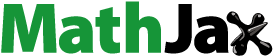
ABSTRACT
The interplay between charges and spins may influence the dynamics of the carriers and determine their thermoelectric properties. In that respect, magneto-thermoelectric power MTEP, i.e. the measurements of the Seebeck coefficient S under the application of an external magnetic field, is a powerful technique to reveal the role of magnetic moments on S. This is illustrated by different transition metal chalcogenides: CuCrTiS4 and CuMnTiS4 magnetic thiospinels, which are compared with magnetic oxides, Curie-Weiss (CW) paramagnetic misfit cobaltites, ruthenates, either ferromagnetic perovskite or Pauli paramagnet quadruple perovskites, and CuGa1-xMnxTe2 chalcopyrite telluride and Bi1.99Cr0.01Te3 in which diluted magnetism is induced by 3%-Mn and 1%-Cr substitution, respectively. In the case of a ferromagnet (below TC) and CW paramagnetic materials, the increase of magnetization at low T when a magnetic field is applied is accompanied by a decrease of the entropy of the carriers and hence decreases. This is consistent with the lack of MTEP in the Pauli paramagnetic quadruple perovskites. Also, no significant MTEP is observed in CuGa1-xMnxTe2 and Bi1.99Cr0.01Te3, for which Kondo-type interaction between magnetic moments and carriers prevails. In contrast, spin glass CuCrTiS4 exhibits negative MTEP like in ferromagnetic ruthenates and paramagnetic misfit cobaltites. This investigation of some chalcogenides and oxides provides key ingredients to select magnetic materials for which S benefits from spin entropy.
Graphical abstract
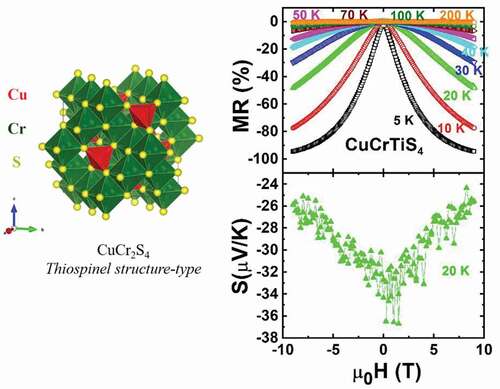
1. Introduction
Thermoelectric (TE) materials have the potential to be one of the green solutions towards todays’ global energy crisis [Citation1,Citation2]. Over the years, to make TE devices a commercial success, many efforts have been made first to optimize material parameters like Seebeck coefficient (S) and electrical resistivity (ρ) through band engineering approaches, including band distortion [Citation3], band convergence [Citation4,Citation5], and band nesting [Citation6], to find a candidate with a large power factor PF as PF enters the TE Figure of merit ZT (= S2T/(ρκ) = (PF)T/κ), where к and T are the thermal conductivity and absolute temperature, respectively. Many chalcogenides having transition metal cations with partially filled d orbitals in them exhibit fascinating TE properties. In most cases, their interesting transport properties cannot be described by standard models. Strong correlations between carriers and interplay between charges and spins can influence the dynamics of the carriers and determine their TE properties [Citation7–9]. Magnetic field-dependent thermoelectric power (MTEP) along with magnetoresistance (MR) studies provide a tool to unveil the possible mechanisms of unconventional transport properties of such magnetic systems and subsequently find new TE materials with suitable parameters. As the Seebeck coefficient depends on both transport coefficients and entropy [Citation10], the application of a magnetic field can affect both terms. A significant MTEP usually signifies large orbital or spin degrees of freedom of the carriers, which through increasing entropy can result in large S, which is required for TE application [Citation11]. At first, considering metal transition oxides, Wang et al., through MTEP measurement of NaxCoO2 samples with mixed valency of low spin Co3+ and Co4+, demonstrated that S is dominated by spin entropy in these layered oxides [Citation8]. As for the latter system, the misfit cobalt oxides, another class of compounds containing CoO2 layers of the CdI2-type (), exhibit a temperature dependence of S, which is not metal like. The low temperature slope of S in BiCaCoO misfit is strongly enhanced by the presence of paramagnetic spins as evidenced by its large negative MTEP below 20 K [Citation12]. However, in oxides, the MTEP is not limited to CdI2-type structure in which the transition metal forms hexagonal layers but is also found in square lattices of 3D perovskites (). A giant negative MTEP between −80% and −100% under 5 T in the 60–225 K temperature range was observed in the Nd0.75Na0.25MnO3 perovskite manganite and was accompanied by a large MR, which can be correlated with magnetic field-induced collapse of antiferromagnetism (AFM) [Citation13]. It is noteworthy to mention that large MTEP and MR can also be observed without the presence of any magnetic cation in some topological insulators and phases having Dirac states [Citation14–16]. Such effects arise from non-trivial band structure and are not in the scope of this study.
Figure 1. Schematic structural representation example of a) CuCr2S4 Thiospinel structure-type, b) Misfit Cobaltite structure, c) SrRuO3 Perovskite structure-type and d) CuGaTe2 Chalcopyrite structure-type
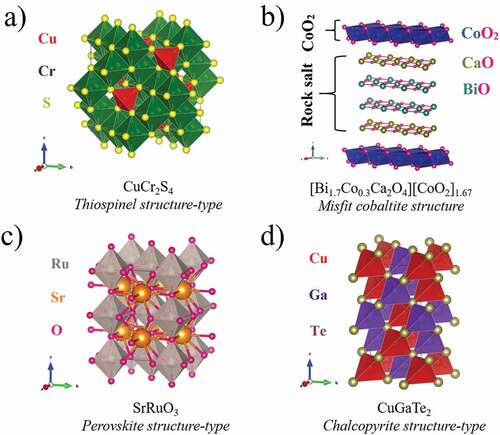
Apart from oxides, there exists other metal transition magnetic compounds containing chalcogen anions like S, Se and Te, which show excellent TE parameters with or without MTEP effect. For sulphides, considering the spinel structure first found in oxides, this is exemplified by the CuCrTiS4 thiospinel (), characterized by a large TEP absolute value (S300K = −180 µV/K), and for which MTEP was reported [Citation17]. This large S absolute value is remarkable if one considers the low S values reported for the metallic behaviours of both end members CuCr2S4 and CuTi2S4 [Citation17]. In some magnetic chalcopyrites also, where all metals are tetrahedrally coordinated to sulphur anions (), magnetism was invoked to explain the large TEP. Compounds derived from AFM CuFeS2 chalcopyrite may exhibit a large power factor, by doping at the Cu site, or by creating S vacancies [Citation18]. Indeed, a maximum power factor of 10−3 Wm−1K−2 was found for Cu0.97Zn0.03FeS2 where large TEP was explained through an enhancement of the effective mass m* due to the interaction between delocalized carriers and localized antiferromagnetic spins [Citation19,Citation20].
Here, we present an MTEP investigation of oxides and sulphides possessing different structures schematically described in . The variety of their crystallographic structures, 2D or 3D, with magnetic cations forming hexagonal or cubic arrays and with very different metal coordinations, octahedral in misfit cobaltites and perovskite ruthenates, tetrahedral in chalcopyrites or both octahedral and tetrahedral coordinations in thiospinels, is at the origin of different electronic and magnetic states. In the following, the relationship between magnetism and thermopower is demonstrated in this diversity of thermoelectric compounds. Though fundamental, our comparative study will help to understand and correlate the interplay between spin and thermal transport in systems having different magnetic properties, which might be important for their further development towards better TE materials.
2. Experimental details
According to our previous studies [Citation21–23], among magnetic chalcogenides, sulphides like CuCrTiS4 thiospinel and tellurides like CuGa1-xMnxTe2 chalcopyrite (0 ≤ × ≤ 0.03) and Bi1.99Cr0.01Te3 were chosen. The synthesis of these materials was carried out using solid state reaction technique by heating the constituent elements under vacuum. A CuMn0.5Ti1.5S4 ceramic sample was also prepared replacing Cr by Mn/Ti metal and using the same synthesis method as in Ref [Citation21]. As for oxides, three families were investigated with different magnetic properties: SrRuO3 perovskite, DCu3Ru4O12 (D= Na, Ca, Ca0.5La0.5, La) quadruple perovskites, and misfit cobaltites like [BiA2O4][CoO2]b1/b2 (A = Ca2+, Sr2+, Ba2+; b1/b2 = crystallographic misfit ratio) were taken for investigation. These oxides were prepared by standard ceramic method. The details of synthesis of the samples along with their in-depth structural characterization and experimental details about magnetic property measurements can be found elsewhere [Citation21–26].
Thermopower (TEP) measurements were performed using a homemade sample puck in a physical property measurement system (9 T-PPMS, Quantum Design, San Diego, USA) [Citation27]. Measurements are performed using a four-point steady-state technique with separate measuring and power contacts. Bars of typical dimension of 2×2×10 mm3 were mounted using GE varnish on the heat sink of the cryostat, and two chromel-constantan thermocouples were attached to monitor the temperature gradient. The thermoelectric voltage was measured through the chromel wires. For MTEP measurements (), magnetic field perpendicular to the temperature gradient was applied ()). Measurements were done either in isothermal conditions by varying the magnetic field or as a function of temperature in an applied magnetic field. The thermoelectric properties of CuGa1-xMnxTe2 and Bi1.99Cr0.01Te3 were measured using the TTO option from Quantum Design. For CuGa1-xMnxTe2, magnetic fields were applied both parallel and perpendicular to the temperature gradient. As shown below, the two different configurations gave essentially the same results. Thus, for Bi1.99Cr0.01Te3, the magnetic field was applied parallel to the direction of heat gradient ()).
Figure 2. Schematic diagram of the MTEP set up in (a) homemade sample puck where direction of magnetic field is perpendicular to the direction of heat flow, as represented by the black circles (b) TTO option from Quantum Design where direction of heat flow and magnetic field is parallel to each other
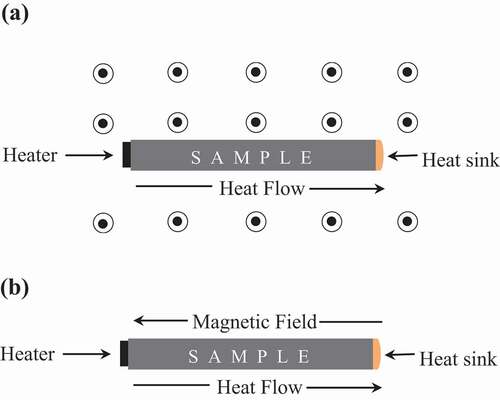
MR was measured in the same instrument by a conventional four probe method by applying magnetic field perpendicular to the current flow. For CuGa1-xMnxTe2 samples, MR measurements were performed by applying magnetic field both parallel and perpendicular to the current.
3. Results and discussions
3.1. Paramagnetic systems
For spinel chalcogenides, magnetic and magnetotransport properties are determined by distribution and localization of metal ions in their tetrahedral as well as octahedral cationic sites and their oxidation states [Citation28–30]. In this family, cubic thiospinel CuTi2S4 having Ti3+ and Ti4+ mixed valence exhibits a Pauli paramagnetic behaviour whereas a double exchange between high spin Cr3+ and Cr4+ is reported to be responsible for ferromagnetism observed in CuCr2S4 [Citation31,Citation32]. A solid solution exists between these two members, and the CuCrTiS4 thiospinel having half-filled t2g Cr3+ and empty t2g Ti4+ shows a paramagnetic behaviour down to low temperature (). A spin glass transition occurs at T = 8 K [Citation33]. Fitting susceptibility data with Curie-Weiss law shows a slight upward deviation of the experimental data below T = 75 K (inset of ). This points to small antiferromagnetic fluctuations occurring due to magnetic interaction among Cr3+ ions. While its magnetic properties are governed by Cr3+, Ti ions mainly contribute to carriers conduction. To describe transport properties for T < 75 K, a variable range hopping model (VRH) was employed for this system [Citation21]. Despite the metallicity and rather small S value of both end members, i.e. ferromagnet CuCr2S4 (S = +16 µV/K) and Pauli paramagnet CuTi2S4 (S = −12 µV/K) [Citation34], a thermopower as high as −140 µV/K at 300 K is observed in CuCrTiS4 (). Due to a small departure from the stoichiometry of the compound, a small fraction of Ti3+ ions having slightly filled t2g orbitals remains in the system, which leads to large negative S. A giant MR, reaching −95% at 5 K for 9 T is observed [Citation21] (top panel of ). This could be attributed to the gradual alignment of neighboring magnetic moments of Cr3+ ions with the application of external magnetic field. Such a large negative MR in the VRH regime is similar to the behaviour observed in colossal magneto-resistive (CMR) manganites [Citation35]. So MR in the whole T range was scaled as a function of , where BJ is the Brillouin function (in [Citation21]), with S = J = 3/2. This confirms that paramagnetic Cr3+ ions progressively align themselves as the temperature is lowered and give rise to giant negative MR. The effect of external magnetic field on S can be observed below 45 K as shown in . A large MTEP with absolute S decreasing from 34 µV/K at 0 T to 25 µV/K at 9 T for a typical temperature T = 20 K is obtained (inset of ). This S lowering can be attributed to the gradual alignment of Cr3+ spins, which decreases the magnetic entropy. For comparison, the properties of the metallic thiospinel CuMn0.5Ti1.5S4 are reported. This compound was found to be isostructural to the cubic CuCrTiS4 thiospinel. As shown in , the susceptibility is smaller in the whole T range, with a rapid increase at low T. A Curie-Weiss fitting to this T-dependent susceptibility in between 100 K and 300 K yields µeff = 5.7 µB/f.u. Such a value is consistent with S = 0 for Ti4+ and S = 5/2 for high spin Mn2+ as a theoretical value µeff = 5.9 µB is calculated for a high spin d4 cation. Thus, in both CuCrTiS4 and CuMn0.5Ti1.5S4, the Ti cations are tetravalent, whereas Cr is trivalent for the former and Mn divalent for the latter. CuMn0.5Ti1.5S4 is metallic with a small upturn of electrical resistivity below 50 K (inset of ), the temperature below which the χ values strongly increase. In this T range, below 50 K, a small negative MR appears, reaching ~ −2% in 9 T at 5 K (bottom panel of ). For this metallic thiospinel, the thermopower, with values in between those of CuCrTiS4 and CuTi2S4, exhibits negative values, varying almost linearly with temperature as expected for a metal (). In clear contrast with CuCrTiS4 which exhibits VRH transport, only a very small MTEP is detected (not shown), showing the major role of localized paramagnetic spins on MTEP.
Figure 3. Temperature dependence of magnetic susceptibility (χ) of CuCrTiS4 and CuMn0.5Ti1.5S4 in a field of 10−2 T. Inset: χ−1 (T) of CuCrTiS4 where the dashed line corresponds to the Curie-Weiss fitting
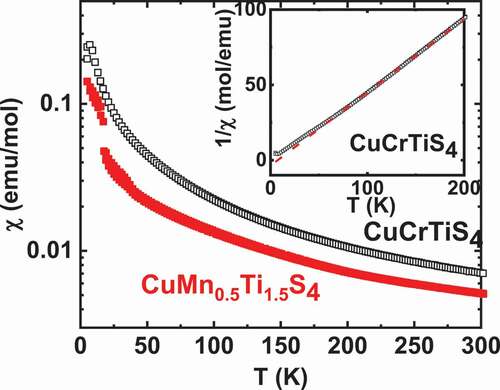
Figure 4. S(T) from 5 K to 325 K for CuCrTiS4, CuMn0.5Ti1.5S4 and CuTi2S4 thiospinels. Inset: Temperature dependence of electrical resistivity ρ for all the three samples
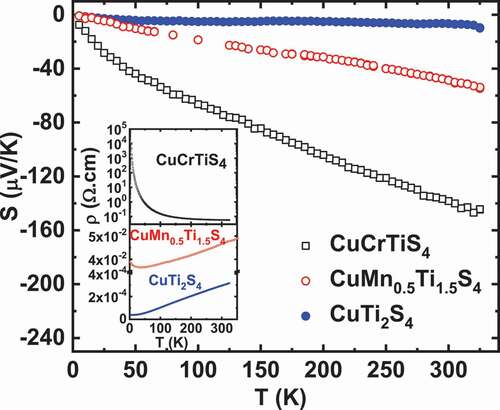
Figure 5. Isothermal Magnetoresistance curves of CuCrTiS4 (top) and CuMn0.5Ti1.5S4 (bottom) samples. Corresponding T values are shown in the graph
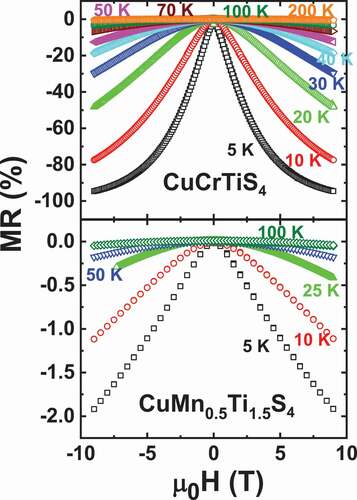
Figure 6. S(T) of CuCrTiS4 measured at 0 T and 9 T. Inset: Isothermal S(H) of CuCrTiS4 and BiCaCoO misfit oxide with the corresponding temperatures labeled in the graph
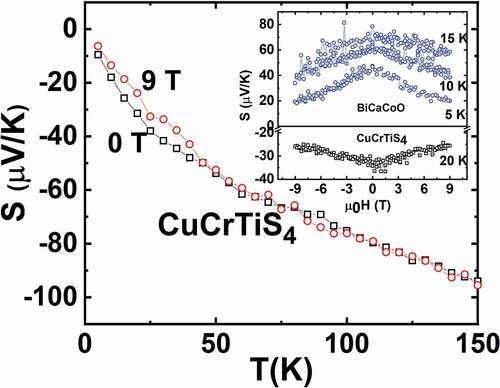
The positive impact of localized spins on thermopower had already been evidenced in Bi-based misfit cobaltites like [BiA2O4][CoO2]b1/b2 (A = Ca2+, Sr2+, Ba2+; b1/b2 = crystallographic misfit ratio). They consist of a single layer of CdI2 type [CoO2], which is stacked with four layers of rocksalt (RS) type structure [Citation24]. Cationic substitutions in the RS type layers are responsible for a doping of the [CoO2] layer by a mechanism of charge transfer and a modification of the positive charge in RS type layer, which is manifested through a change in the misfit ratio (b1/b2). These materials do not show any magnetic ordering and remain paramagnetic down to 2 K [Citation36]. depicts S(T) data for [Bi1.7Co0.3Ca2O4][CoO2]1.67 abbreviated as BiCaCoO. Within the temperature range 20–125 K, a linear variation of S is observed. The large value of the slope in the linear region below 20 K reflects strong electronic correlations in accordance with the high value of the Sommerfeld coefficient γ and the universality of the ratio S/(Tγ) [Citation7,Citation12]. As far as MTEP is concerned, a strong magnetic field dependence of S is observed for BiCaCoO [Citation24] below ~ 125 K: as shown in the inset of , S is dramatically reduced as the magnetic field is applied. This decrease in S can be attributed to the fact that as magnetic field increases, paramagnetic spins gradually align and magnetization (M) is increased leading to the loss of entropy. A maximum decrease of 60% in S is observed for 9 T at 5 K. A large negative MR is also observed reaching −87% for 7 T at 2.5 K for BiCaCoO. All the S(H) curves below 20 K can be scaled down as a function of H/T and fitted by a Brillouin function [Citation12]. Such a scaling behaviour confirms the freezing of spin fluctuations and hence the reduction of entropy in the system, which results in the reduction of S. It must be mentioned for comparison that in the metallic and Pauli paramagnet BiBaCoO misfit, no MTEP is observed [Citation24,Citation36], as discussed for thiospinels. MR and MTEP are observed only for localized carriers, with a large enough susceptibility associated with a Curie-Weiss behaviour.
At room temperature, no MTEP is observed in any of these misfit materials. However, the presence of magnetic cations still plays a role, as S at room temperature is mainly dependent on the formal valency of Co in [CoO2] layers, and on the fact that these Co cations carry a spin as shown by the generalized Heikes formula [Citation37,Citation38]. The S data around 300 K can be described by:
where Sn and Sn+1 are the spins of the transition metal Mn+ and M(n+1)+, respectively, x is the carrier concentration and гorb the orbital degeneracy. In the case of Bi-based misfits, both spin and orbital degeneracy terms related to low spin Co3+ (S = 0) and low spin Co4+ (S = ½) have to be taken into account to describe its room temperature S [Citation39,Citation40]. Taking into account the lifting of the t2g degeneracy [Citation40,Citation41] the spin and orbital terms correspond to ln (2) = ~ 60 µV/K [Citation8,Citation40], a sizable fraction of the thermopower, ~ 140 µV/K at 300 K for x = 0.33 ().
3.2. Ferromagnetic ruthenates
In the case of ruthenium oxides, the role of the spin entropy and magnetism can be investigated for different magnetic states due to the diversity of magnetic behaviour in these most often metallic oxides. The Seebeck coefficients of different ruthenium oxides exhibiting ferromagnetism (SrRuO3), paramagnetism (CaRuO3) and Pauli paramagnetism (quadruple perovskites) have been measured. SrRuO3 is a ferromagnetic metal (TC ~ 160 K) where Ru takes a d4 electronic configuration [Citation42]. In this perovskite structure, a low-spin (S = 1) state of Ru4+ is favoured due to the large crystal field splitting between eg and t2g orbitals in the presence of octahedrally connected oxygens [Citation43]. A close look at reveals that S(T) for SrRuO3 does not exhibit the expected linear dependence for a typical Drude metal, and that magnetism plays a role with an accident observed near TC ~ 160 K. In the case of the paramagnetic CaRuO3 [Citation44], the evolution is smoother up to high temperature. Calculation of the spin only term in Heikes formula using spins of Ru5+ (S = 3/2) in matrix of Ru4+ (S = 1) and Ru3+ (S = 1/2) in the matrix of Ru4+ gives S value of 25 µV/K and 35 µV/K, respectively, which is very close to the measured S for SrRuO3 and related ruthenates around 300 K [Citation25]. Above TC, S is driven by a constant spin entropy. This model is much too simple, and a more rigorous calculation has shown that in the case of Sr2RuO4, the orbital entropy is quenched up to 1200 K, leading to a spin entropy-dominated Seebeck coefficient below 1200 K [Citation45]. In addition, a negative MTEP is also observed below TC for SrRuO3 (inset of for T = 20 K). This field dependence of S follows the magnetization curve M(H) (): a gradual increase of external magnetic field makes the spins more aligned up to its saturation magnetization (MS) (to be compared to M(H), inset of ), leading to a decrease of S. From the inset of , when the saturation magnetization MS is reached, the MTEP becomes constant. The magnitude of the SrRuO3 MTEP is similar to the one measured in the ferromagnetic CaRu0.8Sc0.2O3 (−15% at 30 K) [Citation46].
3.3. Pauli paramagnetic ruthenates
To compare with these perovskites, S and ρ measurements of a group of quadruple perovskite (QP) samples DCu3Ru4O12 (D= Na, Ca, Ca0.5La0.5, La) having Ru oxidation state ranging from 3.75 (D = La3+) to 4.25 (D = Na+) and presenting Pauli like behaviours () were performed. Density of states calculations in these materials shows that the influence of Cu states can only be observed far below EF, and hence have no effect on the transport properties [Citation47]. ρ(T) measurements up to 900 K for LaCu3Ru4O12 exhibit a monotonic increase of ρ, almost linearly with T without any saturation ( in [Citation26]), consistently with the bad metal behaviour observed in many ruthenates such as SrRuO3 or Sr2RuO4 [Citation48–50]. Even though S values of all these QP and single perovskite SrRuO3 are similar (~32 µV/K) at the highest temperature of 900 K, the evolution of S(T) differs with a gradual increase in the case of QP, with a much smaller slope than for SrRuO3 ( and ). In fact, the saturation S value of 32 µV/K for SrRuO3 is reached at T ~ 200 K whereas around this temperature all the S curves of the QP group almost merge to 12 − 15 μV/K. At lower temperatures, there is a substantial change in the value of S of DCu3Ru4O12 and the values of S depend on the M value of the samples, with the smallest Seebeck coefficient measured for NaCu3Ru4O12 () which possesses the smallest Pauli susceptibility. For T > 200 K, all S curves converge and almost a similar S value of all the samples is observed in the temperature range 200–900 K. So formal Ru oxidation state has no effect on S in these samples. A monotonic increase in S up to high temperature can be rather related to classical metal-like picture following Mott’s formula, where band structure plays a significant role. MTEP measurements show no significant effect of external magnetic field on S in these Pauli paramagnets as exemplified for CaCu3Ru4O12 in the inset of .
3.4. Diluted paramagnetism in a telluride, a Kondo effect?
The last example is CuGa1-xMnxTe2 which is a paramagnetic system with no evidence of magnetic order observed at least down to 5 K (). Mn2+ doping in the pristine system increases hole concentration. As a result, smaller electrical resistivity ρ is observed in x = 0.03 as compared to x = 0. But in spite of increasing in carrier concentration, relatively high TEP is observed in Mn-doped sample. shows the effective mass enhancement m*/m0 of CuGa1-xMnxTe2, which has been derived from the Seebeck coefficients and the carrier concentrations at T = 325 K based on a parabolic band model. The effective mass enhances from 0.6m0 for x = 0 to 1.5m0 for x = 0.03 [Citation51]. It has been demonstrated that the interactions between holes and the magnetic ions play a pivotal role in enhancing m*. Strong correlation between magnetic ions and holes is inferred from the magnetoresistance (MR) shown in . At T = 10 K, transverse magnetoresistances MRT shown in decrease significantly, reaching almost −40% for x = 0.01 and −20% for x = 0.02 and 0.03. Shown in is the longitudinal magneto resistance MRL of x = 0.03 with field parallel to current, measured at various temperatures. MRL at T = 10 K almost agrees with MRT of x = 0.03 in , which means that the large MR is intrinsic to the coupling of carriers and magnetic moments. The strong coupling has also manifested itself as the unusually large anomalous Hall effect (AHE), observed in x = 0.03 for T ≤ 20 K [Citation22]. Calculation revealed a negative value of AHE constant RS which also indicates antiferromagnetic coupling between Mn2+ and carriers. It is notable that the magnetoresistance in is most significant in the diluted limit (x = 0.01), suggesting that the on-site interaction between hole and Mn2+ moment is responsible, rather than the Mn-Mn inter-site couplings.
Figure 13. Effective mass m* with respect to the free electron mass m0 of CuGa1-xMnxTe2 derived from a parabolic band model. Broken line is guide for eye
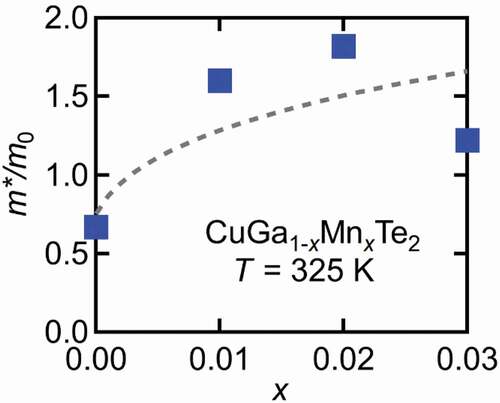
Figure 14. (a) Transverse magnetoresistance (MRT) of CuGa1-xMnxTe2 measured at T = 10 K, where magnetic field is perpendicularly applied to current. (b) Longitudinal magnetoresistance (MRL) of CuGa0.97Mn0.03Te2 where magnetic field is parallel to current
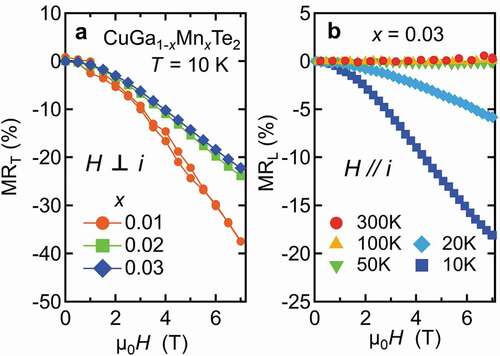
This interpretation is in accordance with the results of magnetic susceptibility. Magnetic susceptibility was fitted with Curie-Weiss function, where C, θ and χ0 is Curie constant, Weiss temperature and the temperature-independent term, respectively. The effective magnetic moment of 5.35μB obtained for x = 0.03 sample is close to the Mn2+ moment (5.92μB) with S = 5/2 and g = 2. In addition, the Weiss temperature comes out to be negative (−108.5 K), which indicates AFM interaction on Mn spins. One of the reasons of getting large negative θ can be AFM exchange between Mn2+ ions. But at such low Mn concentration, Mn-Mn distance is much longer, and it is difficult to imagine that Mn–Mn interaction would give rise to this large negative θ. In addition, the possibility of a direct overlap of 3d states of Mn is quite low in this case which rules out the contribution of Mn-3d impurity band to the large S (). This large negative θ and large S can arise due to Kondo-type interaction between magnetic impurities and carriers [Citation52]. The Kondo model was applied by Osinniy et al. to explain large TEP of ferromagnetic Ga1-xMnxAs [Citation53]. Kondo interaction is a process of compensating the magnetic moments of impurities by forming spin-singlet states with carrier electrons. In the low temperature limit, this effect eliminates the magnetic entropies of impurities, while the entropies are transferred to carrier electrons with enhanced effective mass. As a result, the Seebeck coefficient increases with the Kondo-type interaction. Indeed, enhanced effective carrier mass m* has been observed in CuGa1-xMnxTe2, as shown in .
Figure 15. Seebeck coefficient as a function of temperature of CuGa0.97Mn0.03Te2 measured under µ0H = 0 and 7 T. The field is perpendicular to heat flow in (a), while parallel in (b) . Inset shows electrical resistivity under µ0H = 0 T and 7 T with current perpendicular to field in (a), and parallel to field in (b). Broken line in (a) shows a fit with the Kondo-model
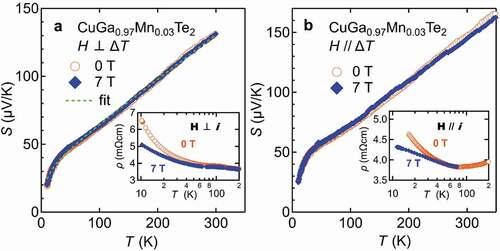
depicts the temperature dependence of S and electrical resistivity measured under zero and applied field of 7 T. Electrical resistivity ρ increases rapidly at low temperatures. Under magnetic field of µ0H = 7 T, this increase is significantly suppressed, as shown in the insets of . This infers that the contribution of magnetic moment and carriers through Kondo-like mechanisms plays an important role in the transport properties of CuGa1-xMnxTe2. It was suggested that the Kondo-type interaction can lead the temperature dependent Seebeck coefficient as: S(T) = aT + S0T/(T + T0), with a, S0, and T0 are parameters [Citation52,Citation53]. The broken line in is the fit with the Kondo model. The model appears to explain the overall behaviour. On the other hand, as shown in the main panel of , S is hardly affected by the application of field, possibly because of the too small Zeeman energy of the field of 7 T compared to the antiferromagnetic coupling between carrier and Mn2+ moment, of the order of θ = −100 K. This contradicting behaviour has recently also been observed in Sr2Fe1+xRe1-xO6 double perovskites [Citation54]. It must be emphasized that the thermopower depends on the entropy and on a transport term associated with the band structure [Citation10]. As shown in all the examples presented here, MTEP is associated with MR, but on the other hand the presence of MR is not sufficient to ensure a MTEP effect due to the complex interplay between the entropic and transport terms.
A second similar example is the Cr-doped Bi2Te3 tetradymite system. Bi2Te3 with diluted Cr doping, as well as with transition-metal TM atoms (V, Mn and Fe), have been found to exhibit ferromagnetic transitions, with Cr doping exhibiting relatively high transition temperatures near ~240 K. An effective magnetic moment of 3.53μB is obtained for Bi1.99Cr0.01Te3 consistent with the Cr3+ moment (3.87 μB). In contrast to the Mn2+ doping in CuGa1-xMnxTe2, the Cr3+ doping does not affect the carrier concentration, as predicted theoretically and confirmed experimentally [Citation23,Citation55]. But a TEP enhancement is observed in the case of Bi1.99Cr0.01Te3 compared to the pristine composition and is associated with an increase of the weighted mobility μw from 73.6 cm2 V−1 s−1 to 76.3 cm2 V−1 s−1 in the doped sample with 1%mol Cr. This representative quality parameter of the charge transport can be defined as , where
is the electron mobility,
is the effective mass and
is the electron mass, and the increasing of this parameter supported that an additional mechanism, such as the carrier-magnetic moment interaction, was effective and increased the TEP. As evidence, the non-magnetic isovalent Ga3+ ions substitution for Bi3+ is compared with the same doping level with magnetic Cr3+. Only the magnetic doping with Cr led to an increase of the TEP. Bi1.99Cr0.01Te3 is reported to have a narrow hysteresis loop for the magnetization curves [Citation23]. The relatively small coercivity, (µ0Hc ≈ 0.2 T), and saturation magnetization indicates that the sample is a weak ferromagnet similar to Mn-doped Bi2Te3 [Citation56]. However, the temperature-dependent S(T) of Bi1.99Cr0.01Te3 measured at 0 T, 5T and 9 T () revealed that the TEP is not affected by the magnetic field. It is suggested that the Bi1.99Cr0.01Te3 TEP enhancement is not likely due to a spin fluctuation effect, as reported for the Fe2V0.9Cr0.1Al0.9Si0.1 [Citation57], but by a localized electron-magnetic moment coupling promoting a heavier effective mass m* as experimentally observed [Citation23].
4. Conclusions
A comparative study of MTEP in magnetically doped chalcogenides showing different magnetic behaviour was performed. A significant negative MTEP is observed in ferromagnetic perovskite ruthenates and paramagnetic misfit cobaltites. In the case of ferromagnetic material, below TC, the increase of magnetization is accompanied by a decrease of the entropy of the carriers and hence decreases. On the other hand, no MTEP is observed in quadruple perovskites indicating that Pauli paramagnetism with a very small susceptibility value is less effective in inducing MTEP. In addition, significant MTEP is absent in CuGa1-xMnxTe2 and Bi1.99Cr0.01Te3 where Kondo-type interaction between magnetic impurities and carriers is indicated to prevail. Spin glass system CuCrTiS4 thiospinel also exhibits negative MTEP like in ruthenates and misfit cobaltites. Variable range hopping behaviour of the thiospinel along with negative MR makes the system similar to mixed-valence manganites [Citation35]. Our MTEP study reveals that, in Pauli paramagnetic materials having smallest magnetic susceptibility values, the effect of spin on S is negligible. In contrast magnetism contributes to enhance S in ferromagnetic and some paramagnetic systems, whereas, when diluted, paramagnetism induces MR through a Kondo effect at very low T, but does not induce MTEP. The low temperature magnetic susceptibility of the different materials ranges from ~ 4.10−3 emu/mol for Pauli quadruple perovskites and CuGa1-xMnxTe2 to 0.06–0.2 emu/mol for paramagnetic misfits and thiospinels, respectively, up to 4 emu/mol in SrRuO3. These very different values give a lower limit (~ 10−2 emu/mol) below which MTEP has not been observed.
This investigation suggests that one route to enhance S for TE applications is to find new materials where localized or weakly coupled magnetic ions introduce spin entropy to the system. It is interesting to notice that for such a negative MTEP, the impact of magnetism is maximum in zero magnetic field which is very important for potential applications. The presence of MTEP is a strong indicator of the role these ions play. In that respect, ferrimagnetic systems would also be of interest for MTEP measurements as they combine non-compensated antiferromagnetically coupled ferromagnetic sublattices.
Acknowledgments
The authors acknowledge the support received through ‘Raman-Charpak Fellowship 2019ʹ jointly funded by the Department of Science and Technology (DST), Government of India and the French Institute in India (IFI), French Embassy in India, Ministry for Europe and Foreign Affairs, Government of France. CB, NT and TM acknowledge support from JST Mirai Program JPMJMI19A1.
Disclosure statement
No potential conflict of interest was reported by the author(s).
Additional information
Funding
References
- Bell LE. Cooling, heating, generating power, and recovering waste heat with thermoelectric systems. Science 2008;321:1457–1461.
- Petsagkourakis I, Tybrandt K, Crispin X, et al. Thermoelectric materials and applications for energy harvesting power generation. Sci Technol Adv Mater. 2018;19:836–862.
- Heremans JP, Jovovic V, Toberer ES, et al. Enhancement of thermoelectric efficiency in PbTe by distortion of the electronic density of states. Science. 2008;321:554–557.
- Pei Y, Shi X, LaLonde A, et al. Convergence of electronic bands for high performance bulk thermoelectrics. Nature. 2011;473:66–69.
- Li W, Chen Z, Lin S, et al. Band and scattering tuning for high performance thermoelectric Sn1−xMnxTe alloys. J Materiomics. 2015;1:307–315.
- Jang H, Abbey S, Nam WH, et al. Order-disorder transition-induced band nestification in AgBiSe2–CuBiSe2 solid solutions for superior thermoelectric performance. J Mater Chem A. 2021;9:4648–4657.
- Behnia K, Jaccard D, Flouquet J. On the thermoelectricity of correlated electrons in the zero-temperature limit. J Phys: Condens Matter. 2004;16:5187–5198.
- Wang Y, Rogado NS, Cava RJ, et al. Spin entropy as the likely source of enhanced thermopower in NaxCo2O4. Nature. 2003;423:425–428.
- Mori T. Novel principles and nanostructuring methods for enhanced thermoelectrics. Small. 2017;13:1702013.
- Peterson MR, Mukerjee S, Sriram Shastry B, et al. Dynamical thermal response functions for strongly correlated one-dimensional systems: hubbard and spinless fermion t−V model. Phys Rev B. 2007;76:125110.
- Koshibae W, Maekawa S. Effects of spin and orbital degeneracy on the thermopower of strongly correlated systems. Phys Rev Lett. 2001;87:236603.
- Limelette P, Hébert S, Hardy V, et al. Scaling behavior in thermoelectric misfit cobalt oxides. Phys Rev Lett. 2006;97:046601.
- Repaka DVM, Mahendiran R. Giant magnetothermopower in charge ordered Nd0.75Na0.25MnO3. Appl Phys Lett. 2013;103:162408.
- Wang K, Petrovic C. Large linear magnetoresistance and magnetothermopower in layered SrZnSb2. Appl Phys Lett. 2012;101:152102.
- Niemann AC, Gooth J, Sun Y, et al. Magneto-thermoelectric characterization of a HfTe5 micro-ribbon. Appl Phys Lett. 2019;115:072109.
- Hu J, Caputo M, Guedes EB, et al. Large magnetothermopower and anomalous Nernst effect in HfTe5. Phys Rev B. 2019;100:115201.
- Ito M, Yamashita T, Ebisu S, et al. Thermodynamic and electrical properties of CuCrTiS4. J Alloys Compd. 2014;598:133–136.
- Li J, Tan Q, Li JF. Synthesis and property evaluation of CuFeS2−x as earth-abundant and environmentally-friendly thermoelectric materials. J Alloys Compd. 2013;551:143–149.
- Tsujii N, Mori T. High thermoelectric power factor in a carrier-doped magnetic semiconductor CuFeS2. Appl Phys Exp. 2013;6:043001.
- Ang R, Khan AU, Tsujii N, et al. Thermoelectricity generation and electron-magnon scattering in a natural chalcopyrite mineral from a deep-sea hydrothermal vent. Angew Chem Int Ed. 2015;54:12909–12913.
- Berthebaud D, Lebedev OI, Maignan A, et al. Magnetothermopower and giant magnetoresistance in the spin-glass CuCrTiS4 thiospinel. J Appl Phys. 2018;124:063905.
- Ahmed F, Tsujii N, Mori T. Thermoelectric properties of CuGa1−xMnxTe2: power factor enhancement by incorporation of magnetic ions. J Mater Chem A. 2017;5:7545–7554.
- Vaney JB, Yamini SA, Takaki H, et al. Magnetism-mediated thermoelectric performance of the Cr-doped bismuth telluride tetradymite. Mat Today Phys. 2019;9:100090.
- Maignan A, Hébert S, Hervieu M, et al. Magnetoresistance and magnetothermopower properties of Bi/Ca/Co/O and Bi(Pb)/Ca/Co/O misfit layer cobaltites. J Phys: Condens Matter. 2003;15:2711–2723.
- Klein Y, Hébert S, Maignan A, et al. Insensitivity of the band structure of substituted SrRuO3 as probed by Seebeck coefficient measurements. Phys Rev B. 2006;73:052412.
- Hébert S, Daou R, Maignan A. Thermopower in the quadruple perovskite ruthenates. Phys Rev B. 2015;91:045106.
- Hejtmánek J, Jirák Z, Maryško M, et al. Interplay between transport, magnetic, and ordering phenomena in Sm1−xCaxMnO3. Phys Rev B. 2000;60:14057.
- Koroleva LI, Kessler YA, Lukina LN, et al. New magnetic semiconductors Fe1−xCr2(1−x)Sn2xS4. J Magn Magn Mater. 1996;157-158:475–476.
- Abramovich AI, Koreleva LI, Lukina LN. Spin-glass and reentrant spin-glass states in iron sulfospinels having dilute A and B sublattices. Phys Solid State. 1999;41:73–79.
- Ito M, Furuta T, Terada N, et al. Relaxation of magnetization in spinel CuCrZrS4. Physica B. 2012;407:1272–1274.
- Lotgering FK. Ferromagnetism in spinels: cuCr2S4 and CuCr2Se4. Solid State Commun. 1964;2:55–56.
- Matsumoto N, Hagino T, Taniguchi K, et al. Electrical and magnetic properties of CuTi2S4 and CuZr2S4. Physica B. 2000;284-288:1978–1979.
- Nagata S, Koseki N, Ebisu S. Spin-glass in the spinel-type CuCrTiS4. Philos Mag B. 2012;92:2957–2969.
- Snyder GJ, Caillat T, Fleurial JP. Thermoelectric properties of chalcogenides with the spinel structure. Mater Res Innovations. 2001;5:67–73.
- Viret M, Ranno L, Coey JMD. Magnetic localization in mixed-valence manganites. Phys Rev B. 1997;55:8067.
- Bobroff J, Hébert H, Lang G, et al. Interplay between magnetic properties and thermoelectricity in misfit and Na cobaltates. Phys Rev B. 2007;76:100407.
- Doumerc JP. Thermoelectric power for carriers in localized states: a generalization of Heikes and Chaikin-Beni formulae. J Solid State Chem. 1994;110:419–420.
- Koshibae W, Tsutsui K, Maekawa S. Thermopower in cobalt oxides. Phys Rev B. 2000;62:6869.
- Hébert S, Kobayashi W, Muguerra H, et al. From oxides to selenides and sulfides: the richness of the CdI2 type crystallographic structure for thermoelectric properties. Phys Status Solidi A. 2013;210:69–81.
- Pollet M, Doumerc J-P, Guilmeau E, et al. Does the orbital degeneracy play any role in the high thermopower of lamellar cobaltites? J Appl Phys. 2007;101:083708.
- Landron S, Lepetit MB. Importance of t2g−eg hybridization in transition metal oxides. Phys Rev B. 2008;77:125106.
- Cao G, Chikara S, Lin XN, et al. Itinerant ferromagnetism to insulating antiferromagnetism: a magnetic and transport study of single crystal SrRu1−xMnxO3(0⩽x<0.60). Phys Rev B. 2005;71:035104.
- Kobayashi H, Nagata M, Kanno R, et al. Structural characterization of the orthorhombic perovskites: [ARuO3 (A = Ca, Sr, La, Pr)]. Mater Res Bull. 1994;29:1271.
- Cao G, McCall S, Shepard M, et al. Thermal, magnetic, and transport properties of single-crystal Sr1−xCaxRuO3 (0<~x<~1.0). Phys Rev B. 1997;56:321.
- Mravlje J, Georges A. Thermopower and entropy: lessons from Sr2RuO4. Phys Rev Lett. 2016;117:036401.
- Yamamoto TD, Taniguchi H, Yasui Y, et al. Magneto-thermopower in the weak ferromagnetic oxide CaRu0.8Sc0.2O3: an experimental test for the kelvin formula in a magnetic material. J Phys Soc Jp. 2017;86:104707.
- Xiang H, Liu X, Zhao E, et al. First-principles study on the conducting mechanism of the heavy-fermion system CaCu3Ru4O12. Phys Rev B. 2007;76:155103.
- Allen PB, Berger H, Chauvet O, et al. Transport properties, thermodynamic properties, and electronic structure of SrRuO3. Phys Rev B. 1996;53:4393.
- Klein L, Dodge JS, Ahn CH, et al. Transport and magnetization in the badly metallicitinerant ferromagnet SrRuO3. J Phys: Condens Matter. 1996;8:10111–10126.
- Tyler AW, Mackenzie AP, Nishizaki S, et al. High-temperature resistivity of Sr2RuO4: bad metallic transport in a good metal. Phys Rev B. 1998;58:R10107.
- The values m* shown here are larger than those reported in ref. [17], where the degenerate limit has been assumed. The present calculation based on a parabolic band model suggested that the Lorenz numbers of CuGa1-xMnxTe2 are L = 1.5 to 1.8×10−8 WΩK−2, thereby suggesting the degenerate limit was not appropriate for this case.
- Kondo J. Theory of dilute magnetic alloys. Solid State Phys. 1970;23:183–281.
- Osinniy V, Dybko K, Jedrzejczak A, et al. Thermoelectric studies of electronic properties of ferromagnetic GaMnAs layers. Semicond Phys, Quantum Electron Optoelectron. 2008;11:257–265.
- Maignan A, Martin C, Lebedev OI, et al. Sr2Fe1+xRe1−xO6 double perovskites: magnetoresistance and (magneto)thermopower. Chem Commun. 2019;55:5878–5881.
- Zhang JM, Ming W, Huang Z, et al. Stability, electronic, and magnetic properties of the magnetically doped topological insulators Bi2Se3, Bi2Te3, and Sb2Te3. Phys Rev B. 2013;88:235131.
- Hor YS, Roushan P, Beidenkopfet H, et al. Development of ferromagnetism in the doped topological insulator Bi2−xMnxTe3. Phys Rev B. 2010;81:195203.
- Tsujii N, Nishide A, Hayakawa J, et al. Observation of enhanced thermopower due to spin fluctuation in weak itinerant ferromagnet. Sci Adv. 2019;5:5935.