1. Current status
Gene therapy is currently in the front rows of biomedical research with an increased focus on direct nuclease-based editing. If until now the research was mainly concentrated on modifications at the DNA level, new adapted tolls are starting to gain momentum due to their ability to induce changes within the RNA sequence. The advantage consists in the half-life of the messenger RNA and implicit the non-permanent character of the editing strategy. Recently, the ability of nucleases like Cas12a and Cas13 to cleave DNA and RNA, respectively, was used for detection of low particle infectious agents with high sensitivity – e.g. DETECTOR and SHERLOCK Systems. The present article briefly presents the latest advancements from the gene editing therapeutic area, as well as the new diagnostic tools with great likelihoods within the clinical environment.
2. Latest advancements in editing
The last decade of biomedical research witnessed an unprecedented increase in the number of advances on non-coding RNAs [Citation1,Citation2] and non-coding DNAs [Citation3]. Year 2017 has been considered the Year of Gene-Therapy Breakthroughs according to the researchers from MIT [Citation4]. The leeway of specifically changing the DNA or RNA sequence in order to correct the causative-specific dysregulation converging to a pathological state has the potential to reshape the future medicine [Citation4]. Programmable nucleases for genome editing like transcription activator-like effector nucleases (TALENs), meganucleases, zinc-finger nucleases (ZFNs) and clustered regularly interspaced short palindromic repeats (CRISPR)-Cas are now in the spotlight of experimental research () [Citation5–Citation8]. Based on these achievements several gene-therapy based treatments get contour in the last couple of years. In March 2017, a young male patient entered into complete clinical remission for sickle cell disease after receiving ex vivo gene therapy by a lentiviral vector [Citation9]; Kymriah (a chimeric antigen receptor CAR-T therapy for non-Hodgkin lymphoma and B-cell acute lymphoblastic leukemia) and Yescarta (a CD19-directed genetically modified autologous T cell immunotherapy), two types of autologous CAR-T cell therapies designed for cancer patients unresponsive to the standard of care received FDA approval in 2017 [Citation10]; in November 2017 a 7-year-old child with junctional epidermolysis bullosa (JEB) had his skin layer replaced by cultured self-stem cells genetically engineered [Citation11]; Luxturna (an adeno-associated virus vector-based gene therapy indicated for the treatment of patients with confirmed biallelic RPE65 mutation-associated retinal dystrophy) received FDA approval in December 2017 for sight-restoring in patients with Leber’s congenital amaurosis related blindness [Citation12,Citation13].
Table 1. Clinical trials based on genomic editing (http://clinicalTrials.gov).
All these advancements are raising hopes regarding the potential eradication of previously untreatable diseases, but nevertheless, there are important concerns related to the ethical aspects. As per example, gene-edited crops could be subject to the same rigorous regulations as conventional genetically modified (GM) organisms, as Court of Justice of the European Union ruled on July 25th, 2018. Also, the long-term effects caused by irreversible changes in the DNA structure are questionable when comes to patients’ involvement. In this sense, a new type of approach is currently emerging into preclinical testing, where instead of inducing permanent modifications within the DNA sequence, a more versatile type of nucleic acid is modified – the RNA. The advantage arises from the half-life of the messenger RNA (mRNA), where the modifications are still translated functionally into modified proteins, but the effect is time and also disease stage manageable.
Point mutations induce single-nucleotide substitutions that can further generate modified forms of proteins specifically associated with different diseases like cancer [Citation14], cystic fibrosis [Citation15], hemophilia A [Citation16] and chronic granulomatous disease [Citation17]. The correction of these point mutations can be made at either DNA or RNA levels with equivalent effects upon the translated protein [Citation18]. RNA editing occurs at post-transcriptional level with a functional outcome mainly upon different forms of proteins [Citation19]; the modifications in the RNA structure can occur through substitution, deletion or insertion [Citation20,Citation21]. In mammalian cell, two main families of enzymes are responsible for the RNA editing process, where adenosine is converted to inosine (guanosine-like complementarity) – adenosine deaminase acting on RNA (ADAR) family – and cytosine is converted to uracil – AID-apolipoprotein B mRNA editing enzyme(APOBEC) enzyme family [Citation22–Citation24]. These modifications can take place in different RNA types and regions; while AID-APOBEC activity has been detected mainly in the 3ʹ-UTRs [Citation24], ADAR can undertake a wide range of RNAs, including primary microRNA transcripts with effects upon gene silencing [Citation25,Citation26]. Even though these correcting processes consist of a single-nucleotide change, the downstream effects can significantly alter the phenotype of a cell. Substitutions in target spots can influence processes related to signaling, catalysis and also post-transcriptional modifications, disrupt the open reading frames, microRNA seed sequences and also splicing signals [Citation27]. Additionally, Shah et al. identified a distinct RNA editing mechanism, independent of APOBEC or ADAR, based on the allelic imbalances of guanosine and thymine alleles within the CCAT2 long non-coding RNA overlapping a functional cancer risk related SNP in myelodysplastic patients and a mouse model [Citation28].
3. Is RNA-based genetic engineering the safer option?
RNA-based gene therapy could rapidly evolve into clinical practice if we take into consideration that for some pathologies, only partial restoration of protein homeostatic activity is sufficient for significant disease amelioration – e.g. Lesch-Nyhan syndrome can switch to a mild form of hyperuricemia after 8% improvement in enzyme activity [Citation29]. Moreover, potential off-target effects in one RNA molecule will be much attenuated than in the case of DNA editing strategies due to the temporary nature of the RNA molecules and also the editing will take place only in the cells that actually express the targeted gene.
At the end of 2017, researchers revealed such a form of gene therapy through enrolment of a different Cas enzyme – Cas13 and CRISPR system entitled REPAIR – RNA Editing for Programmable A to I replacement () [Citation30]. Shortly, Cas9 within the classical CRISPR/Cas9 system was replaced with Cas13 that binds RNA instead of DNA; the latest was further fused with ADAR2, which catalyzes the deamination of adenine to inosine. The specificity of the cut is directed by a guide RNA (gRNA). As stated by the authors, the degradable character of the RNA transforms REPAIR into a tool with reversible consequences, being safer than those who alter the genome. Furthermore, a more targeted version – REPAIRv2 – developed by the same group, achieved up to 50% targeting efficiency without significant off-target events. The feasibility of the method was tested for Fanconi anemia and X-linked nephrogenic diabetes insipidus.
Table 2. Available nucleases for gene editing.
However, the concept of site-directed A-to-I RNA editing has been introduced before the identification of CRISPR/Cas based methods. The first engineered ribonucleoprotein for site-directed RNA modification has been implemented under the form of SNAP–ADARs (adenosine deaminases acting on RNA) [Citation31] and is also the best-characterized method of its purpose at the current time. The strategy is based on the self-labeling protein SNAP-tag developed from human O6-alkylguanine-DNA alkyltransferase that binds in a covalent manner to a custom-made gRNA containing O6-benzylguanine (BG) tag. Competing with Cas13b-based methods, SNAP-ADAR was used for targeted adenosine to inosine editing within KRAS and STAT1, two crucial molecules within a large plethora of disease states, including cancer. The authors obtained up to 90% efficiency and also optimal precision and editing duration; specifically, off-target effects within the mRNA-gRNA duplex were diminished by genomic integration and showed superior specificity in comparison with the methods based on Cas13b where the off-target effects were amplified by the overexpressed editases [Citation32]. Another approach, namely, λN-ADAR2, takes advantage of the non-covalent fusion of a gRNA-deaminase complex [Citation33]. Potentiated efficiency was obtained within the same strategy by introducing a highly active mutant form of ADAR2, namely ADAR2 E/Q, further fused with up to four λN peptides (4λN-ADAR2 E/Q) [Citation34]. The above-presented methods (CRISPR dependent and independent) were recently revised and compared [Citation35]. In the constant attempt to reduce the off-target effects of RNA editing, several groups proposed the idea of taking advantage of the naturally occurring ADARs. Extending the idea of the early study of Woolf where the possibility of recruiting an endogenous ADAR in Xenopus was proved [Citation36], recent studies developed principle-based similar approaches [Citation37–Citation39]. The first study of such manner in a human study models, managed to modify in Hela cells a mutation within PINK1 associated with loss of function effects and forced PINK1 connected mitophagy; this was done by designing a gRNA capable to capture human ADAR2 toward selected mRNA sites [Citation37].
The ability of this RNA-based tool of introducing single-nucleotide changes can be adapted for numerous therapeutic purposes, including modification of primary microRNA sequences with the final purpose of impairing the ability of the ‘seed’ sequence to bind and inhibit complementary genes. MicroRNAs have evolved as important regulators of carcinogenesis [Citation40–Citation42], where numerous therapeutic attempts are made with exogenous mimic or inhibitor sequences for the attenuation of malignant hallmarks [Citation43–Citation46]. However, the delivery method and its specificity, sometimes the necessity of multiple administrations and potential immunogenicity and toxicity of the exogenous sequence are just several factors that are hampering the clinical implementation of microRNA therapeutics. Approaches such as RNA editing of primary microRNA sequence can limit the off-target effects and increase the specificity of the microRNA-based therapies.
4. Novel diagnostic value of genome editing tools
The use of CRISPR/Cas system for RNA-based gene therapy is currently raising numerous potential therapeutic applications; however, this new system can have also applications in the diagnostic sector. Several separate groups are repurposing the basics of CRISPR/Cas in novel diagnostic methods like viral infections difficult to detect by standard assays due to low numbers of circulating viral particles – eg. Zika virus.
New CRISPR sensing systems that have a collateral activity – the cleavage of nearby sequences in an indiscriminately manner – were used as a signal amplifier for the identification of a specific molecule [Citation47] (). The first one, DETECTR (DNA endonuclease targeted CRISPR trans reporter) [Citation48] uses type V CRISPR and Cas12a with collateral activity for the detection of viral DNA; Cas12a is specifically constructed to bind viral DNA where the collateral cleavage ability is disrupting a ssDNA ‘reporter’ marked with a fluorophore at one end and with a quencher molecule at the other designed to capture the fluorescence. Upon cleavage of the ssDNA, the emitted fluorescence (amplified through recombinase polymerase for viral DNA) can be detected for diagnostic of viral infection. This approach can detect minimal concentration of viral particles – one molecule per microliter of sample and also distinguish between different strains of HPV. Through a similar strategy SHERLOCK (specific high sensitivity enzymatic reporter unlocking) was implemented [Citation49] by adapting the detecting system on the ability of Cas13 to cleave RNA sequences. Still, the second system has several advantages in terms of specificity since it uses simultaneously four Cas13 proteins, each with their reporter, that allow the detection of more than one virus at once (maximum four) and is also based on an improved recombinase polymerase amplification for more sensitive diagnosis. Finally, another group [Citation50] based their method on the strategic parameters of SHERLOCK but designed the system to detect the viral infection in body fluids like urine and saliva upon enzyme inactivation. They named the system HUDSON (heating unextracted diagnostic samples to obliterate nucleases). Moreover, their method can distinguish between mutated strains at a sensitivity of a single nucleotide modification – detection of Zyka virus in different geographic areas and also with different mutational pattern.
Figure 1. Diagnostic systems based on the activity of specific Cas enzymes and their collateral activity. DETECTR system is composed of Cas12a enzyme, a specific gRNA for viral DNA recognition and an ssDNA construct loaded with a quencher and a fluorophore; in the OFF state of the system, the fluorescence is captured by the quencher (DETECTR system A). After target recognition, Cas12a cleaves the viral DNA, but also the ssDNA construct, liberating the fluorophore from the quencher (DETECTR system B). SHERLOCK system has similar principles, although the entire construct contains also an additional enzyme – Csm6 – that cleaves a secondary ssDNA construct (SHERLOCK system A). The cleavage products of Cas13 activate Csm6 that further disrupts the second ssDNA segment and amplifies the fluorescence signal (SHERLOCK system B).
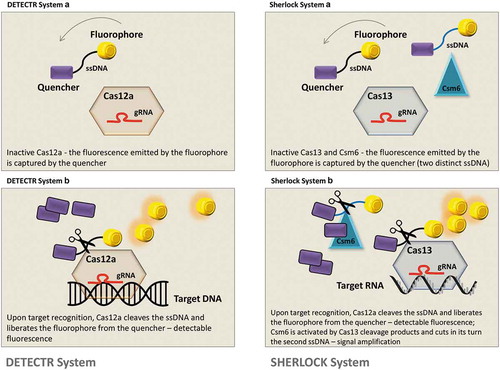
These diagnostic tools are expending the possibilities around the CRISPR/Cas system, applications that are also susceptible to a more rapid implementation within the market due to the economical friendly status. Moreover, these instruments can be relatively easy adapted for the minimally invasive detection of other pathologies like cancer by sensing circulating tumor DNA in the body fluids of patients.
5. Conclusion
Until not long ago, targeted corrections within the DNA sequence using nucleases like Cas9 were considered the future of medicine and many studies were concentrated on minimizing the off-target effects of the genomic editing tools for possible implementation within the clinic. Even so, recent research has uncovered alternative possibilities of genetic manipulation by counteracting the RNA sequence with minimized long-term effects due to the degradable character of this type of nucleic acid. The debate between the genetic manipulation of DNA versus RNA is still on, where the future will reveal which strategy will finally achieve its place in the clinic. On the other side, similar strategies are used for other purposes: diagnostic of viral infections with at an unprecedented sensitivity. This type of research has minimal risks for the patient and can be also adapted for detection of other pathologies based on distinctive markers within liquid biopsies.
Declaration of interest
The authors have no relevant affiliations or financial involvement with any organization or entity with a financial interest in or financial conflict with the subject matter or materials discussed in the manuscript. This includes employment, consultancies, honoraria, stock ownership or options, expert testimony, grants or patents received or pending, or royalties.
Reviewers Disclosure
Peer reviewers on this manuscript have no relevant financial relationships or otherwise to disclose.
Additional information
Funding
References
- Calin GA, Croce CM. Genomics of chronic lymphocytic leukemia microRNAs as new players with clinical significance. Semin Oncol. 2006 Apr;33(2):167–173. PubMed PMID: 16616063.
- Munker R, Calin GA. MicroRNA profiling in cancer. Clin Sci. 2011 Aug;121(4):141–158. PubMed PMID: 21526983; eng.
- Ling H, Vincent K, Pichler M, et al. Junk DNA and the long non-coding RNA twist in cancer genetics. Oncogene. 2015 Sep 24;34(39):5003–5011. PubMed PMID: 25619839; PubMed Central PMCID: PMC4552604.
- Mullin E 2017 Was the year of gene-therapy breakthroughs mit technology review 2018. [cited 2018 Aug 13]. Available from: https://www.technologyreview.com/s/609643/2017-was-the-year-of-gene-therapy-breakthroughs/
- Chira S, Gulei D, Hajitou A, et al. CRISPR/Cas9: transcending the reality of genome editing. Mol Ther Nucleic Acids. 2017 Jun 16;7:211–222. PubMed PMID: 28624197; PubMed Central PMCID: PMCPmc5415201. eng.
- Guha TK, Wai A, Hausner G. Programmable Genome editing tools and their regulation for efficient genome engineering. Comput Struct Biotechnol J. 2017;15:146–160. PubMed PMID: 28179977; PubMed Central PMCID: PMCPMC5279741.
- Chira S, Gulei D, Hajitou A, et al. Restoring the p53 ‘guardian’ phenotype in p53-deficient tumor cells with CRISPR/Cas9. Trends Biotechnol. 2018 Jul;36(7):653–660. PubMed PMID: 29478674.
- Gulei D, Berindan-Neagoe I. CRISPR/Cas9: a potential life-saving tool. What’s next? Mol Ther Nucleic Acids. 2017 Dec 15;9:333–336.
- Ribeil JA, Hacein-Bey-Abina S, Payen E, et al. Gene therapy in a patient with sickle cell disease. N Engl J Med. 2017 Mar 2;376(9):848–855. PubMed PMID: 28249145.
- Zheng PP, Kros JM, Li J. Approved CAR T cell therapies: ice bucket challenges on glaring safety risks and long-term impacts. Drug Discov Today. 2018 Jun;23(6):1175–1182. PubMed PMID: 29501911.
- Hirsch T, Rothoeft T, Teig N, et al. Regeneration of the entire human epidermis using transgenic stem cells. Nature. 2017. 11/08/online 551: 327.https://www.nature.com/articles/nature24487#supplementary-information
- FDA. FDA approves novel gene therapy to treat patients with a rare form of inherited vision loss U.S. Department of Health and Human Services2018 [ cited 2018]. [cited 2018 Aug 13]. Available from: https://www.fda.gov/newsevents/newsroom/pressannouncements/ucm589467.htm
- Bennett J, Wellman J, Marshall KA, et al. Safety and durability of effect of contralateral-eye administration of AAV2 gene therapy in patients with childhood-onset blindness caused by RPE65 mutations: a follow-on phase 1 trial. Lancet. 2016;388(10045):661–672.
- Leisegang M, Engels B, Schreiber K, et al. Eradication of large solid tumors by gene therapy with a t-cell receptor targeting a single cancer-specific point mutation. Clin Cancer Res off J Am Assoc Cancer Res. 2016 Jun 1;22(11):2734–2743. PubMed PMID: 26667491; PubMed Central PMCID: PMC4891260.
- Ferec C, Audrezet MP, Mercier B, et al. Detection of over 98% cystic fibrosis mutations in a Celtic population. Nat Genet. 1992 Jun;1(3):188–191. PubMed PMID: 1284639.
- Higuchi M, Kazazian HH Jr., Kasch L, et al. Molecular characterization of severe hemophilia A suggests that about half the mutations are not within the coding regions and splice junctions of the factor VIII gene. Proc Natl Acad Sci U S A. 1991 Aug 15;88(16):7405–7409. PubMed PMID: 1908096; PubMed Central PMCID: PMC52304.
- Bolscher BG, de Boer M, de Klein A, et al. Point mutations in the beta-subunit of cytochrome b558 leading to X-linked chronic granulomatous disease. Blood. 1991 Jun 1;77(11):2482–2487. PubMed PMID: 1710153.
- Rees HA, Liu DR. Base editing: precision chemistry on the genome and transcriptome of living cells. Nat Rev Genet. 2018 Dec;19(12):770–788. PubMed PMID: 30323312; eng.
- Maas S, Rich A. Changing genetic information through RNA editing. Biol Sci Nat Sci. 2000 Sep;22(9):790–802. PubMed PMID: 10944581.
- Kim H, Kim JS. A guide to genome engineering with programmable nucleases. Nat Rev Genet. 2014 May;15(5):321–334. PubMed PMID: 24690881.
- Nishikura K. Functions and regulation of RNA editing by ADAR deaminases. Annu Rev Biochem. 2010;79:321–349. PubMed PMID: 20192758; PubMed Central PMCID: PMC2953425.
- Nishikura K. A-to-I editing of coding and non-coding RNAs by ADARs. Nat Rev Mol Cell Biol. 2016 Feb;17(2):83–96. PubMed PMID: 26648264; PubMed Central PMCID: PMC4824625.
- Slotkin W, Nishikura K. Adenosine-to-inosine RNA editing and human disease. PubMed PMID: 24289319; PubMed Central PMCID: PMC3979043 Genome Med. 2013;511:105.
- Rosenberg BR, Hamilton CE, Mwangi MM, et al. Transcriptome-wide sequencing reveals numerous APOBEC1 mRNA-editing targets in transcript 3ʹ UTRs. Nat Struct Mol Biol. 2011 Feb;18(2):230–236. PubMed PMID: 21258325; PubMed Central PMCID: PMC3075553.
- Blow MJ, Grocock RJ, van Dongen S, et al. RNA editing of human microRNAs. Genome Biol. 2006;7(4):R27. PubMed PMID: 16594986; PubMed Central PMCID: PMC1557993.
- Kawahara Y, Zinshteyn B, Sethupathy P, et al. Redirection of silencing targets by adenosine-to-inosine editing of miRNAs. Science. 2007 Feb 23;315(5815):1137–1140. PubMed PMID: 17322061; PubMed Central PMCID: PMC2953418.
- Azad MTA, Bhakta S, Tsukahara T. Site-directed RNA editing by adenosine deaminase acting on RNA for correction of the genetic code in gene therapy [Original Article]. Gene Ther. 2017;24(779):779–786. 12/07/online https://www.nature.com/articles/gt201790#supplementary-information
- Shah MY, Ferracin M, Pileczki V, et al. Cancer-associated rs6983267 SNP and its accompanying long noncoding RNA CCAT2 induce myeloid malignancies via unique SNP-specific RNA mutations. Genome Res. 2018 Apr;28(4):432–447. PubMed PMID: 29567676; PubMed Central PMCID: PMC5880235.
- Jinnah HA, De Gregorio L, Harris JC, et al. The spectrum of inherited mutations causing HPRT deficiency: 75 new cases and a review of 196 previously reported cases. Mutat Res. 2000 Oct;463(3):309–326. PubMed PMID: 11018746.
- Cox DBT, Gootenberg JS, Abudayyeh OO, et al. RNA editing with CRISPR-Cas13. Science. 2017 Nov 24;358(6366):1019–1027. PubMed PMID: 29070703; PubMed Central PMCID: PMC5793859.
- Stafforst T, Schneider MF. An RNA-deaminase conjugate selectively repairs point mutations. Angew Chem Int Ed in Engl. 2012 Oct 29;51(44):11166–11169. PubMed PMID: 23038402; eng.
- Vogel P, Moschref M, Li Q, et al. Efficient and precise editing of endogenous transcripts with SNAP-tagged ADARs. Nat Methods. 2018 Jul;15(7):535–538. PubMed PMID: 29967493; eng.
- Montiel-Gonzalez MF, Vallecillo-Viejo I, Yudowski GA, et al. Correction of mutations within the cystic fibrosis transmembrane conductance regulator by site-directed RNA editing. Proc Natl Acad Sci U S A. 2013 Nov 5;110(45):18285–18290. PubMed PMID: 24108353; PubMed Central PMCID: PMCPmc3831439. eng.
- Montiel-Gonzalez MF, Vallecillo-Viejo IC, Rosenthal JJ. An efficient system for selectively altering genetic information within mRNAs. Nucleic Acids Res. 2016 Dec 1;44(21):e157. PubMed PMID: 27557710; PubMed Central PMCID: PMCPmc5137428. eng.
- Vogel P, Stafforst T. Critical review on engineering deaminases for site-directed RNA editing. Curr Opin Biotechnol. 2018 Sep 5;55:74–80. PubMed PMID: 30193161; eng. .
- Woolf TM, Chase JM, Stinchcomb DT. Toward the therapeutic editing of mutated RNA sequences. Proc Natl Acad Sci U S A. 1995 Aug 29;92(18):8298–8302. PubMed PMID: 7545300; PubMed Central PMCID: PMCPmc41144. eng.
- Wettengel J, Reautschnig P, Geisler S, et al. Harnessing human ADAR2 for RNA repair - Recoding a PINK1 mutation rescues mitophagy. Nucleic Acids Res. 2017 Mar 17;45(5):2797–2808. PubMed PMID: 27907896; PubMed Central PMCID: PMCPmc5389476. eng.
- Heep M, Mach P, Reautschnig P, et al. Applying human ADAR1p110 and ADAR1p150 for site-directed RNA editing-G/C substitution stabilizes guideRNAs against editing. Genes. 2017 Jan 14;8(1). PubMed PMID: 28098820; PubMed Central PMCID: PMCPmc5295028. eng.
- Fukuda M, Umeno H, Nose K, et al. Construction of a guide-RNA for site-directed RNA mutagenesis utilising intracellular A-to-I RNA editing. Sci Rep. 2017 Feb 2;7:41478. PubMed PMID: 28148949; PubMed Central PMCID: PMCPmc5288656. eng.
- Berindan-Neagoe I, Calin GA. Molecular pathways: microRNAs, cancer cells, and microenvironment. Clin Cancer Res off J Am Assoc Cancer Res. 2014 Dec 15;20(24):6247–6253. PubMed PMID: 25512634; PubMed Central PMCID: PMC4461223.
- Redis RS, Berindan-Neagoe I, Pop VI, et al. Non-coding RNAs as theranostics in human cancers. J Cell Biochem. 2012 May;113(5):1451–1459. PubMed PMID: 22213511; PubMed Central PMCID: PMC3337365.
- Bullock M, Silva A, Kanlikilicer-Unaldi P, et al. Exosomal non-coding RNAs: diagnostic, prognostic and therapeutic applications in cancer. Noncoding RNA. 2015;1(1):53. PubMed PMID.
- Van Roosbroeck K, Fanini F, Setoyama T, et al. Combining anti-miR-155 with chemotherapy for the treatment of lung cancers. Clin Cancer Res off J Am Assoc Cancer Res. 2016 Nov 30 PubMed PMID: 27903673. doi: 10.1158/1078-0432.CCR-16-1025.
- Gulei D, Magdo L, Jurj A, et al. The silent healer: miR-205-5p up-regulation inhibits epithelial to mesenchymal transition in colon cancer cells by indirectly up-regulating E-cadherin expression. Cell Death Dis. 2018 Jan 19;9(2):66. PubMed PMID: 29352232; PubMed Central PMCID: PMCPmc5833765. eng.
- Braicu C, Ga C, Berindan-Neagoe I. MicroRNAs and cancer therapy - from bystanders to major players. Curr Med Chem. 2013;20(29): 3561–3573. PubMed PMID: 23834177; eng.
- Eastlack SC, Alahari SK. MicroRNA and breast cancer: understanding pathogenesis, improving management. Noncoding RNA. 2015 Apr 20;1(1):17–43. PubMed PMID: 29861413; PubMed Central PMCID: PMCPmc5932537. eng.
- Kocak DD, Gersbach CA. From CRISPR scissors to virus sensors. Nature. 2018 May;557(7704):168–169. 10.1038/d41586-018-04975-8. PubMed PMID: 29730672.
- Chen JS, Ma E, Harrington LB, et al. CRISPR-Cas12a target binding unleashes indiscriminate single-stranded DNase activity. Science. 2018 Apr 27;360(6387):436–439. PubMed PMID: 29449511; eng.
- Gootenberg JS, Abudayyeh OO, Kellner MJ, et al. Multiplexed and portable nucleic acid detection platform with Cas13, Cas12a, and Csm6. Science. 2018 Apr 27;360(6387):439–444. PubMed PMID: 29449508; PubMed Central PMCID: PMC5961727.
- Myhrvold C, Freije CA, Gootenberg JS, et al. Field-deployable viral diagnostics using CRISPR-Cas13. Science. 2018 Apr 27;360(6387):444–448. PubMed PMID: 29700266.