ABSTRACT
A poly(3,4-ethylenedioxythiophene):poly(styrenesulfonate) (PEDOT:PSS)-based conducting polymer, which has biorecognition capabilities, has promising biosensing applications. Previously, we developed a facile method for post-printing chemical modification of PEDOT:PSS thin films from commercial sources. Molecular recognition elements were directly introduced into the PSS side chain by a two-step chemical reaction: introduction of an ethylenediamine linker via an acid chloride reaction of the sulfonate moiety, and subsequent receptor attachment to the linker via amine coupling. In this study, the same method was used to introduce 6-carboxypyridine-3-boronic acid (carboxy-PyBA) into the linker for specifically detecting N-acetylneuraminic acid (sialic acid, SA), as a cancer biomarker. The surface-modified PEDOT:PSS films were characterized by X-ray photoelectron spectroscopy, attenuated total reflection Fourier-transform infrared spectroscopy, and static water contact angle and conductivity measurements. The specific interaction between PyBA and SA was detected by label-free reagent-free potentiometry. The SA-specific negative potential responses of modified PEDOT:PSS electrodes, which was ascribed to an SA carboxyl anion, were observed in a physiologically relevant SA range (1.6–2.9 mM) at pH 5, in a concentration-dependent manner even in the presence of 10% fetal bovine serum. The sensitivity was −2.9 mV/mM in 1–5 mM SA with a limit of detection of 0.7 mM. The sensing performances were almost equivalent to those of existing graphene-based electrical SA sensors. These results show that our chemical derivatization method for printing PEDOT:PSS thin films will have applications in SA clinical diagnostics.
1. Introduction
Poly(3,4-ethylenedioxythiophene):poly(styrenesulfonate) (PEDOT:PSS) is a thin, flexible, printable material with high conductivity, robustness, processability, and biocompatibility [Citation1–6]. These advantages enable its use in various applications in biomedical engineering, such as wearable sensing [Citation7–25]. Medical sensors are mainly classified into three types based on their measurement type: physical, chemical, and biosensors. Physical sensors include electrocardiographs, electromyographs, and electroencephalographs [Citation26–28]. Chemical sensors detect volatile organic compounds, biogases, physiological ions, and metabolites [Citation29–31]. A limited number of biosensor applications that use PEDOT:PSS have been reported. This is because of the unique requirements of biosensing materials. Target specificity is necessary for biosensing, and therefore biosensors consist of a biorecognition layer and a signal transducer. The biorecognition layer includes target recognition elements such as antibodies, enzymes, and aptamers. However, PEDOT:PSS lacks the ability to achieve specific biorecognition, therefore it is unsuitable for biosensing applications in its original form. Facile methods for the introduction of biorecognition elements into PEDOT:PSS will therefore enable its use in a wide range of biosensing applications.
There are two main methods for endowing PEDOT:PSS with biorecognition capabilities. The first is to blend it with a substance that has biorecognition abilities. For example, glucose oxidase has been blended with PEDOT:PSS for specific glucose biosensing [Citation32]. The advantages of this method are its simplicity and cost efficiency. Blending occasionally enhances conductivity [Citation32,Citation33]. However, without fixation, bioreceptors are subject to being released from the matrix. The biorecognition of a protein is easily lost by denaturation during fabrication and long-term usage [Citation32]. The second method is to introduce a biorecognition site into the side chain of PEDOT or PSS via chemical synthesis of PEDOT:PSS derivatives. Hydroxymethyl-PEDOT (PEDOT-OH) enables covalent immobilization of anti-Escherichia coli by using an epoxysilane [Citation34]. Similarly, the covalent immobilization of glucose oxidase has been achieved by epoxysilane treatment to give a poly(vinyl alcohol)/PEDOT:PSS blend [Citation35]. Our group synthesized a PEDOT derivative that contains a phospholipid polar group in the side chain for detecting the inflammation biomarker C-reactive protein [Citation36]. In addition, α2,6-sialyllactose was conjugated with a PEDOT derivative bearing an oxyamine moiety for specific recognition of human influenza A virus [Citation37]. In this way, various PEDOT analogs can be synthesized for biosensing applications. The conductivities of PEDOT adducts are lower than that of pristine PEDOT [Citation38–40].
We have been investigating new methods for obtaining PEDOT:PSS derivatives that possess bioreceptors. In particular, post-printing chemical modification is attractive in terms of simplicity and cost efficiency. Post-printing modification of PSS by using aromatic diazonium salts has been reported [Citation41], but the complexity of the chemical reaction is an issue. Previously, as a proof of concept, we performed a two-step post-printing modification of PSS [Citation42]. First, an ethylenediamine linker was introduced into the sulfonate group via an acid chloride reaction. Then, 4-carboxy-3-fluorophenylboronic acid (carboxy-FPBA) was introduced into the amine end by an amide condensation reaction. FPBA functioned as a synthetic receptor for diol compounds and enabled label-free glucose sensing. Our post-printing modification has the advantages of simplicity, mass productivity, and cost efficiency. Furthermore, by changing the linker and biorecognition element, it can be used to detect a wide variety of analytes. In this study, to demonstrate the versatility of our developed technique, we introduced a pyridylboronic acid (PyBA) bioreceptor into printed PEDOT:PSS films for specifically detecting N-acetylneuraminic acid, namely sialic acid (SA). SA is recognized as inflammation [Citation43–45] and cancer metastasis [Citation46–49] biomarkers. In recent years, SA has been reported to be related to brain diseases [Citation50,Citation51]. The artificial bioreceptor PyBA specifically interacts with SA under weakly acidic conditions among other monosaccharides including glucose [Citation52]. This interaction enables specific SA detection by using PyBA-functionalized PEDOT:PSS (PEDOT:PSS-PyBA). Compared with natural lectins, synthetic PyBA is stable and robust, and is suitable for biosensing in many biomedical fields.
2. Materials and methods
2.1. Materials
A poly(ethylene terephthalate) (PET) substrate-laminated PEDOT:PSS (200 nm thick) thin film (Orgacon® F350, Agfa-Gebhardt, Tokyo, Japan) was used. Oxalyl chloride was purchased from Tokyo Chemical Industry (Tokyo, Japan). 6-Carboxypyridine-3-boronic acid, 4-(4,6-dimethoxy-1,3,5-triazin-2-yl)-4-methylmorpholinium chloride (DMT-MM), and SA were purchased from Fujifilm Wako Pure Chemicals (Tokyo, Japan). Fetal bovine serum (FBS) was purchased from Funakoshi (Tokyo, Japan). All other reagents were of extra-pure grade, obtained from commercial sources, and used as received. Milli-Q water (18.2 MΩ cm, Merck, Darmstadt, Germany) was used in all experiments.
2.2. Chemical modification of PEDOT:PSS film
The PEDOT:PSS film on PET was modified via a two-step reaction () [Citation53]. The first step was an acid chloride reaction at the sulfonate group in PSS, with oxalyl chloride, and subsequent introduction of ethylenediamine, as previously reported [Citation42]. In the second step, dimethyl sulfoxide (10 mL), sodium hydrogen carbonate (42 mg), and 6-carboxypyridine-3-boronic acid (184 mg, 1.1 mmol) were added to a two-necked flask (50 mL). The ethylenediamine-modified PEDOT:PSS (PEDOT:PSS-diamine) and DMT-MM (304 mg, 1.1 mmol) were placed in the two-necked flask under Ar, and the reaction was performed for 12 h at 50°C. The films were washed with water and dried for 1 h at 25°C. The 6-carboxypyridine-3-boronic acid-modified PEDOT:PSS-diamine film (PEDOT:PSS-PyBA) was stored in the dark at 25°C until use.
Figure 1. In situ post-printing chemical derivatization of PEDOT:PSS layer on PET substrate for label-free biosensing of SA. The sulfonate group in PEDOT:PSS was converted into a sulfonate chloride, with subsequent introduction of carboxy-PyBA via ethylenediamine as a linker. SA was recognized by PyBA-modified PEDOT:PSS. The specific binding of anionic SA generated negative surface potentials in potentiometric measurements.
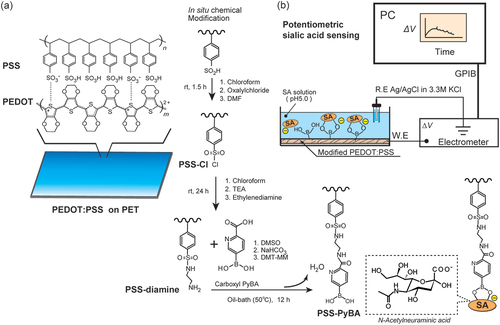
2.3. Characterization
The film surfaces were examined by X-ray photoelectron spectroscopy (XPS; JPS-9010MC spectrometer, JEOL Ltd., Tokyo, Japan). An Mg Kα source (12 kV) and a neutralizer were used. The photoelectron pass energy was 20 eV and the takeoff angle was 90°. Charge correction was based on the C1s peak at 284.2 eV. The mean and standard deviation (SD) were obtained from three measurements.
The surface wettability was evaluated by performing static water contact angle measurements (DM-501 goniometer, Kyowa Interfacial Chemical, Saitama, Japan). The mean and SD were calculated from five measurements.
The surface resistance was measured by the two-terminal method at a 1 mm distance by using a 107 digital multimeter (FLUKE, Everett, WA, USA) with 0.7 mm tip, gold-coated low-resistance probes. The mean and SD were obtained from ten measurements.
An iS50 Fourier-transform infrared (FTIR) spectrometer (Thermo Fisher Scientific Japan, Tokyo, Japan) equipped with a universal attenuated total reflection (ATR) sampling assembly was used to identify the functional groups on the surfaces of the modified PEDOT:PSS films.
2.4. Potentiometry
The modified PEDOT:PSS films (1 cm × 1 cm), which were used as working electrodes, and an Ag/AgCl reference electrode (in 3.3 M KClaq via a salt bridge) were connected to a 6517B multichannel high-resistance electrometer (Keithley Instruments, Cleveland, OH, USA). The working and reference electrodes were immersed in a 50 mM 2-(N-morpholino)ethanesulfonic acid (MES) buffer solution (pH 5.0) (). After conditioning for 2 h, a stock solution of SA was added dropwise at 20 min intervals to increase the SA concentration from 0 to 1, 2, 3, 4, 5, and 10 mM, while potential changes (ΔV) from baseline (stable points just before treating the solution at 0 mM SA) were observed. The interval was set for 20 min at each SA level to minimize the effect of signaling drift over time while assuring the period for the binding equilibrium. The same method was used to examine the potentiometric responses to glucose, galactose, and mannose. The same potentiometric system was used to investigate the pH dependences in buffer solutions at pH 5.0, 6.0, 7.0, and 8.0. MES buffer solutions were used at pH 5.0 and 6.0, and 4-(2-hydroxyethyl)-1-piperazineethanesulfonic acid (HEPES) buffer solutions (10 mM) were used at pH 7.0 and 8.0. A MES buffer containing 10% FBS was used for measurements under realistic conditions. The mean and SD were determined from five measurements.
2.5. Statistical analysis
The data were analyzed by using Student’s t-tests in two groups. In three or more groups, the data were analyzed by analysis of variance (one-way), and multiple comparisons were made by using Tukey’s test; p < 0.05 was considered to be statistically significant.
3. Results and discussion
3.1. Confirmation of surface modification of PEDOT:PSS films
Surface modification of the PEDOT:PSS film in each synthetic step was evaluated by using XPS to perform elemental analysis. The N1s peaks at 398 eV for PEDOT:PSS-diamine and PEDOT:PSS-PyBA were clearly stronger than that for pristine PEDOT:PSS (). These results indicate that the acid chloride reaction introduced ethylenediamine into the PSS side chain. However, the nitrogen in the pyridine ring was indistinguishable from that in ethylenediamine. Alternatively, PyBA introduction was confirmed by the presence of the B1s peak at 190 eV (). Successful chemical modification of PEDOT:PSS-diamine with carboxy-PyBA via amine coupling condensation was therefore confirmed.
Figure 2. High-resolution XPS data of pristine PEDOT:PSS, PEDOT:PSS-diamine, and PEDOT:PSS-PyBA. (a) N1s peaks in PEDOT:PSS-diamine and PEDOT:PSS-PyBA spectra indicate ethylenediamine or pyridine moieties. (b) distinct B1s peak in PEDOT:PSS-PyBA spectrum indicates covalent introduction of PyBA on the film.
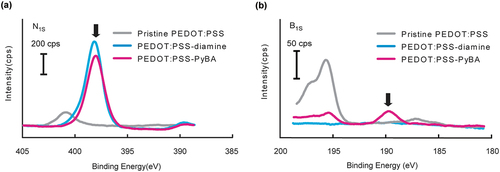
ATR-FTIR spectroscopic analysis also confirmed two-step surface modification (Figure S1). The peaks at 1660 and 3300 cm−1, from C(=O)–NH and NH, respectively, indicated the presence of ethylenediamine in PEDOT:PSS-diamine and PEDOT:PSS-PyBA. The peaks at 1700 and 870 cm−1, from – C=O and aromatic disubstituents, respectively, were exclusively observed for PEDOT:PSS-PyBA. The peak at 720 cm−1, from the benzene ring, was observed for all the samples. The changes in the spectrum of pristine PEDOT:PSS were the same as those previously reported [Citation54,Citation55].
The static water droplet contact angles and surface resistance were measured before and after chemical modifications (). The contact angles for PEDOT:PSS-diamine and PEDOT:PSS-PyBA were higher than that for pristine PEDOT:PSS. The decreased hydrophilicity indicates that the anionic sulfonate groups were replaced by neutral ethylenediamine and PyBA by chemical modification at pH 7.4. The surface resistances of PEDOT:PSS-diamine and PEDOT:PSS-PyBA were 6.9 and 4.6 times higher, respectively, than that of the pristine PEDOT:PSS film as a result of the chemical modifications. Low-impedance surfaces are important for sensitive electrochemical biosensing. These values are comparable to those previously reported for successful potentiometric biosensing of glucose by using chemically modified PDOT:PSS with a surface resistance of 103 Ω/□ [Citation42]. We confirmed that a film with a surface resistance of the order of 104 Ω/□ gave no potentiometric response.
Table 1. Changes in static water contact angle and sheet resistance during surface modification. Water contact angle: mean ± SD (n = 5); sheet resistance: mean ± SD (n = 10).
The surface morphology of PEDOT:PSS before and after chemical modifications were observed by scanning electron microscopy (SEM). A smooth surface was observed on the pristine PEDOT:PSS. On the other hand, rough surfaces were observed on PEDOT:PSS-diamine and PEDOT:PSS-PyBA (Figure S2). These topographical changes may have influenced on the wettability and sensitivity.
3.2. pH-Dependent SA binding to PEDOT:PSS-PyBA
On the basis of the surface characterization results, we used PEDOT:PSS-PyBA for label-free SA biosensing. Under weakly acidic conditions, the non-dissociated form of PyBA has specificity for SA over other diols, including monosaccharides [Citation56,Citation57]. The pH dependence of SA recognition was investigated by performing potentiometric measurements (). The potential changed significantly (p < 0.01) in the negative direction with increasing SA concentration at pH 5.0; this trend was not observed at higher pH conditions. The negative potential change indicated binding of negatively charged SA to the non-dissociated form of PyBA in the PEDOT:PSS side chain. The potential changes for 1–10 mM glucose, galactose, and mannose were negligible at pH 5.0 ( and S3a). These results are consistent with previous reports that these monosaccharides cannot bind to non-dissociated PyBA under weakly acidic conditions, that is, below the pKa of PyBA [Citation58–60]. At pH 7.0, the PyBA moiety started to bind to these monosaccharides (Figure S3b). This shows that PEDOT:PSS-PyBA lost its specificity for SA at neutral pH. The negative potential change that followed the binding of electrically neutral monosaccharides is attributed to dissociation of PyBA.
Figure 3. pH-Dependent selective binding of SA with PyBA on PEDOT:PSS-PyBA. (a) potential changes of PEDOT:PSS-PyBA electrodes at varying concentrations of SA from 1 to 10 mM at pH 5.0–8.0 at 25°C; mean ± SD (n = 5). (b) potential changes of PEDOT:PSS-PyBA electrodes in 5 mM SA, glucose, galactose, and mannose at pH 5.0–8.0 at 25°C. Mean ± SD (n = 5), **p < 0.01.
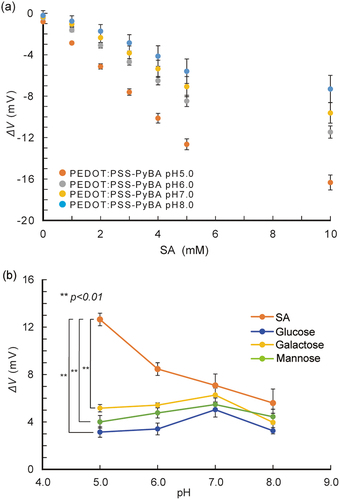
We then performed real-time label-free SA biosensing. PEDOT:PSS-PyBA rapidly responded to changes in the SA solution concentration, and increasingly negative potential signals were observed (). The signal was approximately −12.5 mV at 10 mM SA. In contrast, PEDOT:PSS-diamine, which lacks PyBA as a receptor for SA, gave modest shifts, up to −4 mV at 10 mM SA (Figure S4). These small shifts are considered to be non-specific signals. Statistically significant differences (p < 0.05) were observed between PEDOT:PSS-PyBA and PEDOT:PSS-diamine at SA concentration ≥1 mM (). PEDOT:PSS-PyBA showed a linear response of −1.9 mV/mM at 1–5 mM SA. The 1:1 Langmuir model was used to analyze the differential signals of the two samples. The apparent dissociation constant (Kd) was 12.1 mM (Figure S5), an order of magnitude inferior to that obtained in the solution phase [Citation60]. The decreased binding ability at the solid/liquid interface can be attributed to steric hindrance and electrostatic repulsion by neighboring SA molecules that already adsorbed on the PyBA receptor. The lower limit of detection of PEDOT:PSS-PyBA was determined to be 0.4 mM, based on three times the standard deviations from the baseline.
Figure 4. Potentiometric detection of SA using PyBA-functionalized PEDOT:PSS electrodes at pH 5.0 at 25°C. (a) time course of potential changes during sequential increases in SA concentration from 0 to 10 mM. (b) ΔV as function of SA concentration for PEDOT:PSS-PyBA and PEDOT:PSS-diamine. Mean ± SD (n = 5). Statistically significant differences (*p < 0.05) were observed at ≥1 mM SA.
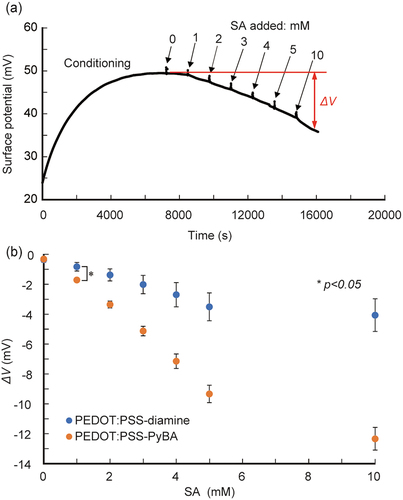
3.3. SA biosensing in realistic samples
We performed SA detection in a solution with 10% FBS to confirm the biosensing ability in realistic samples containing interfering substances (e.g. serum proteins) that cause non-specific adsorptions. The same trend was observed; with increasing SA level from 1 to 10 mM in 10% FBS solution, the signals generated by PEDOT:PSS-PyBA were significantly more negative than those generated by PEDOT:PSS-diamine ( and S6). The sensitivity of PEDOT:PSS-PyBA was −2.9 mV/mM at 1–5 mM SA. The lower limit of detection was 0.7 mM in 10% FBS. The dynamic range covered clinically relevant free SA levels (1.6–2.9 mM) in human serum [Citation56]. However, with PEDOT:PSS-diamine, the undesired response in 10% FBS was higher than those in buffer solutions. We infer that non-specific adsorption of serum proteins occurred on PEDOT:PSS-diamine, which caused non-specific interactions between the adsorbed layer and SA [Citation61]. It may be necessary to introduce non-fouling molecules such as poly(ethylene glycol) and zwitterions on the surface of the conducting layer [Citation62] to suppress non-specific adsorption of serum proteins.
Figure 5. Potentiometric detection of SA using PyBA-functionalized PEDOT:PSS electrodes in 10% FBS at pH 5.0 at 25°C. (a) time course of potential changes during sequential increases in SA concentration. (b) ΔV as function of SA concentration for PEDOT:PSS-PyBA and PEDOT:PSS-diamine. Mean ± SD (n = 5). Statistically significant differences (*p < 0.05) were observed at ≥1 mM SA.
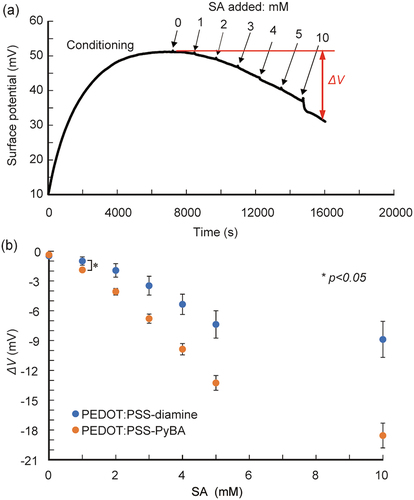
The sensing performance of PEDOT:PSS-PyBA was compared with those of other potentiometric-type label-free SA biosensors (). The biosensors listed all cover clinically relevant free SA levels (1.6–2.9 mM) in human serum [Citation56]. The dynamic range of the combined molecular imprinting/carbon nanotube sensor is wider than that of our biosensor, but the sensitivity is comparable. SA biosensors that combine this molecular imprinting technology with carbon nanotubes are expensive, and have a long fabrication time. In contrast, among other biosensors, our biosensor has the advantages of cost efficiency and simplicity, while giving similar sensing performances. These features may enable the development of SA biosensors in wearable formats. Choosing an appropriate combination of a linker and bioreceptor will enable the method described here to be used in a wide range of biosensors with various capture methods.
Table 2. Comparison of developed biosensor with potentiometric SA biosensors reported in the literature.
4. Conclusions
We extended our previously developed method to the chemical modification of PSS side chains with biorecognition sites in a printed PEDOT:PSS layer. PyBA was chemically anchored on the conducting surface, for the first time, via ethylenediamine for specifically detecting SA as a cancer biomarker under clinically relevant conditions. Our results prove the feasibility of introducing a variety of biorecognition elements into large areas of commercial PEDOT:PSS films. These chemically derivatized conducting polymer films have the potential to be commonly used materials in versatile electrochemical biosensing applications. The in situ surface modification of flexible PEDOT:PSS thin films could be used to develop wearable devices that could contribute to establishing digital healthcare systems in the future.
Supplemental Material
Download MS Word (16.8 MB)Acknowledgements
The authors thank Prof. T. Hanawa at Tokyo Medical and Dental University (TMDU) for offering the use of an XPS instrument, Prof. N. Yui at TMDU for offering the use of a contact angle goniometer, and Prof. A. Kishida at TMDU for offering the use of a FTIR instrument.
Disclosure statement
No potential conflict of interest was reported by the author(s).
Supplementary material
Supplemental data for this article can be accessed online at https://doi.org/10.1080/14686996.2022.2122867
Correction Statement
This article has been corrected with minor changes. These changes do not impact the academic content of the article.
Additional information
Funding
References
- Won Y, Lee JJ, Shin J, et al. Biocompatible, transparent, and high-areal-coverage kirigami PEDOT:PSS electrodes for electrooculography-derived human-machine interactions. ACS Sens. 2021;6(3):967–975. DOI:10.1021/acssensors.0c02154
- Joseph Adekoya G, Emmanuel Sadiku R, Sinha Ray S. Nanocomposites of PEDOT:PSS with graphene and its derivatives for flexible electronic applications: a review. Macromol Mater Eng. 2021;306(3):2000716. doi:10.1002/mame.202000716
- Fenoy GE, Azzaroni O, Knoll W, et al. Functionalization strategies of PEDOT and PEDOT:PSS films for organic bioelectronics applications. Chemosensors. 2021;9(8):212. DOI:10.3390/chemosensors9080212
- Han JW, Wibowo AF, Park J, et al. Highly stretchable, robust, and conductive lab-synthesized PEDOT:PSS conductive polymer/hydroxyethyl cellulose films for on-skin health-monitoring devices. Org Electron. 2022;105:106499. doi:10.1016/j.orgel.2022.106499
- Wang Z, Wang M, Jiao B, et al. Smooth and mechanically robust random metallic mesh electrode modified by thermally transferred PEDOT: PSS for ITO-free flexible organic light-emitting diodes. Org Electron. 2022;106:106498. doi:10.1016/j.orgel.2022.106498
- Jo YJ, Kim SY, Hyun JH, et al. Fibrillary gelation and dedoping of PEDOT:PSS fibers for interdigitated organic electrochemical transistors and circuits. npj Flex Electron. 2022;6(31):1–11. doi:10.1038/s41528-022-00167-7
- Tan Z, Li H, Huang Y, et al. Breathing-effect assisted transferring large-area PEDOT:PSS to PDMS substrate with robust adhesion for stable flexible pressure sensor. Compos Part a Appl Sci Manuf. 2021;143:106299. doi:10.1016/j.compositesa.2021.106299
- Liu G, Chen X, Liu J, et al. Fabrication of PEDOT:PSS/rGO fibers with high flexibility and electrochemical performance for supercapacitors. Electrochim Acta. 2021;365:137363. doi:10.1016/j.electacta.2020.137363
- Zhao X, Li K, Fang Y, et al. Vertically stacked PEDOT:PSS/PbS/CsPbCl3 for flexible optoelectronic devices. J Alloys Compd. 2021;866:158997. doi:10.1016/j.jallcom.2021.158997
- Yang J, Jia Y, Liu Y, et al. PEDOT:PSS/PVA/Te ternary composite fibers toward flexible thermoelectric generator. Compos Commun. 2021;27:100855. doi:10.1016/j.coco.2021.100855
- Xu S, Fan Z, Yang S, et al. Highly flexible, stretchable, and self-powered strain-temperature dual sensor based on free-standing PEDOT:PSS/Carbon Nanocoils-Poly(vinyl) alcohol films. ACS Sens. 2021;6(3):1120–1128. DOI:10.1021/acssensors.0c02390
- Kuzubasoglu BA, Sayar E, Bahadir SK. Inkjet-printed CNT/PEDOT:PSS temperature sensor on a textile substrate for wearable intelligent systems. IEEE Sens J. 2021;21(12):13090–13097. doi:10.1109/JSEN.2021.3070073
- Gao N, Yu J, Tian Q, et al. Application of PEDOT:PSS and its composites in electrochemical and electronic chemosensors. Chemosensors. 2021;9(4):79–104. DOI:10.3390/chemosensors9040079
- Lam TN, Lee GS, Kim B, et al. Microfluidic preparation of highly stretchable natural rubber microfiber containing CNT/PEDOT:PSS hybrid for fabric-sewable wearable strain sensor. Compos Sci Technol. 2021;210:108811. doi:10.1016/j.compscitech.2021.108811
- Lo L-W, Zhao J, Wan H, et al. An inkjet-printed PEDOT:PSS-based stretchable conductor for wearable health monitoring device applications. ACS Appl Mater Interfaces. 2021;13(18):21693–21702. DOI:10.1021/acsami.1c00537
- Sun F, Huang X, Wang X, et al. Highly transparent, adhesive, stretchable and conductive PEDOT:PSS/polyacrylamide hydrogels for flexible strain sensors. Colloids Surf a Physicochem Eng Asp. 2021;625:126897. doi:10.1016/j.colsurfa.2021.126897
- Wang Y, Wang M, Wang P, et al. Urea-treated wet-spun PEDOT: PSS fibers for achieving high-performance wearable supercapacitors. Compos Commun. 2021;27:100885. doi:10.1016/j.coco.2021.100885
- Wang P, Wang M, Zhu J, et al. Surface engineering via self-assembly on PEDOT: PSS fibers: biomimetic fluff-like morphology and sensing application. Chem Eng Sci. 2021;425:131551. doi:10.1016/j.cej.2021.131551
- Liu H, Zhang S, Li Z, et al. Harnessing the wide-range strain sensitivity of bilayered PEDOT:PSS films for wearable health monitoring. Matter. 2021;4(9):2886–2901. DOI:10.1016/j.matt.2021.06.034
- Pattanarat K, Petchsang N, Osotchan T, et al. Wash-durable conductive yarn with ethylene glycol-treated PEDOT:PSS for wearable electric heaters. ACS Appl Mater Interfaces. 2021;13(40):48053–48060. DOI:10.1021/acsami.1c13329
- Xu C, Jiang D, Ge Y, et al. A PEDOT:PSS conductive hydrogel incorporated with Prussian blue nanoparticles for wearable and noninvasive monitoring of glucose. Chem Eng Sci. 2022;431:134109. doi:10.1016/j.cej.2021.134109
- Lee JH, Raman V, Kang C, et al. Highly stretchable transparent bar coated Ag NW/PEDOT:PSS hybrid electrode for wearable and stretchable devices. RSC Adv. 2022;12(5):3055–3061. DOI:10.1039/d1ra08173j
- Jo HS, Park CW, An S, et al. Wearable multifunctional soft sensor and contactless 3D scanner using supersonically sprayed silver nanowires, carbon nanotubes, zinc oxide, and PEDOT:PSS. NPG Asia Mater. 2022;14(1):1–23. doi:10.1038/s41427-022-00370-y
- Wang Y, Zhu J, Shen M, et al. Three-layer core–shell Ag/AgCl/PEDOT: PSS composite fibers via a one-step single-nozzle technique enabled skin-inspired tactile sensors. Chem Eng Sci. 2022;442:136270. doi:10.1016/j.cej.2022.136270
- Shiu BC, Liu YL, Yuan QY, et al. Preparation and characterization of PEDOT:PSS/TiO2 micro/nanofiber-based gas sensors. Polymers. 2022;14(9):1780. DOI:10.3390/polym14091780
- Lam E, Alizadeh-Meghrazi M, Schlums A, et al. Exploring textile-based electrode materials for electromyography smart garments. J Rehabil Assist Technol Eng. 2022;9:20556683211061995. doi:10.1177/20556683211061995
- Maithani Y, Singh A, Mehta BR, et al. PEDOT: PSS treated cotton-based textile dry electrode for ECG sensing. Mate Today: Proc. 2022;62:4052–4057. DOI: 10.1016/j.matpr.2022.04.611
- Hsieh JC, Li Y, Wang H, et al. Design of hydrogel-based wearable EEG electrodes for medical applications. J Mater Chem B. 2022. DOI:10.1039/d2tb00618a
- Xu Z, Song J, Liu B, et al. A conducting polymer PEDOT:PSS hydrogel based wearable sensor for accurate uric acid detection in human sweat. Sens Actuators B Chem. 2021;348:130674. doi:10.1016/j.snb.2021.130674
- Zhu C, Xu Y, Chen Q, et al. A flexible electrochemical biosensor based on functionalized poly(3,4-ethylenedioxythiophene) film to detect lactate in sweat of the human body. J Colloid Interface Sci. 2022;617:454–462. doi:10.1016/j.jcis.2022.03.029
- Fujita H, Hao M, Takeoka S, et al. Paper‐based wearable ammonia gas sensor using organic–inorganic composite PEDOT:PSS with Iron(III) compounds. Adv Mater Technol. 2022:2101486. DOI: 10.1002/admt.202101486
- Abd-Wahab F, Guthoos HFA, Salim WWAW. Solid-state rGO-PEDOT:PSS transducing material for cost-effective enzymatic sensing. Biosensors (Basel). 2019;9(1):36. doi:10.3390/bios9010036
- Strakosas X, Wei B, Martin DC, et al. Biofunctionalization of polydioxythiophene derivatives for biomedical applications. J Mater Chem B. 2016;4(29):4952–4968. DOI:10.1039/c6tb00852f
- He RX, Zhang M, Tan F, et al. Detection of bacteria with organic electrochemical transistors. J Mater Chem A. 2012;22(41):22072–22076. DOI:10.1039/c2jm33667g
- Strakosas X, Sessolo M, Hama A, et al. A facile biofunctionalisation route for solution processable conducting polymer devices. J Mater Chem B. 2014;2(17):2537–2545. DOI:10.1039/c3tb21491e
- Goda T, Toya M, Matsumoto A, et al. Poly(3,4-ethylenedioxythiophene) bearing phosphorylcholine groups for metal-free, antibody-free, and low-impedance biosensors specific for C-reactive protein. ACS Appl Mater Interfaces. 2015;7(49):27440–27448. DOI:10.1021/acsami.5b09325
- Hai W, Goda T, Takeuchi H, et al. Specific recognition of human influenza virus with PEDOT bearing sialic acid-terminated trisaccharides. ACS Appl Mater Interfaces. 2017;9(16):14162–14170. DOI:10.1021/acsami.7b02523
- Mochizuki Y, Horii T, Okuzaki H. Effect of pH on structure and conductivity of PEDOT/PSS. Trans Mat Res Soc Jpn. 2012;37(2):307–310. doi:10.14723/TMRSJ.37.307
- Paulraj I, Liang TF, Yang TS, et al. Enhanced power factor of PEDOT:PSS films post-treated using a combination of ethylene glycol and metal chlorides and temperature dependence of electronic transport (325–450 K). ACS Appl Energy Mater. 2020;3(12):12447–12459. DOI:10.1021/acsaem.0c02411
- Yang J, Chang L, Ma C, et al. Highly electrically conductive flexible Ionogels by drop-casting Ionic liquid/PEDOT:PSS composite liquids onto hydrogel networks. Macromol Rapid Commun. 2022;43(1):e2100557. DOI:10.1002/marc.202100557
- Guselnikova GO, Postnikov PS, Fitl P, et al. Tuning of PEDOT:PSS properties through covalent surface modification. J Polym Sci A Polym Chem. 2017;55(4):378–387. DOI:10.1002/polb.24282
- Fujisaki H, Watcharawittayakul T, Matsumoto A, et al. In-situ chemical modification of printed conducting polymer films for specific glucose biosensing. Sens Actuators B Chem. 2021;349:130829. doi:10.1016/j.snb.2021.130829
- Nigam PK, Narain VS, Kumar A. Sialic acid in cardiovascular diseases. Indian J Clin Biochem. 2006;21(1):54–61. doi:10.1007/BF02913067
- D’souza JMP, Pai VR, Harish S, et al. Relation of serum and salivary total sialic acid and total sialic acid/total protein ratio with clinical parameters of disease progression in preeclampsia. Indian J Clin Biochem. 2022;37(1):113–118. DOI:10.1007/s12291-021-00956-3
- Bordron A, Morel M, Bagacean C, et al. Hyposialylation must be considered to develop future therapies in autoimmune diseases. Int J Mol Sci. 2021;22(7):3402–3415. DOI:10.3390/ijms22073402
- Joshi M, Patil R. Estimation and comparative study of serum total sialic acid levels as tumor markers in oral cancer and precancer. J Cancer Res Ther. 2010;6(3):263–266. doi:10.4103/0973-1482.73339
- Dobie C, Skropeta D. Insights into the role of sialylation in cancer progression and metastasis. Br J Cancer. 2021;124(1):76–90. doi:10.1038/s41416-020-01126-7
- Visser EA, Moons SJ, Timmermans SBPE, et al. Sialic acid O-acetylation: from biosynthesis to roles in health and disease. J Biol Chem. 2021;297(2):100906. DOI:10.1016/j.jbc.2021.100906
- Miyazaki T, Khan T, Tachihara Y, et al. Boronic acid ligands can target multiple subpopulations of pancreatic cancer stem cells via pH-dependent glycan-terminal sialic acid recognition. ACS Appl Bio Mater. 2021;4(9):6647–6651. DOI:10.1021/acsabm.1c00383
- Yang X, Liang S, Wang L, et al. Sialic acid and anti-ganglioside antibody levels in children with autism spectrum disorders. Brain Res. 2018;1678:273–277. doi:10.1016/j.brainres.2017.10.027
- Yadava J, Verma AK, Gargb RK, et al. Sialic acid associated with oxidative stress and total antioxidant capacity (TAC) expression level as a predictive indicator in moderate to severe alzheimer’s disease. Exp Gerontol. 2020;141:111092. doi:10.1016/j.exger.2020.111092
- Matsumoto A, Miyahara Y. ‘Borono-lectin’ based engineering as a versatile platform for biomedical applications. Sci Technol Adv Mater. 2018;19(1):18–30. doi:10.1080/14686996.2017.1411143
- Li J, Zhu H, Wang X, et al. Synthesis of sodium poly[4-styrenesulfonyl(trifluoromethylsulfonyl)imide]-co-ethylacrylate] solid polymer electrolytes. Electrochimica Acta. 2015;175:232–239. doi:10.1016/j.electacta.2015.03.075
- Tehrani P, Kanciurzewska A, Crispin X, et al. The effect of pH on the electrochemical over-oxidation in PEDOT:PSS films. Solid State Ion. 2007;177(39–40):3521–3527. DOI:10.1016/j.ssi.2006.10.008
- Park JK, Jang C, Yun GH, et al. Sensitive relative humidity monitoring sensor based on microwave active resonator with PEDOT:PSS. IEEE Access. 2020;8:166283–166293. doi:10.1109/access.2020.3022389
- Hai W, Pu S, Wang X, et al. Poly(3,4-ethylenedioxythiophene) bearing pyridylboronic acid group for specific recognition of sialic acid. Langmuir. 2020;36(2):546–553. DOI:10.1021/acs.langmuir.9b03442
- Bao W, Hai W, Bao L, et al. Poly(3,4-ethylenedioxythiophene) bearing fluoro-containing phenylboronic acid for specific recognition of glucose. Mater Chem Front. 2021;5(20):7675–7683. DOI:10.1039/d1qm00926e
- Otsuka H, Uchimura E, Koshino H, et al. Anomalous binding profile of phenylboronic acid with N -Acetylneuraminic acid (Neu5ac) in aqueous solution with varying pH. J Am Chem Soc. 2003;125(12):3493–3502. DOI:10.1021/ja021303r
- Matsumoto A, Osawa S, Arai T, et al. Potentiometric determination of circulating glycoproteins by boronic acid end-functionalized Poly(ethylene glycol)-modified electrode. Bioconjug Chem. 2021;32(2):239–244. DOI:10.1021/acs.bioconjchem.0c00657
- Matsumoto A, Stephenson-Brown AJ, Khan T, et al. Heterocyclic boronic acids display sialic acid selective binding in a hypoxic tumor relevant acidic environment. Chem Sci. 2017;8(9):6165–6170. DOI:10.1039/c7sc01905j
- Broncová G, Matějka P, Němečková Z, et al. Electrochemical detection of sialic acid using phenylboronic acid-modified poly(diaminobenzoic acid) electrodes. Electroanalysis. 2018;30(4):672–680. DOI:10.1002/elan.201700634
- Goda T, Miyahara Y. Electrodeposition of zwitterionic PEDOT films for conducting and antifouling surfaces. Langmuir. 2019;35(5):1126–1133. doi:10.1021/acs.langmuir.8b01492
- Zhou Y, Dong H, Liu L, et al. A novel potentiometric sensor based on a poly(anilineboronic acid)/graphene modified electrode for probing sialic acid through boronic acid-diol recognition. Biosens Bioelectron. 2014;60:231–236. doi:10.1016/j.bios.2014.04.012
- Shishkanova TV, Broncová G, Němečková Z, et al. Molecular frameworks of polymerized 3‑aminobenzoic acid for chemical modification and electrochemical recognition. J Electroanal Chem. 2019;832:321–328. doi:10.1016/j.jelechem.2018.11.011
- Huang F, Zhu B, Zhang H, et al. A glassy carbon electrode modified with molecularly imprinted poly(aniline boronic acid) coated onto carbon nanotubes for potentiometric sensing of sialic acid. Mikrochim Acta. 2019;186(5):270–280. DOI:10.1007/s00604-019-3387-8
- Zhou Y, Huangfu H, Yang J, et al. Potentiometric analysis of sialic acid with a flexible carbon cloth based on boronate affinity and molecularly imprinted polymers. Analyst. 2019;144(21):6432–6437. DOI:10.1039/c9an01600g