hand, inside the feathers of Cotinga maynana, an amorphous structure arises from the keratin-based spongy matrix and air, and non-iridescent structural color is observed [Citation79]. This is because the amorphous structure does not have long-range order, maintains short-range order, and causes selective reflection according to the lattice size. Controlling the angle dependence is an important issue in the use of structural colors [Citation80,Citation81]. Interestingly, structural color pellets prepared by assembling PS@PDA particles can easily control the angular dependence of the structural color depending on the thickness of the PDA shell layer [Citation73]. Particles with a thin shell layer (< 5 nm) formed a close-packed colloidal crystal structure, producing an iridescent structural color ( left). On the other hand, particles with a thick shell layer (> 10 nm) showed a non-iridescent structural color due to the disordered amorphous structure ( right). When the PDA layer was thicker, the particle surface became rougher, which disturbed the particle arrangement and suppressed the formation of the close-packed structure. The great advantage of this method is that it is easy to control the assembled structure of the particles and the angular dependence of the structural color simply by controlling the PDA layer thickness.
It is also possible to control the angular dependence of the structural color by mixing particles [Citation82]. When PS@PDA particles with different particle diameters were mixed to form a pellet, the particle arrangement changed depending on the mixing ratio, and the presence or absence of angle dependence was controlled. In this case, the average particle size was adjusted stepwise by the mixing ratio of the particles. As shown in EquationEquation (1)(1)
(1) , the structural color depends on the particle size, so mixing the particles resulted in a neutral structural color (). The randomness of particle arrays is usually discussed in terms of Fourier transform images of scanning electron microscope (SEM) or transmission electron microscope (TEM) images [Citation80,Citation81]. show the SEM images of the pellet samples obtained by particle mixing and their Fourier transform images. On the surface of a pellet composed of one type of particle, the particles formed a close-packed structure, and a clear diffraction pattern was observed in the Fourier transform image. As the proportion of particle mixing increased, the particle arrangement became disordered, and a circular pattern was observed in the Fourier transform image. Although evaluation with a Fourier transform image is easy, it is difficult to visualize the degree of randomness of particle arrays. A Voronoi diagram is a diagram in which multiple points (seeds) at arbitrary positions in a metric space are divided into regions close to other points in the same metric space. Our group showed that the randomness of the array of particles can be visualized by a Voronoi diagram in SEM images using the center of the particle as a seed () [Citation82]. The randomness of a particle arrangement can be assessed by the proportion of hexagonal white polygons due to the close-packed structure (). The tone and angular dependence of the structural color can be adjusted simply by mixing particles, and it is expected to be applied to various coloring systems.
Figure 6. (a) Photographs and (b) SEM images of pellets obtained by mixing PS@PDA particles with different particle sizes. The scale bars are 1 μm. Large particles in the SEM images shown in (b) are marked in pink. (c) Fourier transform spectra and (d) determination of the particle centers of the SEM images shown in (b). (e) Voronoi diagram and (f) number of polygons. Red: 5-sided polygons, white: hexagonal polygons, blue: 7-sided polygons, green: others. Reproduced with permission from [Citation82] (Copyright 2017, American Chemical Society)
![Figure 6. (a) Photographs and (b) SEM images of pellets obtained by mixing PS@PDA particles with different particle sizes. The scale bars are 1 μm. Large particles in the SEM images shown in (b) are marked in pink. (c) Fourier transform spectra and (d) determination of the particle centers of the SEM images shown in (b). (e) Voronoi diagram and (f) number of polygons. Red: 5-sided polygons, white: hexagonal polygons, blue: 7-sided polygons, green: others. Reproduced with permission from [Citation82] (Copyright 2017, American Chemical Society)](/cms/asset/33a6ace3-3123-4080-b31f-573ac0cc39d6/tsta_a_1852057_f0006_oc.jpg)
5.4. Effect of melanin precursor on color
While melanin is the black component of human hair, each person has a different hair color. This is due to the difference in melanin content and melanin composition. In the abovementioned system, an example using dopamine as a starting material for producing artificial melanin was shown. Recent studies have shown that in addition to dopamine, DOPA and norepinephrine, which occur before and after the in vivo metabolic pathway of dopamine, were also oxidatively polymerized to form polyDOPA and polynorepinephrine (PNE) [Citation83–85]. From these findings, our group has shown that core-shell type artificial melanin particles were prepared by using DOPA and norepinephrine as starting materials [Citation86]. Although an oxidizing agent such as sodium periodate was required for the progress of polymerization, core-shell particles were prepared even when DOPA and norepinephrine were used as monomers, and structural coloration with high visibility was possible. In particular, when norepinephrine was used, the smoothness of the particle surface after generation was higher than that when dopamine or DOPA was used, and as a result, a structural color material with high angle dependence was easily obtained. The differences in the intermediates formed during the oxidative polymerization of dopamine and norepinephrine affected the roughness of the resulting PDA and PNE thin films [Citation87,Citation88]. It was found that the properties of the artificial melanin particles produced change depending on the type of melanin precursor monomer, and the structural color that develops is also affected.
5.5. Effect of particle shape on color
In the preparation of artificial structural color materials in which colloidal particles are assembled, spherical and solid particles are generally used as building blocks. Looking at nature, the shapes of melanin granules, which are the constituents of structural coloration, are diverse. As mentioned above, in the structural color of peacock feathers, rod-shaped melanin granules are used as a building block [Citation37–39]. Mimicking the shape of the building block, our group reported the structural coloration from ellipsoidal artificial melanin particles [Citation89]. Ellipsoidal artificial melanin particles were obtained by stretching a polyvinyl alcohol (PVA) film containing PS@PDA particles at a temperature above the glass transition temperature of the core PS particles, cooling the sample, and then melting the PVA. The aspect ratio of particles was controlled from 1 to 2.8 depending on the degree of film stretching. When the ellipsoidal artificial melanin particles were dried on the substrate, they tended to deposit sideways on the substrate. This trend was more clearly observed when ellipsoidal artificial melanin particles were deposited on the substrate by dip coating instead of natural drying (). As a result, the minor axis length of the ellipsoidal particles affected the structural coloration, and the structural color blueshifted as the aspect ratio increased ().
Figure 7. (a) SEM image of a sample in which ellipsoidal PS@PDA particles with an aspect ratio of 2.8 were assembled by a natural drying process and a dip coating method. The insets show photographs of the structural color samples. (b) TEM images of ellipsoidal PS@PDA particles with different aspect ratios and structural colors obtained by assembling those particles. Reproduced with permission from [Citation89] (Copyright 2019, American Chemical Society)
![Figure 7. (a) SEM image of a sample in which ellipsoidal PS@PDA particles with an aspect ratio of 2.8 were assembled by a natural drying process and a dip coating method. The insets show photographs of the structural color samples. (b) TEM images of ellipsoidal PS@PDA particles with different aspect ratios and structural colors obtained by assembling those particles. Reproduced with permission from [Citation89] (Copyright 2019, American Chemical Society)](/cms/asset/d6b6bb3a-65b1-4389-8d9f-28bf3deb2da5/tsta_a_1852057_f0007_oc.jpg)
Hollow-shaped melanin granules are also found as a material to build the microstructure to create the structural color of bird feathers [Citation90,Citation91]. Yi and coworkers reported that the hollow PDA amorphous colloidal structure exhibited an angle-independent structural color [Citation92]. They obtained hollow PDA materials by curing the PS@PDA particle assembled structure with 3-aminopropyltriethoxysilane and then removing the PS core particles by solvent treatment. Our group demonstrated the preparation of an inverse opal structure, which was prepared by depositing PS@PDA particles on the substrate by the dip coating method, curing the sample with a photocuring resin, and then removing the PS site by solvent treatment [Citation93]. In this case, a robust and bright structural color material with excellent angle dependency was obtained. A material with such a hollow PDA structure immobilized thereon is expected to have use as a sensor because the reflection wavelength changes depending on the refractive index of the solvent when immersed in the solvent. It is also possible to produce hollow melanin particles without curing the particle arrangement. Multiple coatings of the PDA shell layer resulted in artificial melanin particles with a thick and tough shell layer [Citation94]. Our group showed that hollow artificial melanin particles were obtained by dissolving the core material by solvent treatment of core-shell particles having thick PDA or PNE shell layers [Citation86]. As determined from EquationEquation (1)(1)
(1) , the wavelength of the structural color is highly dependent on the refractive index of the material. Since the voids inside the hollow artificial melanin particles contained air, the refractive index differed from that of solid particles, and the structural color tone of the pellet samples changed significantly.
5.6. Effect of the PDA composite method on color
As mentioned above, the assembled structure of PDA particles or core-shell particles with a PDA shell layer was useful for producing bright structural colors. On the other hand, according to previous research, the visibility of the structural color can be improved by forming colloidal assemblies by doping black substances. Research is also progressing from the perspective of doping melanin materials. Zhang and coworkers showed that bright structural colors were observed by doping non-spherical natural melanin particles with an average size of approximately 110 nm, obtained from cuttlefish ink, when making an assembled structure composed of PS particles [Citation95]. While there were limits to the size and uniformity of natural melanin particles, the visibility of the structural color was improved simply by doping the melanin material. Our group investigated the effect of the PDA composite method on structural color by comparing the binary co-assembly of cerium(IV) oxide (CeO2) particles and PDA particles of the same size with the unary assembly of CeO2@PDA core-shell particles [Citation96]. In the binary system, the distorted shape of CeO2 particles disturbed the particle arrangement, resulting in a non-iridescent structural color. While color unevenness may occur, bright structural colors with the same tone were obtained by simply mixing PDA particles. On the other hand, the PDA coating produced uniform core-shell particles and formed a colloidal crystal structure that gave an iridescent structural color with high visibility. By controlling the thickness of the PDA shell, it was possible to change the color tone according to the particle size.
6. Potential applications
6.1. Ink application
Background color when observing structural colors is important for the development of ink materials based on structural colors. When observing the structural color on a white background such as paper, the visibility of the structural color is reduced due to the scattering of light. Therefore, a black background is usually used to improve the visibility of structural colors. Our group reported that by using PS@PDA particles that can absorb light, it was possible to observe bright structural colors not only on a black background but also on a white background [Citation97]. The PS@PDA particles were well dispersed in an aqueous medium due to their high zeta potential (approximately −50 mV) [Citation73]. Taking advantage of this feature, our group succeeded in structural color printing by the inkjet method using an aqueous dispersion of PS@PDA particles () [Citation98]. The sample after inkjet printing on the overhead projector film had a dome-shaped structure consisting of particle assemblies. The inkjet method has achieved the structural color printing of each color according to the particle size. Bai and coworkers reported the usability of infiltration-assisted (IFAST) printing of PS@PDA particles on porous substrates for large-scale structural color printing in addition to inkjet printing [Citation99]. By IFAST printing in a nonequilibrium thermodynamic state with strong downward infiltration, the particles formed an amorphous colloidal array, providing an angle-independent structural color ().
Figure 8. (a) Structural color printing by inkjet using an aqueous dispersion of PS@PDA particles. Reproduced with permission from [Citation98] (Copyright 2019, Nature Publishing Group). (b) IFAST printing of PS@PDA particles. Reproduced with permission from [Citation99] (Copyright 2019, American Chemical Society). (c) Structural color film made on a flexible PET substrate by EPD of pre-PDA and SiO2 particles. Reproduced with permission from [Citation100] (Copyright 2019, American Chemical Society). (d) Structural colors obtained by assembling polymer particles on fabrics with and without PDA coating. Reproduced with permission from [Citation102] (Copyright 2019, Elsevier). (e) Structural colors obtained by building a colloidal crystal structure consisting of PS@PDA particles on both sides of the fabric. Reproduced with permission from [Citation103] (Copyright 2019, Elsevier)
![Figure 8. (a) Structural color printing by inkjet using an aqueous dispersion of PS@PDA particles. Reproduced with permission from [Citation98] (Copyright 2019, Nature Publishing Group). (b) IFAST printing of PS@PDA particles. Reproduced with permission from [Citation99] (Copyright 2019, American Chemical Society). (c) Structural color film made on a flexible PET substrate by EPD of pre-PDA and SiO2 particles. Reproduced with permission from [Citation100] (Copyright 2019, American Chemical Society). (d) Structural colors obtained by assembling polymer particles on fabrics with and without PDA coating. Reproduced with permission from [Citation102] (Copyright 2019, Elsevier). (e) Structural colors obtained by building a colloidal crystal structure consisting of PS@PDA particles on both sides of the fabric. Reproduced with permission from [Citation103] (Copyright 2019, Elsevier)](/cms/asset/f114a5e5-c9e1-4db6-a758-b1d41873f3cb/tsta_a_1852057_f0008_oc.jpg)
Echeverri and coworkers demonstrated a large area structural color coating technique using electrophoretic deposition (EPD) [Citation100]. By performing EPD of SiO2 particles in the presence of the precursor of PDA (pre-PDA), a structural color film was obtained on the flexible polyethylene terephthalate (PET) substrate. The pre-PDA enhanced the mechanical properties and enabled flexibility of the structural color coating with bending resistance, showing the possibility of application to flexible displays (). Dyeing using structural colors is also performed using the adhesive properties of PDA. Shi and coworkers reported the creation of a structurally colored fabric by coating cotton fabric with monodisperse polymer particles with carboxyl groups and pre-PDA [Citation101]. The resulting fabric retained its original structural color even after 10 washes or heating to 100°C. They also showed that by assembling polymer particles on a PDA-coated fabric, the black background of the PDA thin layer significantly enhanced the color saturation of the structural color () [Citation102]. Wang and coworkers demonstrated that by building a colloidal crystal structure consisting of PS@PDA particles on both sides of the fabric, bright structural colors were obtained from the entire surface of the fabric () [Citation103].
6.2. Pigment application
A spherical photonic material with a three-dimensional structure constructed from colloidal particles is expected to have use as a structurally colored pigment, and many attempts have been made to achieve this application [Citation104–108]. Our group demonstrated the fabrication of spherical photonic materials by the membrane emulsification method, utilizing the property that PS@PDA particles were well dispersed in water [Citation109]. After making a monodisperse water-in-oil (W/O) emulsion containing PS@PDA particles and evaporating the solvent, a spherical photonic material was obtained, which showed structural color even when dispersed in the solvent. In addition to spherical photonic materials, a combination of microfluidic emulsification and solvent diffusion has also successfully created structural color fibers, which are fibrous photonic materials. Liu and coworkers also reported the microfluidic-assisted fabrication of spherical photonic materials using SiO2@PDA particles [Citation110]. The resulting spherical photonic material exhibited structural color depending on the size of the SiO2@PDA particles. Additionally, biomolecules were immobilized on the surface of spherical photonic materials via the PDA layer, demonstrating their application in bioassays. Xiao and coworkers reported that spherical photonic materials were obtained by a one-pot reverse emulsion process using PDA@SiO2 core-shell particles, which have a melanin core with a high refractive index (~1.74) and a silica shell with a low refractive index (~1.45) [Citation111]. They designed PDA@SiO2 particles by finite-difference time-domain simulations and reported that the refractive index of the material is important for structural coloration. These three-dimensional photonic materials may be used in various applications by adding them to existing materials.
6.3. Anti-counterfeiting application
The structural color obtained by assembling the PDA particles appears dark to the human eye due to the high blackness of the PDA particles. Therefore, to obtain structural color, it is necessary to darken the environment and illuminate the sample with a strong light source. Cho and coworkers reported an application that positively exploited the property that the hue of the structural color changed before and after exposing the assembled PDA particle structure to light [Citation112]. As shown in , when a sample that appeared black due to the light absorption of PDA was irradiated with strong light, the structural color was selectively observed. The PDA particles in the solid matrix showed structural color only when illuminated with strong light, and the construction of arbitrary patterns showed their utility as anti-counterfeit applications. Liu and coworkers reported an anti-counterfeiting system inspired by Diphylleia grayi whose color tone changes before and after getting wet with rain [Citation113]. They showed that when the SiO2@PDA array was wetted with water, the original structural color disappeared, and the array appeared dark brown due to the absorption capacity of the PDA (, upper row). The brown hue could be controlled by the SiO2@PDA particle array thickness since the dark brown color was dependent on the array thickness. Paintings created by varying the size of the SiO2@PDA particles and the thickness of the array showed different patterns before and after wetting (, lower row). The background color is important for the visualization of structural colors, and research focusing on this phenomenon is progressing. For example, it has been reported that structural color was visualized upon changing from a white background to a black background using a polarizing plate [Citation114,Citation115]. Echeverri and coworkers demonstrated a system that visualizes structural color with temperature by creating a binary assembled structure of SiO2 and PDA particles on an epoxy resin film containing thermochromic microcapsules that change from white to black with temperature () [Citation116]. Additionally, it was shown that colloidal assemblies were efficiently constructed with a small amount of colloidal dispersion by using a substrate whose surface was perfluorinated by the cold plasma method. Our group also showed that the surface properties of the substrate were important in the formation of colloidal assemblies [Citation117]. The formation of colloidal crystal structures of PS@PDA particles on substrates was shown to be controlled by creating a surface whose wettability and adhesion were controlled by external stimuli.
Figure 9. (a) Structural color observed from PDA particles in a solid matrix when illuminated by strong light. Reproduced with permission from [Citation112] (Copyright 2017, Wiley-VCH). (b) Photographs of structurally colored arrays fabricated by SiO2@PDA particles exposed in air and water. Reproduced with permission from [Citation113] (Copyright 2019, American Chemical Society). (c) Structural color pattern visible by decreasing the temperature of a binary assembly structure of SiO2 and PDA particles printed on a thermochromic epoxy resin. Reproduced with permission from [Citation116] (Copyright 2020, American Chemical Society). (d) Reversible color changes due to stretching and relaxation of mechanochromic materials composed of elastomers containing binary colloidal arrays of SiO2 and PDA particles. Reproduced with permission from [Citation121] (Copyright 2019, American Chemical Society)
![Figure 9. (a) Structural color observed from PDA particles in a solid matrix when illuminated by strong light. Reproduced with permission from [Citation112] (Copyright 2017, Wiley-VCH). (b) Photographs of structurally colored arrays fabricated by SiO2@PDA particles exposed in air and water. Reproduced with permission from [Citation113] (Copyright 2019, American Chemical Society). (c) Structural color pattern visible by decreasing the temperature of a binary assembly structure of SiO2 and PDA particles printed on a thermochromic epoxy resin. Reproduced with permission from [Citation116] (Copyright 2020, American Chemical Society). (d) Reversible color changes due to stretching and relaxation of mechanochromic materials composed of elastomers containing binary colloidal arrays of SiO2 and PDA particles. Reproduced with permission from [Citation121] (Copyright 2019, American Chemical Society)](/cms/asset/dfa07be7-2ab1-477e-8267-9cfc5c09415f/tsta_a_1852057_f0009_oc.jpg)
6.4. Sensing application
Structural color-based sensing readouts are directly visible to the human eye without the need for instrumental measurements. One useful technique is the formation of colloidal crystal structures on elastomers such as rubber, which change the structural color upon stretching [Citation112–120]. Lee and coworkers reported the preparation of mechanochromic materials composed of elastomers containing binary colloidal arrays of SiO2 and PDA particles [Citation121]. Reversible color changes due to stretching and relaxation were demonstrated. In this case, as the elastomer was created by photocuring, various designs of structural color patterns were created by photolithography techniques (). Sensing with improved visibility of structural colors was achieved because the composite PDA particles reduced incoherent scattering. These materials are expected to have use as sensors that can detect local strain and stress with high sensitivity.
7. Summary and perspective
Artificial melanin materials based on PDA were created by mimicking natural melanin, which is important for vivid structural coloration in nature [Citation98,Citation122,Citation123]. By constructing a microstructure with black artificial melanin materials or incorporating them into the microstructure, the reflection and absorption of light were properly controlled, and highly visible structural coloration was realized. Since PDA is a highly biocompatible polymer that utilizes substances that are present in the living body, it may be useful to develop applications such as cosmetics that come into contact with the skin and safe and secure coloring agents, in addition to ink, anti-counterfeiting, and sensing materials. While the usefulness of artificial melanin-based structural color materials has been shown, the mechanism for constructing a periodic structure composed of natural melanin in vivo is still unknown. PDA is an easy-to-handle material that mimics natural melanin. In the future, it is expected that the knowledge introduced in this review article will contribute not only to practical research but also to the progress of basic research for understanding the development of structural colors in nature.
Acknowledgments
The author thanks the many collaborators listed in the references. The author is thankful for the experimental efforts and results achieved by many graduate students at Chiba University.
Disclosure statement
No potential conflict of interest was reported by the author.
Additional information
Funding
References
- Saito A. Material design and structural color inspired by biomimetic approach. Sci Technol Adv Mater. 2011;12:064709.
- Stefik M, Guldin S, Vignolini S, et al. Block copolymer self-assembly for nanophotonics. Chem Soc Rev. 2015;44:5076–5091.
- Sano K, Kim YS, Ishida Y, et al. Photonic water dynamically responsive to external stimuli. Nat Commun. 2016;7:12559.
- Mudachathi R, Tanaka T. Up scalable full colour plasmonic pixels with controllable hue, brightness and saturation. Sci Rep. 2017;7:1199.
- McConney ME, Rumi M, Godman NP, et al. Photoresponsive structural color in liquid crystalline materials. Adv Opt Mater. 2019;7:1900429.
- Yamanaka J, Murai M, Iwayama Y, et al. One-directional crystal growth in charged colloidal silica dispersions driven by diffusion of base. J Am Chem Soc. 2004;126:7156–7157.
- Yoshinaga K, Fujiwara K, Mouri E, et al. Stepwise controlled immobilization of colloidal crystals formed by polymer-grafted silica particles. Langmuir. 2005;21:4471–4477.
- Takeoka Y. Angle-independent colored materials based on the christiansen effect using phase-separated polymer membranes. Polym J. 2017;49:301–308.
- Minato H, Takizawa M, Hiroshige S, et al. Effect of charge groups immobilized in hydrogel microspheres during the evaporation of aqueous sessile droplets. Langmuir. 2019;35:10412–10423.
- Ohno K, Mizuta Y. Structural color materials using polymer-brush-decorated hybrid particles. ACS Appl Polym Mater. 2020;2:368–375.
- Ge J, Yin Y. Responsive photonic crystals. Angew Chem Int Ed. 2011;50:1492–1522.
- Yoshioka S, Takeoka Y. Production of colourful pigments using amorphous arrays of silica particles. ChemPhysChem. 2014;15:2209–2215.
- Goerlitzer ESA, Klupp Taylor RN, Vogel N. Bioinspired photonic pigments from colloidal self-assembly. Adv Mater. 2018;30:1706654.
- Zhao Y, Xie Z, Gu H, et al. Bio-inspired variable structural color materials. Chem Soc Rev. 2012;41:3297–3317.
- Dumanli AG, Savin T. Recent advances in the biomimicry of structural colours. Chem Soc Rev. 2016;45:6698–6724.
- Tadepalli S, Slocik JM, Gupta MK, et al. Bio-optics and bio-inspired optical materials. Chem Rev. 2017;117:12705–12763.
- Kolle M, Lee S. Progress and opportunities in soft photonics and biologically inspired optics. Adv Mater. 2018;30:1702669.
- Forster JD, Noh H, Liew SF, et al. Biomimetic isotropic nanostructures for structural coloration. Adv Mater. 2010;22:2939–2944.
- Takeoka Y, Yoshioka S, Takano A, et al. Production of colored pigments with amorphous arrays of black and white colloidal particles. Angew Chem Int Ed. 2013;52:7261–7265.
- Wang F, Zhang X, Lin Y, et al. Fabrication and characterization of structurally colored pigments based on carbon-modified ZnS nanospheres. J Mater Chem C. 2016;4:3321–3327.
- Takeoka Y. Environment and human friendly colored materials prepared using black and white components. Chem Commun. 2018;54:4905–4914.
- Zhang Y, Han P, Zhou H, et al. Highly brilliant noniridescent structural colors enabled by graphene nanosheets containing graphene quantum dots. Adv Funct Mater. 2018;28:1802585.
- Fu F, Chen Z, Wang H, et al. Graphene hybrid colloidal crystal arrays with photo-controllable structural colors. Nanoscale. 2019;11:10846–10851.
- Wang H, Liu Y, Chen Z, et al. Anisotropic structural color particles from colloidal phase separation. Sci Adv. 2020;6:eaay1438.
- He L, Wang M, Ge J, et al. Magnetic assembly route to colloidal responsive photonic nanostructures. Acc Chem Res. 2012;45:1431–1440.
- Teshima M, Seki T, Takeoka Y. Simple preparation of magnetic field-responsive structural colored janus particles. Chem Commun. 2018;54:2607–2610.
- Yang X, Ge D, Wu G, et al. Production of structural colors with high contrast and wide viewing angles from assemblies of polypyrrole black coated polystyrene nanoparticles. ACS Appl Mater Interfaces. 2016;8:16289–16295.
- Kinoshita S, Yoshioka S, Fujii Y, et al. Photophysics of structural color in the Morpho butterflies. Forma. 2002;17:103–121.
- Yoshioka S, Kinoshita S. Structural or pigmentary? Origin of the distinctive white stripe on the blue wing of a Morpho butterfly. Proc R Soc B. 2016;273:129–134.
- Riley PA. Melanin. Int J Biochem Cell Biol. 1997;29:1235–1239.
- Xiao M, Chen W, Li W, et al. Elucidation of the hierarchical structure of natural eumelanins. J R Soc Interface. 2018;15:20180045.
- Yoshioka S, Kinoshita S, Iida H, et al. Phase-adjusting layers in the multilayer reflector of a jewel beetle. J Phys Soc Jpn. 2012;81:054801.
- Schenk F, Wilts BD, Stavenga DG. The Japanese jewel beetle: a painter’s challenge. Bioinspired Biomimetic. 2013;8:045002.
- Schultz TD, Rankin MA. The ultrastructure of the epicuticular interference reflectors of tiger beetles (Cicindela). J Exp Biol. 1985;117:87–110.
- Seago AE, Brady P, Vigneron JP, et al. Gold bugs and beyond: a review of iridescence and structural colour mechanisms in beetles (Coleoptera). J R Soc Interface. 2009;6:S165–S184.
- Yabu H, Nakanishi T, Hirai Y, et al. Black thin layers generate strong structural colors: a biomimetic approach for creating one-dimensional (1D) photonic crystals. J Mater Chem. 2011;21:15154–15156.
- Yoshioka S, Kinoshita S. Effect of macroscopic structure in iridescent color of the peacock feathers. Forma. 2002;17:169–181.
- Zi J, Yu X, Li Y, et al. Coloration strategies in peacock feathers. Proc Natl Acad Sci USA. 2003;100:12576–12578.
- Li Y, Lu Z, Yin H, et al. Structural origin of the brown color of barbules in male peacock tail feathers. Phys Rev E. 2005;72:010902.
- Medina JM, Dias JA, Vukusic P. Classification of peacock feather reflectance using principal component analysis similarity factors from multispectral imaging data. Opt Express. 2015;23:10198–10212.
- Lee H, Dellatore SM, Miller WM, et al. Mussel-inspired surface chemistry for multifunctional coatings. Science. 2007;318(5849):426–430.
- Dreyer DR, Miller DJ, Freeman BD, et al. Perspectives on poly(dopamine). Chem Sci. 2013;4:3796–3802.
- Liu Y, Ai K, Lu L. Polydopamine and its derivative materials: synthesis and promising applications in energy, environmental, and biomedical fields. Chem Rev. 2014;114:5057–5115.
- Liu M, Zeng G, Wang K, et al. Recent developments in polydopamine: an emerging soft matter for surface modification and biomedical applications. Nanoscale. 2016;8:16819–16840.
- Ryu JH, Messersmith PB, Lee H. Polydopamine surface chemistry: a decade of discovery. ACS Appl Mater Interfaces. 2018;10:7523–7540.
- Lee HA, Park E, Lee H. Polydopamine and its derivative surface chemistry in material science: a focused review for studies at KAIST. Adv Mater. 2020;1907505. DOI:https://doi.org/10.1002/adma.201907505
- Yabu H. Molecular technology, volume 3: materials innovation: catechol-containing polymers: a biomimetic approach for creating novel adhesive and reducing polymers. Wiley-VCH; 2019. p. 53–70.
- d’Ischia M, Napolitano A, Ball V, et al. Polydopamine and eumelanin: from structure-property relationships to a unified tailoring strategy. Acc Chem Res. 2014;47:3541–3550.
- Xie W, Pakdel E, Liang Y, et al. Natural eumelanin and its derivatives as multifunctional materials for bioinspired applications: a review. Biomacromolecules. 2019;20:4312–4331.
- Ju KY, Lee Y, Lee S, et al. Bioinspired polymerization of dopamine to generate melanin-like nanoparticles having an excellent free-radical-scavenging property. Biomacromolecules. 2011;12:625–632.
- Huang Y, Li Y, Hu Z, et al. Mimicking melanosomes: polydopamine nanoparticles as artificial microparasols. ACS Cent Sci. 2017;3:564–569.
- Zhang S, Xiao M, Zhang Y, et al. Mimicking neuromelanin nanoparticles as a selective Pb2+ probe. Anal Chim Acta. 2020;1105:208–213.
- Zou Y, Wang Z, Chen Z, et al. Synthetic melanin hybrid patchy nanoparticle photocatalysts. J Phys Chem C. 2019;123:5345–5352.
- Kim HS, Kim M, Kang MS, et al. Bioinspired synthesis of melaninlike nanoparticles for highly N‑doped carbons utilized as enhanced CO2 adsorbents and efficient oxygen reduction catalysts. ACS Sustainable Chem Eng. 2018;6:2324–2333.
- Bao X, Zhao J, Sun J, et al. Polydopamine nanoparticles as efficient scavengers for reactive oxygen species in periodontal disease. ACS Nano. 2018;12:8882–8892.
- Kohri M, Kohma H, Shinoda Y, et al. A colorless functional polydopamine thin layer as a basis for polymer capsules. Polym Chem. 2013;4:2696–2702.
- Kohri M, Shinoda Y, Kohma H, et al. Facile synthesis of free-standing polymer brush films based on a colorless polydopamine thin layer. Macromol Rapid Commun. 2013;34:1220–1224.
- Kohma H, Uradokoro K, Kohri M, et al. Hierarchically structured coatings by colorless polydopamine thin layer and polymer brush layer. Trans Mat Res Soc Jpn. 2014;39:157–160.
- Kohri M, Nannichi Y, Kohma H, et al. Size control of polydopamine nodules formed on polystyrene particles during dopamine polymerization with carboxylic acid-containing compounds for the fabrication of raspberry-like particles. Colloids Surf, A. 2014;449:114–120.
- Kohri M, Nannichi Y, Taniguchi T, et al. Biomimetic non-iridescent structural color materials from polydopamine black particles that mimic melanin granules. J Mater Chem C. 2015;3:720–724.
- Kohri M, Uradokoro K, Nannichi Y, et al. Hairy polydopamine particles as platforms for photonic and magnetic materials. Photonics. 2018;5:36.
- Nishizawa N, Kawamura A, Kohri M, et al. Polydopamine particle as a particulate emulsifier. Polymers. 2016;8:62.
- Kawamura A, Kohri M, Taniguchi T, et al. Surface modification of polydopamine particles via magnetically-responsive surfactants. Trans Mat Res Soc Jpn. 2016;41:301–304.
- Xiao M, Li Y, Allen MC, et al. Bio-inspired structural colors produced via self-assembly of synthetic melanin nanoparticles. ACS Nano. 2015;9:5454–5460.
- Xiao M, Li Y, Zhao J, et al. Stimuli-responsive structurally colored films from bioinspired synthetic melanin nanoparticles. Chem Mater. 2016;28:5516–5521.
- Eom T, Woo K, Cho W, et al. Nanoarchitecturing of natural melanin nanospheres by layer-by-layer assembly: macroscale anti-inflammatory conductive coatings with optoelectronic tunability. Biomacromolecules. 2017;18:1908–1917.
- Wu TF, Hong JD. Dopamine-melanin nanofilms for biomimetic structural coloration. Biomacromolecules. 2015;16:660–666.
- Kawamura A, Kohri M, Oku H, et al. Structural color materials from polydopamine-inorganic hybrid thin films inspired by rock pigeon feathers. Kobunshi Ronbunshu. 2017;74:54–58. Japanese.
- Nakamura E, Yoshioka S, Kinoshita S. Structural color of rock dove’s neck feather. J Phys Soc Jpn. 2008;77:124801.
- Shawkey MD, D’Alba L, Wozny J, et al. Structural color change following hydration and dehydration of iridescent mourning dove (Zenaida macroura) feathers. Zoology. 2011;114:59–68.
- Zhang C, Wu BH, Du Y, et al. Mussel-inspired polydopamine coatings for large-scale and angle-independent structural colors. J Mater Chem C. 2017;5:3898–3902.
- Vega M, Martín Del Valle EM, Pérez M, et al. Color engineering of silicon nitride surfaces to characterize the polydopamine refractive index. ChemPhysChem. 2018;19:3418–3424.
- Kawamura A, Kohri M, Morimoto G, et al. Full-color biomimetic photonic materials with iridescent and non-iridescent structural colors. Sci Rep. 2016;6:33984.
- Yi B, Shen H. Facile fabrication of crack-free photonic crystals with enhanced color contrast and low angle dependence. J Mater Chem C. 2017;5:8194–8200.
- Yi B, Shen H. Structurally colored films with superhydrophobicity and wide viewing angles based on bumpy melanin-like particles. Appl Surf Sci. 2018;427:1129–1136.
- Chen G, Yi B, Huang Y, et al. Development of bright and low angle dependence structural colors from order-disorder hierarchical photonic structure. Dyes Pigm. 2019;161:464–469.
- Liu P, Chen J, Zhang Z, et al. Bio-inspired robust non-iridescent structural color with self-adhesive amorphous colloidal particle arrays. Nanoscale. 2018;10:3673–3679.
- Shen H, Liang Q, Song L, et al. Facile fabrication of mechanically stable non-iridescent structural color coatings. J Mater Sci. 2020;55:2353–2364.
- Prum RO, Torres R, Williamson S, et al. Coherent light scattering by blue feather barbs. Nature. 1998;396:28–29.
- Takeoka Y. Angle-independent structural coloured amorphous arrays. J Mater Chem. 2012;22:23299–23309.
- Katagiri K, Tanaka Y, Uemura K, et al. Structural color coating films composed of an amorphous array of colloidal particles via electrophoretic deposition. NPG Asia Mater. 2017;9:e355.
- Kawamura A, Kohri M, Yoshioka S, et al. Structural color tuning: mixing melanin-like particles with different diameters to create neutral colors. Langmuir. 2017;33:3824–3830.
- Hong S, Kim J, Na YS, et al. Poly(norepinephrine): ultrasmooth material-independent surface chemistry and nanodepot for nitric oxide. Angew Chem Int Ed. 2013;52:9187–9191.
- Liang RP, Xiang CY, Wang JW, et al. Preparation of polynorepinephrine adhesive coating via one-step self-polymerization for enantioselective cpillary electrochromatography coupled with electrogenerated chemiluminesense detection. J Chromatogr A. 2013;1284:194–201.
- Wei H, Ren J, Han B, et al. Stability of polydopamine and poly(DOPA) melanin-like films on the surface of polymer membranes under strongly acidic and alkaline conditions. Colloids Surf B. 2013;110:22–28.
- Iwasaki T, Tamai Y, Yamamoto M, et al. Melanin precursor influence on structural colors from artificial melanin particles: polyDOPA, polydopamine, and polynorepinephrine. Langmuir. 2018;34:11814–11821.
- Hong S, Na YS, Choi S, et al. Non-covalent self-assembly and covalent polymerization co-contribute to polydopamine formation. Adv Funct Mater. 2012;22:4711–4717.
- Hong S, Wang Y, Park SY, et al. Progressive fuzzy cation-π assembly of biological catecholamines. Sci Adv. 2018;4:eaat7457.
- Kohri M, Tamai Y, Kawamura A, et al. Ellipsoidal artificial melanin particles as building blocks for biomimetic structural coloration. Langmuir. 2019;35:5574–5580.
- Eliason CM, Bitton PP, Shawkey MD. How hollow melanosomes affect iridescent colour production in birds. Proc Biol Sci B. 2013;280:20131505.
- Shawkey MD, D’Alba L, Xiao M, et al. Ontogeny of an iridescent nanostructure composed of hollow melanosomes. J Morphol. 2015;276:378–384.
- Yi B, Shen H. Liquid-immune structural colors with angle-independence inspired from hollow melanosomes. Chem Commun. 2017;53:9234–9237.
- Kohri M, Kobayashi A, Okoshi T, et al. Bright solvent sensor using an inverse opal structure containing melanin-mimicking polydopamine. Chem Lett. 2020. DOI:https://doi.org/10.1246/cl.200626
- Yang L, Wang C, Ye Z, et al. Anisotropic polydopamine capsules with an ellipsoidal shape that can tolerate harsh conditions: efficient adsorbents for organic dyes and precursors for ellipsoidal hollow carbon particles. RSC Adv. 2017;7:21686–21696.
- Zhang Y, Dong B, Chen A, et al. Using cuttlefish ink as an additive to produce non-iridescent structural colors of high color visibility. Adv Mater. 2015;27:4719–4724.
- Iwasaki T, Harada S, Okoshi T, et al. Effect of the polydopamine composite method on structural coloration: comparison of binary and unary assembly of colloidal particles. Langmuir. 2020;36:11880–11887.
- Kohri M, Yamazaki S, Kawamura A, et al. Bright structural color films independent of background prepared by the dip-coating of biomimetic melanin-like particles having polydopamine shell layers. Colloids Surf A. 2017;532:564–569.
- Kohri M. Artificial melanin particles: new building blocks for biomimetic structural coloration. Polym J. 2019;51:1127–1135.
- Bai L, Lim Y, Zhou J, et al. Bioinspired production of noniridescent structural colors by adhesive melanin-like particles. Langmuir. 2019;35:9878–9884.
- Echeverri M, Patil A, Xiao M, et al. Developing noniridescent structural color on flexible substrates with high bending resistance. ACS Appl Mater Interfaces. 2019;11:21159–21165.
- Shi X, He J, Wu L, et al. Rapid fabrication of robust and bright colloidal amorphous arrays on textiles. J Coat Technol Res. 2020;17:1033–1042.
- Shi X, He J, Xie X, et al. Photonic crystals with vivid structure color and robust mechanical strength. Dyes Pigm. 2019;165:137–143.
- Wang X, Li Y, Zhou L, et al. Structural colouration of textiles with high colour contrast based on melanin-like nanospheres. Dyes Pigm. 2019;169:36–44.
- Moon JH, Yi GR, Yang SM, et al. Electrospray-assisted fabrication of uniform photonic balls. Adv Mater. 2004;16:605–609.
- Shen Z, Zhu Y, Wu L, et al. Fabrication of robust crystal balls from the electrospray of soft polymer spheres/silica dispersion. Langmuir. 2010;26:6604–6609.
- Teshima M, Seki T, Kawano R, et al. Preparation of structurally colored, monodisperse spherical assemblies composed of black and white colloidal particles using a micro flow-focusing device. J Mater Chem C. 2015;3:769–777.
- Teshima M, Suzuki M, Seki T, et al. Spontaneous preparation of monodispersed, structural colored, spherical particles by rotational stirring. ChemNanoMat. 2018;4:621–625.
- Ohnuki R, Sakai M, Takeoka Y, et al. Optical characterization of the photonic ball as a structurally colored pigment. Langmuir. 2020;36:5579–5587.
- Kohri M, Yanagimoto K, Kawamura A, et al. Polydopamine-based 3D colloidal photonic materials: structural color balls and fibers from melanin-like particles with polydopamine shell layers. ACS Appl Mater Interfaces. 2018;10:7640–7648.
- Liu P, Sheng T, Xie Z, et al. Robust, highly visible, and facile bioconjugation colloidal crystal beads for bioassay. ACS Appl Mater Interfaces. 2018;10:29378–29384.
- Xiao M, Hu Z, Wang Z, et al. Bioinspired bright noniridescent photonic melanin supraballs. Sci Adv. 2017;3:e1701151.
- Cho S, Shim TS, Kim JH, et al. Selective coloration of melanin nanospheres through resonant Mie scattering. Adv Mater. 2017;29:1700256.
- Liu P, Chang W, Ju L, et al. Bioinspired noniridescent structural color with hidden patterns for anticounterfeiting. ACS Appl Nano Mater. 2019;2:5752–5760.
- Iwata M, Teshima M, Seki T, et al. Bio-inspired bright structurally colored colloidal amorphous array enhanced by controlling thickness and black background. Adv Mater. 2017;29:1605050.
- Sakai M, Seki T, Takeoka Y. Bio-inspired colour materials combining structural, dye, and background colours. Small. 2018;14:1800817.
- Echeverri M, Patil A, Hu Z, et al. Printing a wide gamut of saturatedstructural colors using binary mixtures, with applications in anticounterfeiting. ACS Appl Mater Interfaces. 2020;12:19882–19889.
- Kohri M, Irie S, Yamazaki S, et al. Acid-induced control of surface properties using a catecholic silane coupling reagent. Chem Lett. 2019;48:551–554.
- Fudouzi H, Sawada T. Photonic rubber sheets with tunable color by elastic deformation. Langmuir. 2006;22:1365–1368.
- Ito T, Katsura C, Sugimoto H, et al. Strain-responsive structural colored elastomers by fixing colloidal crystal assembly. Langmuir. 2013;29:13951–13957.
- Lee GH, Choi TM, Kim B, et al. Chameleon-inspired mechanochromic photonic films composed of non-close-packed colloidal arrays. ACS Nano. 2017;11:11350–11357.
- Lee GH, Han SH, Kim JN, et al. Colloidal photonic inks for mechanochromic films and patterns with structural colors of high saturation. Chem Mater. 2019;31:8154–8162.
- Kohri M. Biomimetic structural color materials based on artificial melanin particles. J Photopolym Sci Technol. 2020;33:111–116.
- Xiao M, Shawkey MD, Dhinojwala A. Bioinspired melanin‐based optically active materials. Adv Opt Mater. 2020;87:2000932.