Abstract
The temperature dependence of the yield stress, effective stress and activation volume on the Cu content in steel was assessed using polycrystalline ultra-low carbon steels with 0.5, 1 and 2 mass% Cu added. A small hump was seen in the effective stress–temperature curve for ultra-low carbon steel, which has also been reported for high-purity single-crystal iron. The effective stress was found to decrease with increasing the Cu content. The activation volume was found to be linearly related to the temperature at low temperatures for all types of specimens considered. The activation volume was found to increase abruptly at a certain temperature, which increased with increasing the Cu content. The change in the relationship of the activation volume to temperature is discussed in this paper with respect to the thermally activated process of dislocation glide from the Peierls mechanism to the interaction between dislocations and solute Cu atoms.
1. Introduction
Ductile materials with high Peierls potential become brittle when deformed at low temperatures. The transition of the fracture mode from ductile to brittle is called brittle-to-ductile transition (BDT). St. John [Citation1] demonstrated, using single-crystal silicon, that the elementary process which controls the BDT is a thermally activated process because the following Arrhenius-type equation applies to the relationship between the BDT temperature and strain rate:(1)
where ε0 is a coefficient of the exponential term, ΔG is the activation energy, k is the Boltzmann constant and TBDT is the transition temperature. He concluded that the process that controls the BDT is dislocation glide because, in his experiment, the value of the activation energy obtained using Equation (Equation1(1) ) was close to that for dislocation glide. Some subsequent studies conducted using other pure single crystals, such as gallium nitride, sapphire and so on, clarified that the activation energies obtained with Equation (Equation1
(1) ) for those materials are also close to those for dislocation glide around the BDT temperature [Citation2–6]. The effects of additional elements on the BDT have also been investigated for single-crystal silicon. The BDT temperature was found to decrease with increasing the As dopant amount while activation energy obtained with Equation (Equation1
(1) ) was decreased with increasing the As dopant amount [Citation7]. The results of a discrete dislocation dynamics simulation indicated that the increase in the dislocation mobility decreases the BDT temperature [Citation8]. This suggests that the change in dislocation mobility with alloying elements strongly influences the BDT temperature.
In the cases of iron and steels, some elements are always added to achieve appropriate strength, deformability and hardenability for their practical use. It is essential to understand the effects of those elements on the BDT. It has been reported, for instance, that Ni and Cu decrease the BDT temperature, while Si and Al increase the BDT temperature [Citation9–12]. The decrease in the BDT temperature can be explained by the increase in the dislocation mobility at low temperatures, which is manifested by a decrease in the yield stress at low temperatures of steels with Ni added [Citation9,13,14]. Measurements of the temperature dependence of the yield stress and the activation volume provide insight into the thermal activation of dislocation glide. The temperature dependence of the activation volume is essential to presume the process of thermal activation in dislocation gliding, which controls the BDT. However, the relationship between the temperature/element dependence of the activation volume has not been clarified. Therefore, in the present study, the temperature dependence of the yield stress, the effective stress, and the activation volume of ultra-low carbon steels with Cu added were measured. The change in the activation volume with temperature and the Cu content was then examined, with a focus on the thermally activated process of dislocation glide.
2. Experimental procedure
Table shows the chemical compositions of the materials employed in this study. The base material was ultra-low carbon steel with a C concentration of less than 21 mass ppm. Specimens with 0, 0.5, 1 and 2 mass% of Cu added to the base material were labelled as 0Cu, 0.5Cu, 1Cu and 2Cu, respectively. The grain sizes of the 0Cu, 0.5Cu, 1Cu and 2Cu specimens were measured to be 147, 99, 101 and 67 μm, respectively. Specimens were hot rolled at 1293 K, then 0Cu, 0.5Cu and 1Cu were as air cooled and 2Cu was quenched in order to prevent the precipitation of Cu.
Table 1. Chemical compositions of the materials employed (mass%).
The parallel portions and widths of the tensile specimens were 8 and 2 mm, respectively, and their thickness was 0.9 mm. Strain gauges were attached to the parallel portions of the specimens. A Shimadzu AG-IS was used for the tests. Strain-rate jump tests were performed to obtain the activation volume. The strain rate was increased from 4.2 × 10−4 s−1 to 4.2 × 10−3 s−1 at the total strain of after approximately 0.02 during the tensile tests.
3. Results
Figure (a) and (b) show the stress–strain (s–s) curves obtained at room temperature (RT) and, 77 K, respectively. The yield stress was found to increase and the total elongation to decrease with decreasing temperature, which is typical of the temperature dependence of the s–s behaviour of body-centred cubic (bcc) crystals. The total elongations in some bcc C–Mn and C–Ni steels increases with decreasing temperatures at lower temperatures, which is one of properties of solid solution softening [Citation14,15]. Yield point phenomenon was observed in all specimens at RT, while continuous yielding was observed at 77 K. The yield elongation was shorter and the lower yield stress was increased with the increase in Cu at RT. The 0.2% proof stress of 0.5Cu, 1Cu and 2Cu was slightly higher than that of 0Cu at 77 K.
Figure 1. (colour online) Stress–strain curves for 0Cu, 0.5Cu, 1Cu and 2Cu. (a) at RT and (b) at 77 K.
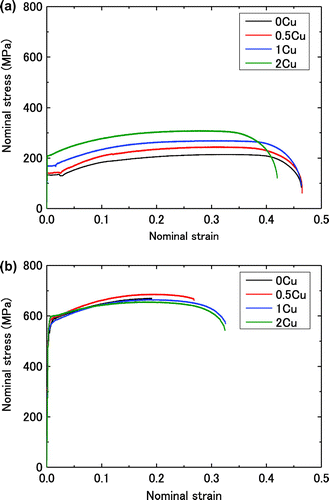
Figure shows SEM images of the side surfaces of the specimens after tensile tests at RT and 77 K. Figure (a)–(d) show that all specimens ruptured after necking at RT, indicating that there was not a significant difference in rupture behaviour for different Cu contents.
Figure 2. SEM images of side surfaces after tensile tests. (a)–(d) at RT and (e)–(h) at 77 K. (a) and (e) are for 0Cu, (b) and (f) are for 0.5Cu, (c) and (g) are for 1Cu, and (d) and (h) are for 2Cu.
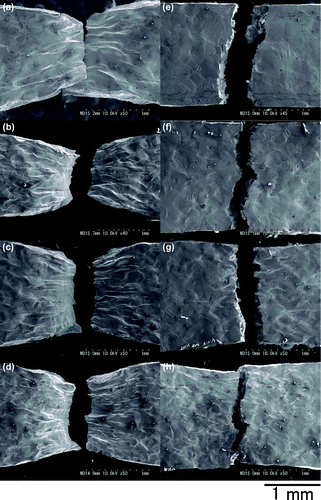
Several twins were observed on the side surfaces of 0Cu and 0.5Cu specimens that ruptured at 77 K, as shown in Figure (a) and (b). Twins are often observed at low temperatures in the deformation of bcc steels which contain few impurities. The twinning stress in those steels is usually much higher than the stress for the motion of slip dislocations at room temperature, therefore, motions of slip dislocations result in plastic deformations at room temperature. The stress for the motion of slip dislocations increases with the decrease in the deformation temperature, which is due to the high Peierls potential. When the stress for the motion of slip dislocations becomes high close to the twinning stress, deformation twinning results in plastic deformations. Twinning is competitive deformation mechanism with slip, therefore, deformation twins are usually observed at deformations at low temperatures in bcc steels while it appears at the late stage of plastic deformations in fcc crystals. Few twins were observed on the side surface of 2Cu. This suggests that twin formation was inhibited with increasing Cu content, as the increase in the Mn content inhibits the deformation twinning [Citation15].
Figure 3. (a) and (b) show enlarged images of Figure (e) and (f), respectively. Arrows in the figure indicate deformation twins.
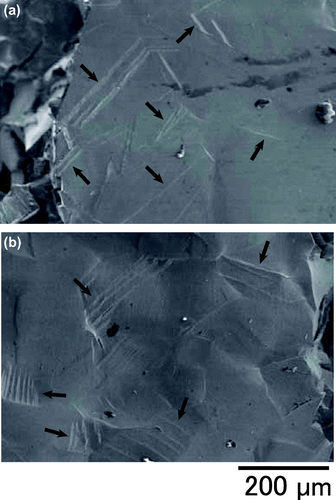
The area reduction at failure increased with increasing Cu content at 77 K, as shown in Figure (e)–(h), and a large number of slip bands were seen on the side surface of 2Cu, as shown in Figure (h), suggesting that Cu increases the deformability of steel at low temperatures. To investigate the change in dislocation activity with change in the Cu content, the temperature dependence of the yield stress was measured.
Here, since some specimens show a yield point phenomenon with Lüders band formation and some do not, the yield stress was defined as follows in this study. When specimens showed continuous yield, 0.2% proof stress was taken as the yield stress. When specimens showed a yield point phenomenon, the yield stress was defined at the stress obtained by extrapolating the smooth part of the s-s curves into the elastic line as shown in Figure .
Figure illustrates the temperature dependence of the yield stress. One plot point in the figure was obtained from a single experiment. Several experiments were performed at some temperatures, such as 77 K, room temperature and so on, where there are some plot points in the figure. It was selected in this study to obtain the series of temperature of the yield stress instead of performing many experiments in the limited numbers of temperatures. Although the relative large grain size would induce the slight deviation of the yield stress, the smooth temperature dependence of the yield stress in Figure suggests that the effect is not significant. The yield stress was observed to decrease with temperature for all specimens, which is typical in bcc crystals. A slight hump was observed in the temperature dependence of the yield stress for 0Cu between approximately 120 and 225 K, which is consistent with the behaviour reported by Kuramoto et al. for high-purity single-crystal iron [Citation16]. They measured the temperature dependence of the critical resolved shear stress and the activation volume of single-crystalline pure iron in the tensile direction for a single slip condition. A hump or convex part was observed in the plot of the activation volume versus temperature/the effective stress. They suggested that the Peierls potential of iron has a double-hump, explaining the hump in the curve. Itakura et al. calculated the shape of the Peierls potential in iron crystals with a first-principles calculation and showed that the Peierls potential does not have the double-hump but in fact smooth [Citation17].
The yield stresses for 0.5Cu, 1Cu and 2Cu were lower than that for 0Cu in the temperature range of the hump, i.e. between 120 and 200 K as shown in Figure . The yield stresses for 0.5 Cu, 1Cu and 2Cu were higher than that for 0Cu below 120 K. The yield stress consists of two components: the effective stress, σe, which is temperature dependent, and the athermal stress, σath, which is temperature independent. Thus, the yield stress can be described by the following equation:(2)
The athermal stress was defined in this study as the yield stress at 350 K, at which the temperature dependence of the yield stress nearly disappeared. The values of the athermal stress were 78, 99, 135 and 196 MPa for 0Cu, 0.5Cu, 1Cu and 2Cu, respectively. The effective stress at each temperature was obtained by subtracting the value of the athermal stress from the value of the yield stress at the same temperature.
Figure illustrates the temperature dependence of the effective stress. The effective stresses for 1Cu and 2Cu were smaller than the effective stress of 0Cu at any temperature, while that of 0.5Cu was nearly the same as that of 0Cu below 110 K. The effective stress of 2Cu was slightly lower than that of 1Cu throughout the range of test temperatures. The effective stress is relating to the process of thermal activation of dislocation glide, so a decrease in the effective stress with increasing Cu content suggests an increase in the dislocation mobility. In addition, focusing on the results for 1Cu and 2Cu, Figure shows that the effective stress plots on a straight line at temperatures below approximately 200 K, while the line is curved at temperatures above approximately 200 K. The change in the slope of the curves for effective stress versus temperature indicates that there is a boundary temperature for the change in the thermally activated process of dislocation glide. Therefore, the temperature dependence of the activation volume was measured to assess the change in the thermally activated process of dislocation glide with respect to both temperature and Cu content.
The activation volume is defined as a function of the strain rate, , determined in tensile tests, according to the following equation [Citation18]:
(3)
where M is a Taylor factor of 2 [Citation19] and τe is the effective shear stress, which is equal to . Figure shows a schematic of a s–s curve when the strain rate was changed from
to
(
>
) for the specimens showing Lüders bands. In this study, the strain rate was changed from 4.2 × 10−4 s−1 to 4.2 × 10−3 s−1. When the strain rate was changed to be higher, the flow stress increased. The increase value in the flow stress corresponds to that in the effective stress. The flow stress after the increase in the strain rate was obtained at the intersection between the extrapolation of the s–s curve in the strain rate of
and the slope just after the change in the strain rate as shown in Figure . The value of ∂σe was obtained from Δσe in Figure . The activation volume was obtained at the strain of after approximately 0.02 where Lüders bands have been developed completely.
Figure 6. Schematic of a s–s curve in a strain rate jump test for the specimens showing Lüders bands, where >
σy denotes the yield stress defined in this study.
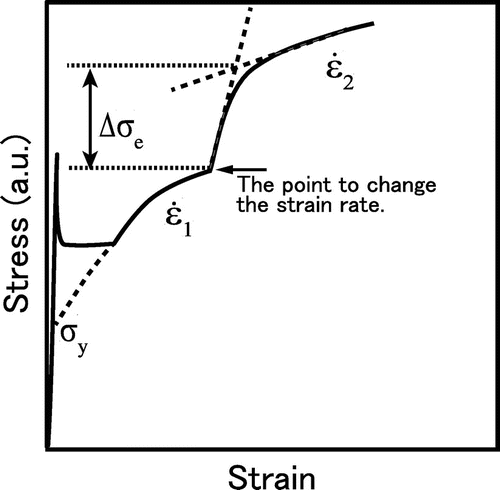
Figure illustrates the temperature dependence of the activation volume. In this figure, the vertical axis is normalised with respect to the cube of Burgers vector for α-iron (b = 0.25 nm). The activation volume increases linearly with temperature below approximately 175 K for any Cu content. The value of the activation volume is below 30b3. The value is close to those reported for the dislocation glide in the Peierls mechanism [Citation16,20–22]. The dependence of the activation volume on the Cu content is nearly negligible below 175 K, which suggests that the activation area for double-kink nucleation should not be influenced by Cu. The activation volume starts to deviate from linear behaviour at temperature above approximately 175 K. The temperature at which this deviation occurs depends on the Cu content; the higher the Cu content is the lower the deviation temperature is. The temperature for the deviation from a linear relationship, or in other words, the abrupt increase in the activation volume, depends on the Cu content. The relationship between the rapid increase in the activation volume and the change in the thermally activated process of dislocation glide is discussed in the next section.
4. Discussion
There are two steps in the thermally activated process of dislocation glide in bcc alloys; one is overcoming Peierls barrier and the other is overcoming the interaction of solute atoms. In pure crystals with high Peierls potential, such as iron, the thermally activated process of controlling the dislocation glide involves nucleating a double-kink to overcome the Peierls barrier [Citation23]. The dislocation velocity in this process is given by the following equation:(4)
where v0, τ0 and m are constants; T is the absolute temperature; k is the Boltzmann constant; and En and Em are the activation energies for double-kink nucleation and migration, respectively. Dislocation glide is considered to be controlled by the frequency of double-kink nucleation at low temperatures, because En >> Em.
On the other hand, in alloys, dislocations interact with solute atoms in addition to overcoming the Peierls barrier. The edge components of dislocations mainly interact with solute atoms, based on elastic theory. The force on dislocations required to overcome the Peierls barrier is much larger than that required to overcome the interaction with solute atoms in bcc crystals. Therefore, the interaction between dislocations and solute atoms appears in cases that the applied effective stress on a dislocation is much smaller than the effective stress for double-kink nucleation at 0 K.
The elementary process of dislocation glide as a thermally activated process in bcc alloys is discussed here with respect to two temperature ranges: (1) low temperature for high effective stress on a dislocation, and (2) high temperatureFootnote1 for low effective stress on a dislocation. In the first case i.e. the temperature range of (1), the first step for a dislocation to move is the thermally activated process of double-kink nucleation to overcome the Peierls barrier, which is the same as in the case of pure bcc crystals. Pure edge components of the dislocation migrate along the ridge of the Peierls barrier. Although the interaction between the edge components of the dislocation and solute atoms should occur during the migration, the edge components overcome the interaction only with a sufficiently high effective stress. That is, solute atoms do not work as short-range barriers, so dislocations overcome the barrier only with the effective stress, without thermal assistance. The elastic interaction between dislocations and solute atoms does not increase the activation energy for dislocation glide in this temperature range.
Solute Cu appears to decrease En in Equation (4) because the effective stress decreases with increasing the Cu content, as seen in Figure . The mechanism behind the decrease in En was not determined in this study. However, two types of mechanisms can be suggested. One mechanism involves Cu reducing the Peierls barriers, which could be presumed from the calculation of the atomic potential of the elements. Gordon and Neeraj [Citation24] demonstrated in atomistic modelling that the energy barrier for atomic sliding decreases with increasing the content of Cu or some other elements in α-iron. The other mechanism involves solute atoms decreasing the double-kink nucleation energy. Sato and Meshii suggested that the dislocation line was bowed by the elastic field because of solute atoms [Citation25]. Because the bowing direction of the dislocations is the same as that of nucleating double-kinking in overcoming the Peierls barrier, solute atoms can be inferred to decrease the net energy required for double-kink nucleation.
In second case i.e. the temperature range of (2), the nucleation rate of a double-kink is sufficiently high because of the thermal activation at high temperatures where the effective stress on a dislocation is low. Dislocation segments tend to be round, i.e. mixed dislocations, at high temperatures, while straight pure screw dislocations are dominant at low temperatures [Citation26,27]. The effective stress on the dislocation becomes lower than the stress required for dislocations to overcome the solute atoms with the effective stress alone, so the motion of dislocations with edge components becomes a thermally activated process that overcomes the solute atoms. Therefore, the activation energy in this temperature range contains the energy required for dislocations to overcome the interaction with solute atoms as well as the energy for the double-kink nucleation. This is manifested as the deviation from linear activation volume behaviour at certain temperatures. Thus, Figure suggests that the thermal activation for dislocations to overcome the interaction with solute atoms appear approximately above 225 K, 200 K and 170 K for 0.5Cu, 1Cu and 2Cu, respectively. It should be stressed here that there are two temperature regimes for the thermally activated process of dislocation glide in bcc; one is that the Peierls barrier is dominant, and the other is that the interaction between dislocations and solute atoms is dominant. The relationship between the activation volume and effective shear stress is discussed next.
Figure illustrates the dependence of the activation volume on the effective shear stress. The trends are nearly the same for all Cu contents except 0Cu. Figure indicates that the activation volumes for 0.5Cu, 1Cu and 2Cu deviate from a linear relationship between the activation volume and effective shear stress beyond approximately 50 MPa. This suggests that this is the maximum stress for dislocations required to overcome the interaction with solute atoms. Although the effective shear stress should depend on the concentration of Cu, it seems to be nearly the same for 0.5Cu, 1Cu and 2Cu. This might be due to fact that the experiment performed in this study did not have sufficient precision to distinguish differences in the critical stress.
To clarify that the activation volume relationship with increasing temperature deviates from a straight line is due to the onset of the interaction between dislocations and solute atoms, we performed additional experiments with another type of specimen. Over-aged 2Cu at 873 K for 518.4 ks was employed for these experiments. This material should contain fewer solute Cu atoms than not-aged 2Cu because supersaturated Cu atoms precipitate after the heat treatment.
Figure illustrates the temperature dependence of the activation volume of aged 2Cu in comparison with that of 0.5Cu and 2Cu without ageing. The temperature of aged 2Cu at which the activation volume deviates from a linear relationship with respect to temperature is higher than that of 2Cu without ageing. The relationship for aged 2Cu is nearly the same as that for 0.5Cu. Therefore, it can be concluded that the abrupt increase in the activation volume that occurs above 150 K is due to the interaction of dislocations and solute atoms as a thermally activated process of dislocation glide.
Figure 9. (colour online) Temperature dependence of activation volume for aged 2Cu. The results for 0.5Cu and 2Cu are the same as those shown in Figure .
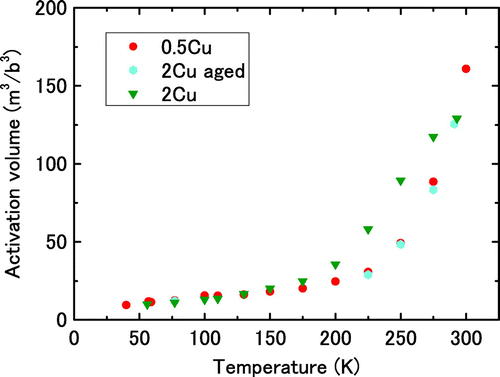
Figure shows force–displacement curves with respect to a dislocation when the dislocation overcomes short-range barriers. The activation force on the dislocation with the length of L under the effective shear stress of τe is given by τebL. Figure (a) shows the force–displacement curve for the double-kink nucleation. Since the double-kink nucleation stress is relatively high and the activation distance of the barrier is short, the potential curve should be high and sharp. The maximum effective stress for the double-kink nucleation is given as , where the activation distance is small.
Figure 10. Schematic drawing of the force on a dislocation with respect to distance in a thermally activated process: (a) double-kink nucleation, (b) interaction with a solute atom, and (c), (d) simultaneous double-kink nucleation and interaction with a solute atom.
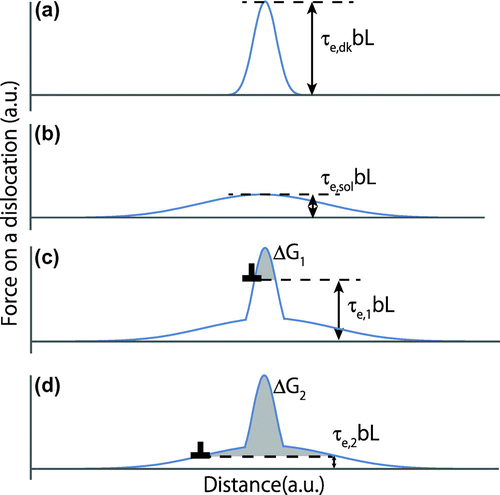
Figure (b) shows a force–displacement curve for the interaction between a dislocation and a solute atom. Since the stress on the dislocation to overcome the interaction should be much lower than that corresponding to the double-kink nucleation and since the activation distance of the barrier is much wider than that of double-kink nucleation, the curve should be small with a long tail. The maximum effective stress for dislocations to overcome the interaction nucleation is given as , where the activation distance is larger than that for double-kink nucleation. The potential curve should be short and has a long tail.
The total force in such cases for a dislocation to overcome both the Peierls barrier with the formation of the double-kink and the solute atom simultaneously can be expressed as the curves shown in Figure (c) and (d), which are obtained by combining the force–displacement relationships in Figure (a) and (b). At low temperatures, the effective stress τe,1 is as shown in Figure (c), assuming L is constant. The thermally activated process in this stress/temperature regime is only the double-kink nucleation under the activation energy of ΔG1. The changes in ΔG and in the activation volume are gradual, as expected from Figures and .
When the temperature increases, approximately above 225 K, 200 K, and 170 K for 0.5Cu, 1Cu, and 2Cu, respectively, as shown in Figure , the effective stress decreases to be as shown in Figure (d). The dominant thermally activated process in this stress/temperature regime for the dislocation glide is the interaction between dislocations and solute atoms under the activation energy of ΔG2. The changes in ΔG and the activation volume are much larger than those at low temperatures, as shown in Figures and . Thus, the solid solution hardening in bcc alloys appears as a thermally activated process only if the effective stress is lower, i.e. temperature is sufficiently higher.
Although the specimens employed in this study contain 17–21 mass ppm carbon as indicated in Table , the effect of carbon has not been discussed so far. Since the carbon concentration in each specimen is nearly the same, it is possible to consider that the change in the activation volume in Figure should represent the effect of Cu. In addition to that, it is considered that there is no influence of static strain ageing due to C in this study, since the strain rate jump tests to obtain the activation volume were performed at the strain after the completion of the formation of Lüders bands. The deviation of the activation volume from the linear behaviour as shown in Figures and should represent the change in the thermally activated process of dislocation glide. Therefore, a similar trend in the activation volume evolution of 0Cu with others indicates that the change which occurred in the thermally activated process of dislocation glide in 0Cu was the same as those in 0.5Cu, 1Cu and 2Cu. It is speculated that the main thermally activated process of dislocation glide in that temperature range (>250 K) is the interaction between dislocations and solute carbon atoms in 0Cu as solution hardening. This will be discussed further in a separated paper.
5. Conclusions
In the present study, the temperature dependence of the yield stress, effective stress and activation volume of ultra-low carbon steel was measured for steels with Cu contents up to 2 mass%. The effective stress was found to decrease with increasing Cu content. The temperature dependence of the activation volume was also influenced by the Cu content. The change in activation volume with temperature and the Cu content was examined. It is suggested that the abrupt increase in the activation volume that occurs with increasing temperature reflects the onset of the interaction between dislocations and solute atoms as a thermally activated process.
Disclosure statement
No potential conflict of interest was reported by the authors.
Notes
1. “High temperature” does not mean that for creep deformation here.
References
- C. St. John, The brittle-to-ductile transition in pre-cleaved silicon single crystals, Philos. Mag. 32 (1975), pp. 1193–1212.
- H.S. Kim and S. Roberts, Brittle-ductile transition and dislocation mobility in sapphire, J. Am. Ceram. Soc. 77 (1994), pp. 3099–3104.10.1111/jace.1994.77.issue-12
- S. Fujita, K. Maeda, and S. Hyodo, Dislocation-mobility-controlled cracking in GaAs caused by constant-rate indentaion, Philos. Mag. A 65 (1992), pp. 131–147.10.1080/01418619208201485
- P.B. Hirsch, A.S. Booth, M. Ellis, and S.G. Roberts, Dislocation-driven stable crack-growth by microclevage in semibrittle crsytals, Scr. Mater. 27 (1992), pp. 1723–1728.10.1016/0956-716X(92)90009-4
- F.C. Serbena and S.G. Roberts, The brittle-to-ductile transition in germanium, Acta Metall. 42 (1994), pp. 2505–2510.10.1016/0956-7151(94)90331-X
- J. Samuels and S.G. Roberts, The brittle-ductile transition in silicon. I. Experiments, Proc. R. Soc. Lond. A 421 (1989), pp. 1–23.
- M. Brede and P. Haasen, The brittle-to-ductile transition in doped silicon as a model substance, Acta Metall. 36 (1988), pp. 2003–2018.
- Y.-J. Hong, M. Tanaka, and K. Higashida, The effect of arsenic on the brittle-to-ductile transition in Si single crystals, Mater. Trans. 50 (2009), pp. 2177–2181.
- Y.T. Chen, D.G. Atteridge, and W.W. Gerberich, Plastic flow of Fe-binary alloys. I. A description at low temeprture, Acta Metall. 29 (1981), pp. 1171–1185.
- W.W. Gerberich, Y.T. Chen, D.G. Atteridge, and T. Johnson, Plastic flow of Fe-binary alloys. II. Application of the description to the ductile-brittle transition, Acta Metall. 29 (1981), pp. 1187–1201.
- J. Syarif, T. Tsuchiyama, and S. Takaki, Mechanism of toughening in ferritic iron by solute copper at low temperature, ISIJ Int. 43 (2003), pp. 1100–1104.
- M. Tanaka, K. Maeno, K. Higashida, M. Fujikura, and K. Ushioda, The increase in a brittle-to-ductile transition temperature in Fe–Al single crystals, ISIJ Int. 51 (2011), pp. 999–1004.
- K. Maeno, M. Tanaka, N. Yoshimura, H. Shirahata, K. Ushioda, and K. Higashida, Change in dislocation mobility with Ni content in ferritic steels and its effect on brittle-to-ductile transition, Tetsu-to-Hagané 98 (2012), pp. 667–674.
- K. Okazaki, Solid-solution hardening and softening in binary iron alloys, J. Mater. Sci. 31 (1996), pp. 1087–1099.
- M. Tanaka, K. Matsuo, N. Yoshimura, G. Shigesato, M. Hoshino, K. Ushioda, and K. Higashida, Effects of Ni and Mn on brittle-to-ductile transition in ultralow-carbon steels, Mater. Sci. Eng. A 682 (2017), pp. 370–375.
- E. Kuramoto, Y. Aono, and K. Kitajima, Thermally activated slip deformation of high-purity iron single-crystals between 4.2 K and 300 K, Scripta, Metall. 13 (1979), pp. 1039–1042.
- M. Itakura, H. Kaburaki, and M. Yamaguchi, First-principles study on the mobility of screw dislocations in bcc iron, Acta. Mater. 60 (2012), pp. 3698–3710.
- S.K. Mitra and J.E. Dorn, On nature of strain hardening in face-centered cubic metals, Trans. AIME 224 (1962), pp. 1062–1071.
- M.J. Marcinkowski and H.A. Lipsitt, The plastic deformation of chromium at low temperatures, Acta Metall. 10 (1962), pp. 95–111.
- E. Kubamoto, Y. Aono, K. Kitajima, K. Maeda, and S. Takeuchi, Thermally activated slip deformation between 0.7 and 77 K in high-purity iron single crsytals, Philos. Mag. A 39 (1979), pp. 717–724.
- D. Caillard, An in situ study of hardening and softening of iron by carbon interstitials, Acta Mater. 59 (2011), pp. 4974–4989.
- W.A. Spitzig and W.C. Leslie, Solid-solution softening and thermally activated flow in alloys of Fe with 3 at.% Co, Ni or Si, Acta Metall. 19 (1971), pp. 1143–1152.
- A. Seeger, The temperature dependence of the critical shear stress and of work-hardening of metal crystals, Philos. Mag. 46(382) (1955), pp. 1194–1217.
- P.A. Gordon and T. Neeraj, Do substitutional solutes soften or embrittle cracktips in alpha-Fe?, Acta. Mater. 57 (2009), pp. 3091–3100.
- A. Sato and M. Meshii, Solid solution softening and solid solution hardening, Acta Metall. 21 (1973), pp. 753–768.
- D. Caillard, Kinetics of dislocations in pure Fe. Part I. In situ straining experiments at room temperature, Acta. Mater. 58 (2010), pp. 3493–3503.
- D. Caillard, Kinetics of dislocations in pure Fe. Part II. In situ straining experiments at low temperature, Acta. Mater. 58 (2010), pp. 3504–3515.