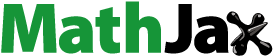
ABSTRACT
The present study aims to develop a layered zirconium phosphate/phosphonate (LZP) powder to control the release of therapeutic inorganic ions. Organically modified LZPs were successfully prepared with various contents of phenyl groups via a reflux method in an aqueous solution containing phosphoric and phenylphosphonic acids. Powder X-ray diffraction analysis and Fourier transform infrared spectroscopy revealed that the crystal structure of the synthesized LZP samples was identical to that of α-zirconium phosphate, even after modification. The amount of incorporated organic molecules increased with increasing molar fractions of phenylphosphonic acid in the starting composition, as determined from the thermal analysis. Cobalt ion (Co2+), a type of therapeutic inorganic ion, was incorporated into the organically modified LZP through treatment with an acetonitrile solution containing tetrabutylammonium ions, followed by treatment with an acetonitrile solution containing CoCl2. The amount of incorporated Co2+ depended on the concentration of the phenyl groups. Furthermore, the highest amount of Co2+ was incorporated in the sample (ZP-Ph-0.5) prepared with equimolar phosphoric/phenylphosphonic acid. The ZP-Ph-0.5 sample additionally showed the ability to incorporate copper or iron ions (Cu2+ or Fe3+). The incorporated ion, either Co2+ or Cu2+, was continuously released from the ZP-Ph-0.5 sample in a saline solution over a period of three weeks, whereas the release of Fe3+ was negligible. The quantity of Co2+ released was higher than that of Cu2+. The controlled release of Co2+ from the ZP-Ph-0.5 sample was also observed in a simulated body fluid that mimicked the ionic concentration of human blood plasma. These results confirm that a specific degree of phenyl modification makes LZP a candidate host material for releasing therapeutic inorganic ions.
Graphical abstract
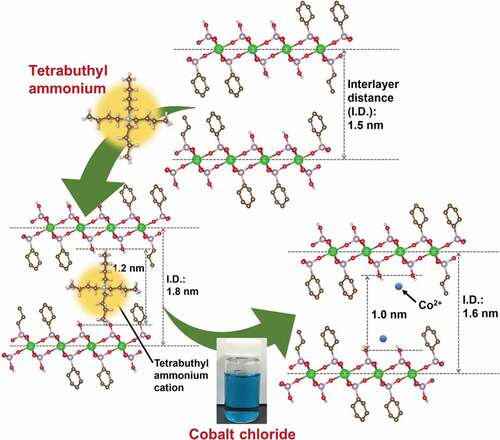
1. Introduction
Ceramic biomaterials are a class of materials that exhibit a specific biological affinity for biological tissues; they can be used for the reconstruction of living tissues. Bioactive ceramics have been used as materials to rectify bone defects by direct bonding with living bone after implantation at the site of defect. They have also been recognized as effective osteoconductive materials [Citation1]. Bioactive glasses and glass-ceramics have been studied as biomaterials that show osteoconductivity after implantation at the site of defect; that is, they possess bone-bonding properties after implantation. Biomaterials with bone-bonding properties have been studied as bone substitutes. In recent years, there has been a growing demand for biomaterials that can enhance tissue regeneration and that possess antibacterial properties [Citation2,Citation3]. In the design of these biomaterials, controlled release of therapeutic inorganic ions and organic molecules is desired [Citation4]. Although ceramics have been modified to incorporate organic molecules and inorganic ions as biological factors to enhance bone formation or to impart antibacterial properties, it has been challenging to achieve such physiological effects efficiently using conventional bioactive ceramic materials [Citation5]. Therefore, the development of novel biomaterials with controlled release of therapeutic inorganic ions, as well as biologically active molecules, is necessary. As a host material for releasing biological factors, inorganic materials with layered structures have received considerable attention for controlled dissolution of the factors through the organic modification of its interlayer.
Various inorganic layered compounds, such as clays [Citation6,Citation7], layered double hydroxides [Citation8,Citation9], and layered phosphates [Citation10,Citation11] have been explored as biomaterials for drug delivery carriers and gene reservoirs. Among the layered compounds, the focus of this study is α-zirconium phosphate (α-ZrP: Zr(HPO4)2·H2O). This is because α-ZrP is made of inorganic constituents that have been intensely investigated with diverse biomedical applications, such as zirconia and phosphate. The present authors previously reported that α-ZrP and its organically modified derivatives induced adhesion of human stem cells, comparable to that of hydroxyapatite, a typical ceramic biomaterial [Citation12]. α-ZrP is a type of layered zirconium phosphate that allows the incorporation of inorganic ions by ion exchange; furthermore, it facilitates the organic modification of the interlayers [Citation13,Citation14]. Thus, the organic modification of layered zirconium phosphate could lead to improved incorporation and controlled release of therapeutic inorganic ions. Although previous studies have reported the incorporation of inorganic elements into the interlayer in layered zirconium phosphate/phosphonate (LZP) [Citation15,Citation16], there have been few studies on the incorporation of therapeutic inorganic ions into the organically modified LZP. Furthermore, studies on its ion-releasing behavior are rare. These properties demonstrate potential for the development of substrates for novel delivery systems.
Among the therapeutic inorganic ions, cobalt ion (Co2+) was the focus here because of its angiogenic stimulatory effect on vascular endothelial cells [Citation17–19]. The purpose of this study is to investigate the Co2+ incorporation capability of organically modified LZPs depending on the degree of modification, and to examine their ion-releasing properties. Moreover, the ability of the LZPs to incorporate copper or iron ions (Cu2+ or Fe3+) and, furthermore, their ion-release properties for these constituents were compared with those of Co2+. Organically modified LZP powders were prepared from the starting materials with varying amounts of phenylphosphonic acid. The present authors hypothesize that partial modification of the phosphate group with hydrophobic phenyl groups will impede the rate of ion exchange. The controlled release of Co2+, Cu2+, and Fe3+ from LZP will be the outcome. The incorporation of Co2+ into each organically modified LZP powder was then attempted using tetrabutylammonium (TBA)-modified LZP. Furthermore, the release behavior of the incorporated Co2+, Cu2+, and Fe3+ ions from each organically modified LZP was investigated in a saline solution.
2. Experiments
2.1. Preparation of organically modified LZP
For the synthesis of α-ZrP and its derivatives under suitable conditions with appropriate modifications, the preparation procedures were according to a previous report [Citation15]. All chemicals were used as received without further purification. Zirconyl chloride octahydrate (5.0 g; ZrOCl2 · 8H2O, Kanto Chemical, Japan), and appropriate amounts of phosphoric acid solution (85%; H3PO4, Nacalai Tesque, Japan) and phenylphosphonic acid (C6H5PO3H, Tokyo Chemical Industry, Japan), were added to 48 mL of deionized water. The P/Zr molar ratio of the starting material is two. The mixture was refluxed at 100°C for 24 h with stirring. The product was centrifuged at 6500 × g for 10 min (MX-301, TOMY SEIKO, Japan) and upon discarding the supernatant, dispersed in deionized water (40 mL) through sonication. These clean-up steps were repeated three times. Subsequently, the sample was dried for 1 d at 65°C in a drying oven (DRYING OVEN DO-300, IUCHI, Japan), followed by pulverization. Hereafter, the samples are denoted as ZP-Ph-x, where x equals 0, 0.25, 0.50, 0.75, or 1.0, as given in .
Table 1. Prepared samples with different amounts of phenyl group
Powder X-ray diffraction (XRD) patterns of the ZP-Ph-x samples were obtained using Cu Kα radiation on an X-ray diffractometer (RINT-2100/PC, Rigaku, Japan) at scanning rates of 1 and 0.5°/min for measurement ranges 2 θ = 2–40° and 2–10°, respectively, to observe in detail the changes in the interlayer distance (ID). The samples were also characterized by differential thermal and thermogravimetric analysis (TG-DTA, DTG-60, Shimadzu, Japan) as well as Fourier transform infrared spectroscopy (FTIR, FT/IR-6100, Jasco, Japan) using the KBr pellet method. The temperatures at the onset and end of mass loss were estimated by extrapolation of the thermogravimetric (TG) curves (denoted hereafter as the extrapolation method) unless specifically mentioned. The temperatures were estimated from the intersecting points between a tangential line drawn to the steepest point of the mass loss and the extrapolated baseline before and after mass loss. The morphologies of the samples were observed using a transmission electron microscope (TEM, JEM-2100Plus, JEOL, Japan). The specific surface area of the ZP-Ph-x particles was measured using the Brunauer Emmett Teller (BET) nitrogen adsorption method (Nova 1000e, Quantachrome Instruments, USA).
2.2. Incorporation of cobalt, copper, and iron into ZP-Ph-x samples and evaluation of their releasing behavior
The incorporation of cobalt into the samples was carried out through the subsequent addition of an acetonitrile solution containing CoCl2 to the ZP-Ph-x powder treated with an acetonitrile solution containing TBA. Specifically, after 0.2 g of ZP-Ph-x was dispersed in a solution (TBA solution) of acetonitrile (CH3CN, Fujifilm Wako Pure Chemical, Japan) and tetrabutylammonium hydroxide (40%, (C4H9)4NOH, Tokyo Chemical Industry, Japan) at a volume ratio of 19.5:0.5 (mL), the slurry was kept at approximately 25°C. After standing for 2 d, the powder was collected by centrifugation at 7300 × g for 10 min and characterized by powder XRD.
To achieve the incorporation of Co2+ into the ZP-Ph-x samples, a CoCl2 solution was prepared by dissolving cobalt (II) chloride hexahydrate (7.1 mg) (CoCl2 · 6H2O, Nacalai Tesque, Japan) in acetonitrile (20 mL). This resulted in the concentration of Co2+ in the solution being 1.5 mmol·L−1, which was subsequently added to the centrifuged samples after treatment with the TBA solution. Specifically, the sample were dispersed in the aforementioned CoCl2 solution by shaking at approximately 25°C using a shaking bath (LT-10 F, Taitec, Japan). After shaking for 1 d, the sample powders were collected by centrifugation at 7300 x g for 10 min. The precipitates were analyzed using powder XRD, whereas the concentration of residual Co2+ in the supernatant was determined using inductively coupled plasma atomic emission spectroscopy (ICP-AES, Optima 2000 DV, PerkinElmer, USA). The samples for the evaluation of yield after treatment with TBA solution followed by centrifugation were separately prepared following the described procedures and then dried at 40°C for 3 d.
The incorporation of Cu2+ or Fe3+ was monitored using ultraviolet–visible (UV–vis, V-670, Jasco, Japan) absorption spectrometry. Copper acetate (II) monohydrate ((CH3COO)2Cu·H2O, Fujifilm Wako Pure Chemical, Japan) and iron (III) chloride hexahydrate (FeCl3·H2O, Kishida Chemical, Japan) were used as starting materials for the incorporation of Cu2+ and Fe3+, respectively, into ZP-Ph-0.5, which showed a high capability of incorporating Co2+. The incorporated amounts of Cu2+ and Fe3+ were estimated from the concentration of residual Cu2+ and Fe3+ in the supernatant, as determined from the absorbance at 252 and 370 nm for Cu2+ and Fe3+, respectively, in the UV–vis absorption spectra.
The release behavior of Co2+, Cu2+, and Fe3+ from ZP-Ph-0.5 incorporated with these ions was evaluated in a saline solution with a NaCl concentration of 0.9 wt%. Each sample powder (20 mg) was immersed in a saline solution (10 mL) and placed in an incubator (MIR-162, SANYO, Japan) at 37°C for a certain period (2, 4, 6, 8, 24, 72, and 168 h). The powders were collected through centrifugation at 7300 × g for 10 min and dispersed in a renewed 0.9 wt% NaCl solution (10 mL) through sonication, whereas the concentrations of Co2+, Cu2+, and Fe3+ in the supernatant were determined using ICP-AES. The release behavior was monitored through changes in the concentration of ions in the supernatants obtained after each immersion period (2, 4, 6, 8, 24, 72, and 168 h).
The release behavior of Co2+ from the ion-incorporated ZP-Ph-0.5 was also investigated at 37°C in both a simulated body fluid (SBF, pH 7.4) and water using a procedure similar to that for saline. To prepare the SBF, ultrapure water (700 mL) was added to a beaker. The reagents listed in Table S1 were then dissolved in the order listed under stirring. Each reagent was completely dissolved before the addition of the following reagents. The pH of the solution was adjusted to 7.4 by adding 1.0 mol·L−1 hydrochloric acid at 36.5°C; the solution was then transferred to a volumetric flask and ultrapure water was added to adjust the total volume to 1 L. The SBF or water was renewed at immersion periods of 2, 4, 6, 8 and 24 h.
0.05 g of ZP-Ph-0.5 powder incorporated with Co2+ was dispersed in 1 mL of ethanol via sonication. A sub-sample of the obtained dispersion (50 µL) was spread on one side of a polyethylene disc (PE disc, diameter 14 mm, PEN-050501, AS ONE Co., Japan) preheated at 90°C on a hot plate (HHP-140D, AS ONE Co., Japan). The amount of sample powder on a PE disk was adjusted to be 2.5 mg. The substrate was then hot-pressed using hotplate and stainless-steel weight blocks (350 MPa for 30 s at 140°C). The prepared substrate was placed in each well of a 24-well plate (Cellstar 662,160, Greiner Bio-One International, Austria) with the powder-embedded side facing upward. Subsequently, 1.25 mL of the SBF or saline was added to each well. The 24-plate was placed in an incubator (EL-600 V, AS ONE Co., Japan) for 24 h at 37°C, and the concentrations of Co2+ in the supernatants were measured using ICP-AES.
3. Results
3.1. Characterization of the prepared ZP-Ph-x powders
shows the powder XRD patterns of the prepared ZP-Ph-x samples. The diffraction pattern of ZP-Ph-0, which is free from organic modification, shows 002, 110, 112, and 06 reflection peaks that were assigned to α-ZrP according to the International Centre for Diffraction Data card ICDD 33–1482. The diffraction patterns of the sample powders subjected to organic modification, that is, x = 0.25, 0.50, 0.75, and 1.0, were assigned according to ICDD 44–2000. The sample powders subjected to organic modification showed a 002 reflection shift from 2θ = 11° to approximately 2θ = 6°. Broad reflection peaks were observed in the range 2θ of 20–40°, corresponding to the 111,
04,
06, and
11 reflections of zirconium phenylphosphonate. The lowered shift of the 002 diffraction peak was caused by the increase in ID with organic modification (the incorporation of phenyl groups), even in the ZP-Ph-0.25 sample. The higher concentration of phenyl groups in the organic modification process led to a higher intensity of 002 reflection even after organic modification, as can be seen in the diffraction patterns of ZP-Ph-0.75 and ZP-Ph-1.0. The estimated ID in ZP-Ph-0 (α-ZrP) was 0.8 nm, whereas that in ZP-Ph-1.0 was 1.6 nm. Disordered crystalline structures were observed for ZP-Ph-0.25 and ZP-Ph-0.5.
Figure 1. Powder XRD patterns of the ZP-Ph-x samples. Spectra are vertically offset for presentation purposes
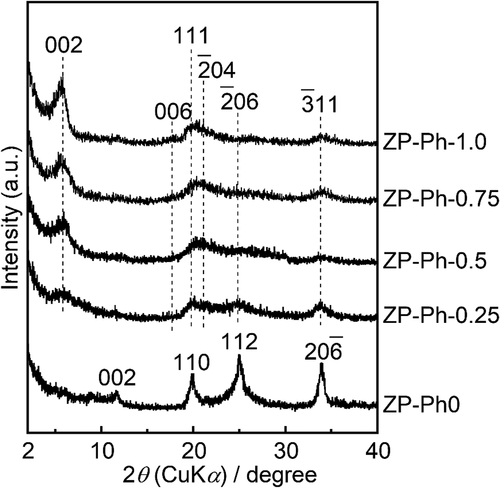
shows the FTIR spectra of the prepared ZP-Ph-x samples. ZP-Ph-0 showed broad absorption peaks with wavenumbers between 950 and 1100 cm−1, which were assigned to P-O vibrations. The absorption peak centered at 1050 cm−1 was assigned to the P-O symmetric stretching vibration, whereas that at 960 cm−1 corresponds to the O-P-O bending vibration. The organically modified samples ZP-Ph-0.25, ZP-Ph-0.5, ZP-Ph-0.75, and ZP-Ph-1.0 showed absorption peaks at 750, 1156, and 3061 cm−1, which were assigned to C-H, P-C, and C-H bonds in the phenylphosphonic component, respectively. The absorption intensities for these peaks increased with increasing amounts of phenylphosphonic acids in the starting composition in the following order: ZP-Ph-0.25 < ZP-Ph-0.5 < ZP-Ph-0.75 < ZP-Ph-1.0. Conversely, the intensity of the absorption peak at 1245 cm−1, which was assigned to P-OH bonds, decreased with increasing amounts of phenylphosphonic acid in the starting compositions. The peaks at 1450 cm−1 correspond to the stretching of the C = C bonds in the phenyl group. The peak at 570 cm−1 was assigned to the bending vibration of the PO3 group. The peaks at 610 and 520 cm−1 were assigned to the deformation of the PO4 group. Among the ZP-Ph-X samples, ZP-Ph-1.0 had the lowest relative intensity of the peak at 520 cm−1. This suggests that the quantity of PO4 groups in ZP-Ph-1.0 was minimized corresponding to the preparation composition.
Figure 2. FTIR spectra of the ZP-Ph-x samples. Spectra are vertically offset for presentation purposes
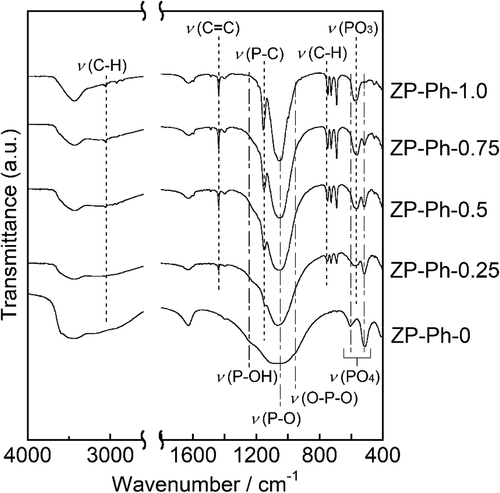
shows the TEM images of the prepared ZP-Ph-x samples. All prepared ZP-Ph-x samples (x = 0, 0.25, 0.50, 0.75, and 1.0) showed similar morphologies, that is, the aggregates of the particles had diameters of ~10 nm. The specific surface area (SSA) of these samples was measured as 21, 103, 105, 155 and 146 m2/g for ZP-Ph-0, ZP-Ph-0.25, ZP-Ph-0.5, ZP-Ph-0.75, and ZP-Ph-1.0, respectively. shows the TG (solid line) and differential thermal analysis (DTA, dashed line) curves of the prepared ZP-Ph-x samples. ZP-Ph-0 showed a significant mass loss below 150°C, accompanied by an endothermic peak, which was attributed to elimination of water from the interlayers of the α-ZrP crystal [Citation20]. Thus, the mass losses of the prepared samples below 150°C were ascribed to the removal of water. ZP-Ph-0 showed a gradual mass loss with a broad endothermic peak at 500–800°C, which could be ascribed to the dehydration of -OH groups at the interlayer to form pyrophosphate (P-O-P) groups. The TG and DTA curves of the samples prepared with phenylphosphonic acid also showed notable mass loss between 420 and 650°C accompanied by exothermic peaks, which were attributed to the combustion of the organic components of phenyl-modified zirconium phosphate/phosphonate under ambient conditions. Based on the TG results, the mass losses caused by the removal of water and combustion of organic components were estimated using the extrapolation method; the results are summarized in . The initial temperature of the TG analysis (35°C) was considered as the onset temperature for the removal of water because it was difficult to find the baseline for the method. When the fraction of phenylphosphonic acid in the starting composition increased, the fraction of the lost water mass decreased, whereas conversely, the fraction of the combusted organic components increased.
Table 2. Estimated mass losses corresponding to water molecules and organic functional groups in the samples. The estimated temperature ranges were determined by the extrapolation method
3.2. Incorporation of cobalt into ZP-Ph-x samples
shows the powder XRD patterns of the samples immersed in an acetonitrile solution containing TBA (TBA solution) for 2 d ()), as well as those of the samples subsequently treated with the CoCl2 solution following treatment with the TBA solution ()). For the ZP-Ph-0 sample, the reflection peaks appeared at 2θ = 5.1° and 10.1° (d = 1.8 and 0.9 nm, respectively); these peaks were attributed to the 002 and 004 reflections of α-ZrP after intercalation of the TBA molecule [Citation21]. A decrease in intensity of the peak at 2θ = 11.7°, which was assigned to the 002 reflection of α-ZrP, was observed. The other peaks of ZP-Ph-0 treated with TBA solution showed a slight decrease in intensity without any shifts compared to those of the as-prepared ZP-Ph-0. The pattern of ZP-Ph-0 subsequently treated with CoCl2 showed a decrease in intensity of the peak attributed to the 002 and 004 reflections. The intensity of peaks attributed to the 002 reflections in ZP-Ph-0.25, ZP-Ph-0.5, ZP-Ph-0.75, and ZP-Ph-1.0 decreased upon treatment with TBA solution. These reductions are considered to originate from the swelling of the interlayer due to the treatment. After treatment with CoCl2, the ZP-Ph-0, ZP-Ph-0.25, ZP-Ph-0.50, ZP-Ph-0.75, and ZP-Ph-1.0 samples showed no significant decrease in the intensities of the 002, 110, 112, and 06 peaks.
Figure 5. Powder XRD patterns of the samples soaked in acetonitrile solution containing TBA (TBA-solution) for 2 d, as well as those of the samples treated with the TBA-solution followed by CoCl2 solution (CoCl2 solution). (a) samples treated with TBA-solution. (b) samples treated with TBA-solution followed by treatment with CoCl2 solution. Spectra are vertically offset for presentation purposes
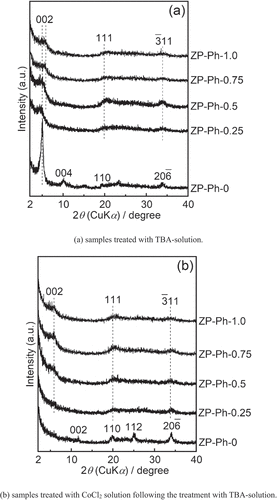
For a detailed discussion on the changes in the ID of ZP-Ph-x (x = 0.25–1.0), slowly scanned profiles ranging from 2θ = 2° to 10° were investigated (). The powder XRD patterns of the as-prepared samples, samples treated with TBA solution, and samples treated with CoCl2 solution were compared. In the XRD profile of ZP-Ph-0.25, a broad diffraction peak at 2θ = 6.2° was observed. Another broad peak at approximately 2θ = 4.6° appeared after the treatment with TBA solution. Moreover, the ZP-Ph-0.25 sample treated with CoCl2 showed a broad peak at approximately 2θ = 5.7°. For ZP-Ph-0.5, a broad peak appeared at 2θ = 5.8°, and another broad peak was observed at approximately 2θ = 5.4° after CoCl2 treatment. The peak appearing at 2θ = 5.0° was assigned to the enlargement of ID after treatment with TBA solution. An increase in the intensity of the peak at approximately 2θ = 6° was observed with an increase in the quantity of organic groups in the interlayer in as-prepared samples. The appearance of broad peaks at approximately 2θ = 5° on ZP-Ph-0.25, ZP-Ph-0.5, and ZP-Ph-0.75 after treatment with TBA solution indicated that TBA was intercalated in the interlayer. However, the ID of the ZP-Ph-0.25 and ZP-Ph-0.5 samples treated with CoCl2 exhibited diffraction angles similar to those of the as-prepared samples. Fig. S1 shows the FTIR spectra of ZP-Ph-0.5 after treatment with TBA solution and after TBA treatment followed by CoCl2. The sample treated with TBA solution showed absorption peaks at 1382, 1462, and 1486 cm−1, which were assigned to the C-H bond in the TBA molecules. These peaks were absent for the CoCl2 treated sample indicating that partial organic modification with phenyl groups allowed the intercalation of TBA molecules, and TBA was de-intercalated after treatment with CoCl2. ZP-Ph-1.0 showed a small peak shift after treatment with TBA solution, along with an increase in the peak width. This result suggests that the organically modified layer was strongly stacked during the treatment with TBA. The masses of the dried powder samples after treatment with TBA followed by centrifugation were measured. The masses were 0.20, 0.09, 0.06, 0.05 and 0.15 g for ZP-Ph-0, ZP-Ph-0.25, ZP-Ph-0.5, ZP-Ph-0.75 and ZP-Ph-1.0, respectively.
Figure 6. Powder XRD patterns of the as-prepared samples (as-prepared), the samples with the TBA-treatment (TBA-solution), and the samples treated with TBA-solution followed by CoCl2 solution (CoCl2 solution). (a) ZP-Ph-0.25, (b) ZP-Ph-0.5, (c) ZP-Ph-0.75, (d) ZP-Ph-1.0 and (e) summary of calculated interlayer distances. Spectra are vertically offset for presentation purposes
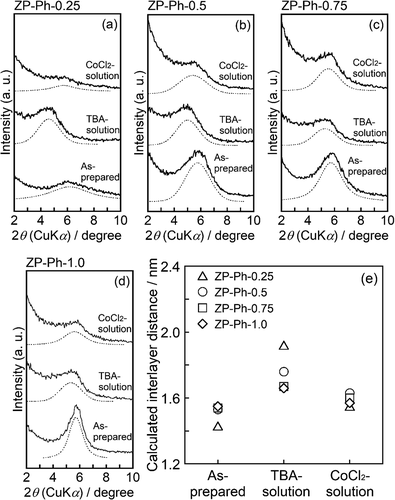
The supernatants from the CoCl2 treatment step were analyzed using ICP-AES to determine the Co2+ content in the samples. shows the relationship between the contents of Co2+ and phenyl groups in the organically modified LZPs, as estimated from the TG curves of the samples. Maximum Co2+ content was observed in ZP-Ph-0.5: 0.3 mmol·g−1. Therefore, ZP-Ph-0.5 was chosen as the sample for loading ions and for evaluating the ion-releasing behavior.
3.3. Release of inorganic ions from ZP-Ph-0.5
Cu2+ and Fe3+ were incorporated into ZP-Ph-0.5 by immersing the sample in acetonitrile solutions containing either copper acetate (Cu(CH3COO)2) or iron chloride (FeCl3), respectively, following TBA treatment. shows the XRD patterns of the obtained samples. The samples treated with the Cu(CH3COO)2 or FeCl3 solutions showed peaks at 2θ = 5.1 or 5.4° (d = 1.7 or 1.6 nm), respectively. These diffraction angles were close to those of ZP-Ph-0.5 processed with CoCl2 (). Moreover, the ID in this case was shorter than that after treatment with TBA (2θ = 5.0°, d = 1.8 nm). Therefore, the introduction of Cu2+ and Fe3+ in the interlayer could have occurred. After measuring the residual ion concentrations in the solution by UV–vis spectrometry, the determined amounts of Cu2+ and Fe3+ incorporated into 1 g of ZP-Ph-0.5 were 1.0 and 0.5 mmol·g−1, respectively.
Figure 8. Powder XRD patterns of the ZP-Ph-0.5 treated with TBA-solution followed by Cu(CH3COO)2 solution or FeCl3 solution
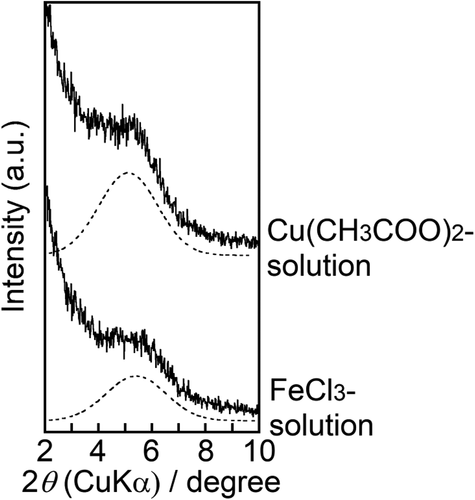
The samples incorporated with Co2+, Cu2+, and Fe3+ were immersed in a saline solution that was changed at certain specific periods. shows the Co2+, Cu2+, and Fe3+ concentrations of the saline after sample immersion. The filled and meshed bars indicate the concentrations for the individual immersion period and accumulated concentrations, respectively. Co2+ showed rapid dissolution within 2 h of immersion. The concentration of Co2+ reached 0.242 mmol·L−1. Sustained release of Co2+ was observed from 4 to 672 h after immersion. The release rate was in the range 0.1–0.5 µmol·L−1·h−1 after 24 h of immersion. Similarly, the sustained release of Cu2+ was observed following rapid dissolution for up to 2 h after immersion. The releasing rate of Cu2+ was approximately 2 µmol·L−1·h−1 between 24 and 72 h after immersion, which then decreased to 0.1–0.5 µmol·L−1·h−1. The release of Fe3+ from the samples was negligible.
Figure 9. Release behaviors of ions from the ZP-Ph-0.5 which was treated with TBA-solution followed by CoCl2 solution (Co), Cu(CH3COO)2 solution (Cu) or FeCl3 solution (Fe)
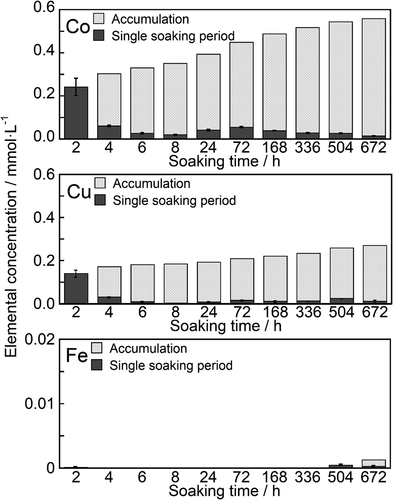
The release behavior of Co2+ from ZP-Ph-0.5 was also evaluated by immersion in SBF and water for 24 h. shows the concentrations of Co2+ in SBF and water after immersion of the samples. The concentration reached 0.074 mmol·L−1 within the initial 2 h. Sustained release of Co2+ was observed from 2 h to 24 h after immersion. The release rate was in the range 2–37 µmol·L−1·h−1. The release of Co2+ from water was negligible throughout the experimental period.
To investigate the effect of polymer embedding on ion release, Co2+-incorporated ZP-Ph-0.5 powder was hot-pressed onto one side of the PE substrate, and then immersed in SBF or saline. The Co2+ concentrations in the SBF and saline reached 0.062 and 0.125 mmol·L−1, respectively, after immersion of the substrates for 24 h.
4. Discussion
Layered materials have structures wherein heterolayers with a thickness of a few atomic layers are stacked via weak interactions, such as electrostatic forces with counter-charged ions or hydrogen bonding with water molecules. Because of the structural flexibility at the interlayer, organic drug molecules and inorganic ions of various sizes can be inserted between the layers on the basis of ion-exchange chemistry. Organically modified LZPs have been explored as excellent host materials to incorporate guest organic molecules via intercalation [Citation22–24] or ion exchange [Citation15,Citation25,Citation26]. The availability of acidic groups such as phosphate and sulfonate (SO3H) groups in the organically modified LZP is key for the uptake of inorganic ions caused by the ion-exchange property. Organically modified LZP with available acidic groups has been prepared using a mixture of phosphoric and aromatic phosphonic acids as the starting materials [Citation15] and through post-synthesis sulfonation of the aromatic groups [Citation26]. Here, phenyl-modified zirconium phosphate/phosphonate was prepared using a mixture of phosphoric and phenylphosphonic acids at various molar fractions.
From the XRD results, the ID calculated from the peak of the 002 reflection was 1.48 nm in the sample synthesized using phenylphosphonic acid as the starting material (ZP-Ph-1.0). This ID was identical to the distance of zirconium phenylphosphonate (Zr(C6H5PO3)2) reported by Alberti et al. [Citation27]. Furthermore, broad peaks observed at 2θ = 19.5°, 21.1°, 24.8°, and 33.5° were known to originate from 111, 04,
06, and
11 of zirconium phenylphosphonate (ICDD 44–2000), respectively, as reported by Poojary et al. [Citation28]. These results indicate that ZP-Ph-1.0 has a structure in which a layered skeleton of zirconium phosphate/phosphonate is laminated by a phenyl group. ZP-Ph-0.75, ZP-Ph-0.5, and ZP-Ph-0.25 showed diffraction peaks at angles identical to those of ZP-Ph-1.0. The presence of phenyl groups was also confirmed by the presence of ν(P-C) and ν(C-H) peaks in the FTIR spectrum. These results collectively indicate the successful formation of phenyl-modified LZPs at various molar fractions of phenylphosphate/phosphoric acid in the starting materials. lists the compositions of the samples estimated from the TG-DTA data in . The estimated composition of the C6H5PO3 group in the samples decreased to 60%–70% of the theoretical composition estimated from the compositions of the starting materials. Moreover, the FTIR spectra of the samples showed a broad peak at approximately 3500 cm−1, which corresponds to the ν(O-H) of the phosphate groups. These results indicate that the content of phenyl groups in the prepared samples increased with increasing molar fraction of the starting materials. In addition, some of the phenyl groups underwent hydrolysis to form phosphate (PO-H) groups during sample preparation.
Table 3. Compositions estimated from mass fractions corresponding to water molecules and organic functional groups in the samples listed in
The ions of therapeutic inorganic elements, such as cobalt, were introduced into the samples, which were mediated by the temporal insertion of TBA cations. The IDs of the organically modified LZPs increased after immersion in TBA solution, whereas the extent of increase heightened as the quantity of phosphate groups rose in the prepared samples. The increase in ID followed the order of ZP-Ph-0 (increase of 1.0 nm) > ZP-Ph-0.25 (increase of 0.5 nm) > ZP-Ph-0.5 (increase of 0.3 nm) > ZP-Ph-0.75 (increase of 0.1 nm) ˃ ZP-Ph-1.0 (increase of 0.1 nm). Hence, the increase in ID was attributed to the exchange of protons at the phosphate groups with the TBA cations. ZP-Ph-0.25, ZP-Ph-0.5, and ZP-Ph-0.75 showed relatively small yields after treatment with TBA solution, followed by centrifugation. These phenomena correspond to the elimination of exfoliated products during centrifugation. The ZP-Ph-1.0 showed the second-largest yield. This sample contained the smallest molar fraction of the HPO4 group (). The exfoliation process of ZP-Ph-1.0 is considered to be retarded owing to the minimum content of PO-H groups, which act as ion-exchange sites. ZP-Ph-0 had the lowest SSA and the highest yield among the samples. The exfoliation process of ZP-Ph-0 was considered to proceed slowly compared to that of the other samples. An acetonitrile solution containing CoCl2 was used to treat organically modified LZP samples. The solvent was chosen as it is aprotic and, hence, suppresses ion exchange between the TBA cation and H+. During this treatment, all samples were supplemented with Co2+ in excess of their estimated ion-exchange capacities. After immersion in CoCl2 solution, ZP-Ph-0.5 showed the maximum content of Co2+, accompanied by a decrease in ID. Therefore, it is suggested that the TBA cations at the interlayer of ZP-Ph-0.5 are exchanged with Co2+. ZP-Ph-0.25 contained less Co2+ than ZP-Ph-0.5, even though the yield of dried samples after treatment with TBA was similar. The peaks attributable to the 002 reflections were negligibly small in the XRD patterns of ZP-Ph-0.25. Hence, the disordered ID in ZP-Ph-0.25 leads to a relatively small uptake of Co2+. ZP-Ph-0.75 and ZP-Ph-1.0 showed relatively small uptakes of Co2+, with minimum changes in the IDs among the samples. These phenomena were correlated with the relatively small content of phosphate groups in both samples. shows the schematic structure of ZP-Ph-0.5, based on the crystalline structure of zirconium phenylphosphonate proposed by Poojary et al. [Citation28]. The as-prepared ZP-Ph-0.5 had an ID of approximately 1.5 nm, consistent with the proposed structure. The size of the vacancy between the two phenyl or two phosphate groups was approximately 0.3 and 0.8 nm, respectively ()). After treatment with TBA, the ID increased to 1.8 nm; hence, the distance between the two phosphate groups was estimated to be 1.2 nm. The TBA cation is considered to bind to the phosphate groups because the estimated distance matches the molecular size of a single TBA cation ()). The ID decreased to 1.6 nm after treatment with CoCl2. This decrease in ID was attributed to the exchange of the TBA cation with Co2+ ()). These results demonstrate the possible use of TBA cations as the temporal ID increasing agent with the ability to be easily exchanged with other inorganic cations through a simple immersion process under ambient conditions.
Figure 11. A schematic structure of ZP-Ph-0.5 based on the proposed crystalline structure of zirconium phenylphosphonate by Poojary et al [Citation28]. (a) as prepared sample, (b) samples treated with TBA-solution, and (c) samples treated with TBA-solution followed by treatment with CoCl2 solution
![Figure 11. A schematic structure of ZP-Ph-0.5 based on the proposed crystalline structure of zirconium phenylphosphonate by Poojary et al [Citation28]. (a) as prepared sample, (b) samples treated with TBA-solution, and (c) samples treated with TBA-solution followed by treatment with CoCl2 solution](/cms/asset/ebbe5e78-2814-4251-8e8d-666d78f90a88/tsta_a_1993728_f0011_oc.jpg)
The amounts of Co2+, Cu2+, and Fe3+ introduced into 1 g of ZP-Ph-0.5 were determined to be 0.3, 1.0, and 0.5 mmol·g−1, respectively. These values corresponded to 18, 54, and 41% of the theoretical ion-exchange capacity in ZP-Ph-0.5 (3.7 mmol(+)·g−1). The rapid dissolution of Co2+ and Cu2+ was observed within the initial 2 h, followed by gentle sustained release for up to 672 h. The rates of sustained release were 0.1–0.5 µmol·L−1·h−1 for both ions. Release of Fe3+ from the samples was negligible. Therefore, Fe3+ was found to be fixed more firmly to the organically modified LZP than Co2+ and Cu2+.
Co2+ release behavior from ZP-Ph-0.5 was also evaluated in SBF for investigation in an environment that mimics human body fluids. Sustained release of Co2+ was observed from 2 h to 24 h after immersion. The release rate was 2–37 µmol·L−1·h−1. The release of Co2+ in water was negligible throughout the 24 h experiment. These results suggest that sustained release of Co2+ from organically modified LZPs is facilitated by ion exchange with inorganic ions, including Na+, in saline or SBF.
The particles of drug delivery materials are often embedded into polymer scaffolds and/or coating films for use in vivo. Here, Co2+-incorporated ZP-Ph-0.5 powder was hot-pressed onto one side of the PE substrate, and then immersed in SBF or saline. The mass-to-volume ratio of ZP-Ph-0.5-to-media was identical to that of the immersion test of the powder samples. The Co2+ concentrations in the saline and SBF reached 0.125 and 0.062 mmol·L−1, respectively, after immersion of the substrates for 24 h. These values correspond to approximately one-third of the accumulated concentrations of Co2+ released from the powder sample during the same period.
These concentrations are very close to the effective concentration range of Co2+ for stimulation of angiogenesis by vascular endothelial cells. A previous report showed that Co2+ in the range of 3–12 ppm (0.051–0.204 mmol·L−1) induced the expression of vascular endothelial growth factor in human umbilical vein endothelial cells (HUVECs) cultured on cobalt-containing bioactive glass scaffolds [Citation19]. Cu2+ released from Cu-doped calcium silicate ceramics at a concentration of 0.7 μg·mL−1 (0.011 mmol·L−1) was reported to promote angiogenesis in HUVECs [Citation29]. Here, ZP-Ph-0.5 showed sustained release of Co2+ and Cu2+ at concentrations within the therapeutic range. Therefore, ZP-Ph-0.5 is a potential material for tissue repair utilizing the sustained release of inorganic ions.
5. Conclusion
Layered zirconium phosphate/phosphonate (LZP) with approximately 35 mol% phenyl modification (ZP-Ph-0.5) showed improved incorporation and controlled release of therapeutic inorganic ions. Organically modified derivatives of LZP were successfully prepared with various molar fractions of phenyl groups through reflux processing. When immersed in an acetonitrile solution containing CoCl2, the sample prepared with an equimolar phosphoric acid/phenylphosphonic acid mixture (ZP-Ph-0.5) showed the maximum Co2+ content among the samples. ZP-Ph-0.5 was also capable of being loaded with Cu2+ and Fe3+. The sustained release of Co2+ and Cu2+ was observed up to 672 h after the immersion of ZP-Ph-0.5 in saline solution. The sample also showed controlled release of Co2+ in the simulated body fluid (SBF) for 24 h.
Acknowledgement
A part of this work was conducted at Nagoya University, supported by the “Nanotechnology Platform Program” of the Ministry of Education, Culture, Sports, Science and Technology (MEXT), Japan, Grant Number [JPMXP09F20NU0081].
Disclosure statement
No potential conflict of interest was reported by the author(s).
Additional information
Funding
References
- Cao W, Hench LL. Bioactive materials. Ceram Int. 1996;22:493–507.
- Xynos ID, Edgar AJ, Buttery LD, et al. Ionic products of bioactive glass dissolution increase proliferation of human osteoblasts and induce insulin-like growth factor II mRNA expression and protein synthesis. Biochem Biophys Res Commun. 2000;276:461–465.
- Cho YH, Lee SJ, Lee JY, et al. Antibacterial effect of intraprostatic zinc injection in a rat model of chronic bacterial prostatitis. Int J Antimicrob Agents. 2002;19:576–582.
- Zhang F, Yang X, Zhuang C, et al. Design and evaluation of multifunctional antibacterial ion-doped β-dicalcium silicate cements favorable for root canal sealing. RSC Adv. 2016;6:19707–19715.
- Hench LL. Biomaterials: a forecast for the future. Biomaterials. 1998;19:1419–1423.
- Aguzzi C, Cerezo P, Viseras C, et al. Use of clays as drug delivery systems: possibilities and limitations. Appl Clay Sci. 2007;36:22–36.
- Yang JH, Lee JH, Ryu HJ, et al. Drug–clay nanohybrids as sustained delivery systems. Appl Clay Sci. 2016;130:20–32.
- Choy JH, Jung JS, Oh JM, et al. Layered double hydroxide as an efficient drug reservoir for folate derivatives. Biomaterials. 2004;25:3059–3064.
- Choi G, Kwon OJ, Oh Y, et al. Inorganic nanovehicle targets tumor in an orthotopic breast cancer model. Sci Rep. 2014;4:4430.
- Díaz A, González ML, Pérez RJ, et al. Direct intercalation of cisplatin into zirconium phosphate nanoplatelets for potential cancer nanotherapy. Nanoscale. 2013;5:11456–11463.
- Díaz A, Saxena V, González J, et al. Zirconium phosphate nano-platelets: a novel platform for drug delivery in cancer therapy. Chem Commun. 2012;48:1754–1756.
- Nakamura J, Endo K, Sugawara-Narutaki A, et al. Human stem cell response to layered zirconium phosphate. RSC Adv. 2020;10:36051–36057.
- Lanari D, Montanari F, Marmottini F, et al. New zirconium hydrogen phosphate alkyl and/or aryl phosphonates with high surface area as heterogeneous brønsted acid catalysts for aza-Diels–Alder reaction in aqueous medium. J Catal. 2011;277:80–87.
- Zhang F, Xie Y, Lu W, et al. Preparation of microspherical α-zirconium phosphate catalysts for conversion of fatty acid methyl esters to monoethanolamides. J Colloid Interface Sci. 2010;349:571–577.
- Silbernagel R, Martin CH, Clearfield A. Zirconium(IV) phosphonate–phosphates as efficient ion-exchange materials. lnorg Chem. 2016;55:1651–1656.
- Terban MW, Shi C, Silbernagel R, et al. Local environment of terbium(III) ions in layered nanocrystalline zirconium(IV) phosphonate–phosphate ion exchange materials. Inorg Chem. 2017;56:8837–8846.
- Liu XH, Kirschenbaum A, Yao S, et al. Upregulation of vascular endothelial growth factor by cobalt chloride-simulated hypoxia is mediated by persistent induction of cyclooxygenase-2 in a metastatic human prostate cancer cell line. Clin Exp Metastasis. 1999;17:687–694.
- Wu C, Zhou Y, Fan W, et al. Hypoxia-mimicking mesoporous bioactive glass scaffolds with controllable cobalt ion release for bone tissue engineering. Biomaterials. 2012;33:2076–2085.
- Quinlan E, Partap S, Azevedo MM, et al. Hypoxia-mimicking bioactive glass/collagen glycosaminoglycan composite scaffolds to enhance angiogenesis and bone repair. Biomaterials 2015;52:358–366.
- Menendez A, Barcena M, Jaimez E, et al. Intercalation of n-alkylamines by gamma-titanium phosphate. Synthesis of new materials by thermal treatment of the intercalation compounds. Chem Mater. 1993;5:1078–1084.
- Kim HN, Keller SW, Mallouk TE, et al. Characterization of zirconium phosphate/polycation thin films grown by sequential adsorption reactions. Chem Mater. 1997;9:1414–1421.
- Alberti G, Costantino U, Dionigi C, et al. Layered and pillared zirconium phosphate-phosphonates and their inclusion chemistry. Supramol Chem. 1995;6:29–40.
- Ruiz VSO, Airoldi C. Thermochemical data for n-alkylmonoamine intercalation into crystalline lamellar zirconium phenylphosphonate. Thermochim Acta. 2004;420:73–78.
- Alberti G, Costantino U. Recent progress in the intercalation chemistry of layered α-zirconium phosphate and its derivatives, and future perspectives for their use in catalysis. J Mol Catal. 1984;27:235–250.
- Kullberg LH, Clearfield A. The ion exchange selectivity behavior of zirconium sulphophosphonates towards alkali and alkaline earth cations. Solvent Extr Ion Exch. 1989;7:527–540.
- Yang CY, Clearfield A. The preparation and ion-exchange properties of zirconium sulphophosphonates. React Polym Ion Exch Sorbents. 1987;5:13–21.
- Alberti G, Costantino U, Allulli S, et al. Crystalline Zr(R-PO3)2 and Zr(R-OPO3)2 compounds (R = organic radical): a new class of materials having layered structure of the zirconium phosphate type. J lnorg Nucl Chem. 1978;40:1113–1117.
- Poojary MD, Hu HL, Campbell III FL, et al. Determination of crystal structures from limited powder data sets: crystal structure of zirconium phenylphosphonate. Acta Crystallogr Sect B. 1993;49:996–1001.
- Kong N, Lin K, Li H, et al. Synergy effects of copper and silicon ions on stimulation of vascularization by copper-doped calcium silicate. J Mater Chem B. 2014;2:1100–1110.