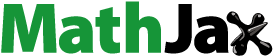
ABSTRACT
Agriculture and forest residues have a large energy potential and there are eligible to become sustainable promising energy resources in future. Present study deals with the analysis of thermo-physical properties, Fourier transform infrared spectroscopy (FTIR), and kinetics of corncob and eucalypts. The pyrolysis, Combustion characteristics and Kinetic deconvolution of biomass have been evaluated by thermo-gravimetric analyser from atmospheric temperature to 1100°C at a heating rate of 10°C/min. The kinetic analysis of biomass was determined by Flynn-Wall-Ozawa (FWO) methods. From the results, the average activation energies of corncob and eucalyptus were found as 91.29 and 77.21 kJ/mol respectively. The results showed that corncob was sensitive to rate of heating due to lower lignin content.
KEYWORDS:
Introduction
Biomass is seen as a renewable, secure and high potential sustainable energy option for the future due to the decrease of fossil fuel supply worldwide and the increasing need for energy security, environmental quality and climate change. Biomass was the first energy fuel to be discovered and used by human beings to fulfil everyday needs. Biomass ranks fourth worldwide as an energy reserve and supports about 14% of existing energy requirements (Naqvi et al. Citation2018). In the first quarter of this century, energy generation from biomass is expected to rise 3 times. Biomass is derived from biological component which was obtained from organisms (Zhang and Xu Citation2021). In present 10% of the global annual energy demand was fulfilled by biomass (Demirbas Citation2004). Biomass is a carbon-based resource which is considered to have an outstanding ability to lead the fulfilling energy requirements. A large part of the world’s energy needs are met from biomass, mainly in rural areas, where people use it in conventional ways to cooking the food and thermal application. Biomass from agricultural waste is one of the most effective sustainable sources of energy. In addition, the biomass used as fuel helps to reduce CO2 emissions by eliminating the combustion of petroleum based product and also reduces the NOx generation by reducing the nitrogen percentage in biofuels (Bram, De Ruyck, and Lavric Citation2009). There is various kind of biomass fuels available, including timber, short-rotation agriculture crop (rice husk, pearl millet cob and corn cob), herbaceous plants (Jeguirim and Trouvé Citation2009; Jeguirim, Dorge, and Trouvé Citation2010), municipal solid waste (Jayaraman, Kok, and Gokalp Citation2017), Biomass like corncob and eucalyptus are useful source of energy which is abundant in natures (Pinto et al. Citation2017). Biofuel produced from biomass like oil or gas can be used as substitutes for petroleum refinery product. Biomass fuel is an unpolluted and cheap energy source due to the low sulphur and no lead content than petroleum fuel. A large source of biomass resources present in nature like agricultural residue and municipal solids wastes in rural and urban areas can be recycled as an energy source for producer gas generation (White, Catallo, and Legendre Citation2011; Wilson et al. Citation2011; Pinto et al. Citation2017). Biomass can be converted into useful solid, liquid and gaseous fuels by means of thermochemical and biological conversion processes (Ferreira et al. Citation2016). In practice various biochemical and thermochemical processes used for conversion of biomass feedstocks into biofuels. The chemical composition, thermal degradation behaviour and the reactivity of these biomass resources during their thermochemical transformation have to be analysed for the effective design and operation of the thermochemical conversion units. Thermochemical conversion process include various method such as carbonisation, pyrolysis, biomass gasification, conversion of biomass by hydrothermally and combustion etc. (Tippayawong et al. Citation2013; Jaroenkhasemmeesuk and Tippayawong Citation2015; Wilk et al. Citation2016; Punnarapong, Sucharitakul, and Tippayawong Citation2017). Pyrolysis is one of these methods, the thermal breakdown of biomass in the absence of oxygen, which has been developed extensively as a promising technique for extracting biofuels or chemicals from different forms of biomass. The generation of char, bio oil and syngas depend on the condition of pyrolysis (Bach and Chen Citation2017). The TGA studies are very useful in studying the thermal behaviour of biomass and knowledge acquisition about the thermal stability, chemical composition and structure of biomass. Numerous researchers have investigated pyrolysis of biomass on the basis of its key components, e.g. hemicellulose, cellulose and lignin (Peters Citation2011; Pasangulapati et al. Citation2012; Burhenne et al. Citation2013; Stefanidis et al. Citation2014). The mass losses of biomass occur in three stages, the first elimination of moisture, the second portion of decomposition organic matter (cellulose and degradation of hemicellulose) the third dissociation of other elements present in the biomass. The cellulose and hemicellulose in biomass were playing an important role in combustion and pyrolysis. For producer gas generation kinetic analysis of CC and resources necessarily is required to understand its behaviour. Derivative thermogravimetric (DTG) is a technique that evaluates the variation of mass loss (or mass gain) with temperature. It is used for evaluation of kinetic parameters of agriculture residue. The thermal degradation behaviour and kinetics of biomass can be employed to understand the thermal behaviour and constituent of biomass degradation rate prior to gasification. Assessment of thermophysical characteristics of agricultural residues facilitate its utilisation and processing for syngas production in the gasifier. The thermophysical properties have prejudged the suitability of biomass for energy generation such as biofuel.
On the other hand, thermochemical conversion of biomass for producing biofuel comes with certain operational problems, such as ash deposits, slagging, fouling or corrosion, which reduce the thermal efficiency of boilers and gasifier (Yao et al. Citation2020). Due to the high contents of alkali and alkaline earth metals in biomass, the co-firing of biomass and coal has also encountered certain operational problems, such as ash slagging, fouling and corrosion (Yao et al. Citation2019; Yao et al. Citation2020; Yao et al. Citation2020; Yao et al. Citation2020). (Öhman et al. Citation2004) reported an agglomeration mechanism which was based on three different stages. Firstly, they reported that the deposition of ash coating (containing fine particles and alkali compounds) on the bed material through condensation and surface chemical reactions. Secondly, they mentioned that the sintering of the inner layer of the coating occurs, and lastly, the partial melting of the coating develops a sticky outer layer on the bed particle which results in agglomeration.
Different forms of biomass are reported in the literature, including biomass from the forest (wood, leaves, and bark), agricultural waste (corncob, millet cob, rice husk, and grasses), domestic waste, sanitary waste biomass and different types and energy crop. Corn is worldwide grown in about 160 countries on about 178 million hectares and contributes approximately 50% of global grain supply (1.170 million MT) (Abebe et al. Citation2016). In India, the maize accounts for approximately 9% of the total grains and third most essential food grain after rice (approx. 42%). Also, it accounts approximately 38% of total amount of cereals and barley produced (Abebe et al. Citation2016). A significant fraction of corn residue, around 0.70 kg of stover and 0.15 kg of cob for every kg of dried corn residue, is not usually utilised, although this either left as a waste or burning freely in its fields (Onsree et al. Citation2018). The need for the paper industry (e.g. paper pulp, wood, and fiber) is entirely fulfilled by eucalyptus, a tree with a high growth rate. There is a lot of eucalyptus residue produced during paper processing such as plant dust, leaves, bark, and chips. Aggregation and degradation of residues of eucalyptus may be a cause of environmental concerns (Chen et al. Citation2015). However, by the thermochemical conversion process, the corn and eucalyptus residues are converted into useful energy resources, not only can the residues be handled efficiently, but environmental emissions and energy insecurity can also be minimised in future.
Based on the above background, it is found that the agriculture residues namely Eucalyptus and Corncob are available in plenty. Thus, they can be used to produce the biofuel in future. In this context, the present study was carried out in order to identify the potential use corncob and eucalypts as biofuel in future. The main objectives of the present work were:
Determination of the thermal degradation properties of heating corncob (CC) and eucalyptus (EU) residues for gasification using thermogravimetric analysis (TGA) rates of 10°C/min in a nitrogen atmosphere. The study discusses the effect of heating rate on the composition and kinetic parameters of biomass.
Determination of the kinetic parameters of the corn cob (CC) and eucalyptus (EU) by using FWO (Flynn-wall-Ozawa) methods.
Determination of the kinetic deconvolution of biomass, combustion characteristics parameters from TGA measurement under inert gas (nitrogen) using weight loss of the samples with time.
Materials and methods
Preparation of biomass samples
Biomass sample was grinded in ball mill hammer and chopper machine, which was shown in . The proximate and ultimate analysis was shown in . The calorific value HHV (High heating value) and LHV (lower heating value) was determined by the help of Dulongs equation (Hosokai et al. Citation2016):
Where H, O, S and Ware hydrogen, oxygen, sulphur and water (hygroscopic moisture) contents (% by weight) of fuel, respectively.
Table 1. Characteristics of the biomass sample.
Experimental apparatus and procedures
The thermogravimetric analysis was carried using the method adopted by the investigator (Seo et al. Citation2010), with slight modification using a nitrogen environment having a flow rate of 200 ml/min. The wall temperature was measured by R-type thermocouple. The initial weight of the sample (10.52 mg) was loaded on the pan in the TGA heating zone. The range of a sample of biomass 0.001–2.000 gm has been used for the analysis in the thermogravimetric analysis. Initially, biomass samples were dried at 110°C for 30 min for pyrolysis achieved up to 1000°C. Whole methodology of the process was shown in and the detail of thermo-gravimetric analysis setup was represented in .
Results and discussion
Thermo-gravimetry analysis (TG and DTG)
Pyrolysis characteristics
In each biomass sample, the cellulose, hemicelluloses and lignin are the main component which has unique thermal degradation behaviour in the pyrolysis and combustion. The decomposition of these components in pyrolysis occurs at a different temperature. CC contains 40.3 wt.% cellulose, 28.6 wt.% hemicellulose, 16.6 wt.% lignin and EU contain 41.58 wt.% cellulose, 32.81 wt.% hemicellulose and 33.56 wt.% lignin. The joint thermo-gravimetry (TG) and differential thermo-gravimetry (DTG) thermograph of CC and EU were performed at 10°C min−1 heating rate shown in and . The detail of devolatilisation of CC and EU initiates at 250°C and 246°C, respectively are shown in and . In the second region of thermograph, a broad devolatilisation occurred due to the destruction of weak bond of polymeric structure which is present in the biomass and form strengthen bond (Pinto et al. Citation2017). In the devolatilisation step, two distinct peaks were observed in the DTG thermograph depicting the CC and EU of DTG at 275 and 336°C respectively. The probable pattern is due to the degradation of hemicelluloses and cellulose (315°C for CC and 474°C for EU).
Kinetic deconvolution of biomass
For CC and EU, this model is the best fit which is shown in and . It was fit for all biomasses. According to the profile of DTG, the devolatisation of CC and EU was modelled by deconvolution profiles i.e. three independent profiles of three different components namely hemicellulose, cellulose and lignin. From these profiles, the hemicellulose was found active at low temperature, cellulose was reactive at narrower temperature range, and the reactivity of lignin lie in a wider range of temperature between 400 and 1000°C.
Proximate and ultimate analysis
The thermo-gravimetry and differential thermo-gravimetry thermographs show the decomposition CC and EU represented in . In , first is the moisture release, secondly the devolatilisation and thirdly was the char degradation. The moisture, volatile matter, fixed carbon, and ash content of biomass were shown in . The different parameters of biomass such as heating value and proximate analysis of the CC and EU are in adequate coordination with the experimental result of CC and EU. From the proximate analysis data, it is marked that the CC ash content is less than that of the EU and heating value and volatile matter of the CC is higher than that of the EU which is shown in .
Table 2. Proximate analysis (wt% on air dry basis).
Moisture evolution profiles
The moisture contour shows that the CC degrades the smallest weight loss of 3.47% than EU has 5.12% weight loss. The moisture peak in the DTG contour of EU has 0.029 value at 70°C and for CC peak whose moisture peak occurred at 65°C. It shows that for removal of moisture in EU require more enthalpy than CC because in EU water molecule was tightly bound in the plant cell. The maximum loss of moisture occurred at 90°C for CC and 110°C for EU. The variation of temperature in the initial decomposition of biomass was related to the variation of the elemental and chemical bond structure of the cell.
Volatiles profiles
Volatile matter plays an important role in pyrolysis during thermal degradation of biomass. The reactivity of biomass sample depends on the rate of mass loss which is depicted by combined TG and DTG curve under the analysis. The volatile release depicts by thermograph of CC and EU occurred at 82% and 73.46%, respectively. The CC is more sensitive in devolatilisation compared to the EU. The first peak of CC occurred at −0.362 mg/min due to hemicellulose degradation after 30 min. The DTG peak of EU occurred at −0.11 mg/min which was lower than CC. The mass degraded up to 490°C was 90% for the CC and 85.12% for EU of total volatile matter present in the biomasses. The formation of char started after complete elimination of volatile matter from the biomass. The mass fraction of char degradation to the total mass of CC and EU is 3.81% and 6.6%, respectively which is summarised in .
Table 3. Mass loss summary of two materials.
Combustion characteristics
To depict the combustion characteristics of solid fuel, the burn out temperature has been broadly used by investigator (Miller Citation2011; Guangyin and Youcai Citation2017; Kiang Citation2018). Burnout temperature is the rate of weight loss of solid fuel steadily reduced to less than 1% per min. The burn out the temperature of CC and EU was 690°C and 544°C, respectively. The burnout temperature of CC is lower than EU means that CC has rapid combustion characteristics in comparison to EU.
Fourier transform infrared spectroscopy
The Fourier transform infrared spectroscopy (FTIR) was performed to classify the wood and to estimate the cellulose and hemicellulose and lignin (by functional group) present in the biomass by measuring the FTIR spectra in the 4000–500 cm−1 in the transmission mode. The FT-IR spectrum is shown in , and the functional groups found in CC and EU are listed in based on literature (Guimarães et al. Citation2009; Mothé and De Miranda Citation2009; Naik et al. Citation2010; Özçimen and Ersoy-Meriçboyu Citation2010; Akhtar, Goyal, and Goyal Citation2016). The highest percentage of transmittance was observed for a period of 3415–3626 cm−1, referring to the stretching deformation of O-H pairs, reflecting free water, and absorbed water is present in the wood eucalyptus and lesser water is found in corncob. The value for CC and EU occurs at 2887 and 2900 cm−1respectively it notes that the C–H stretching of the aliphatic system is present in the biomass components of hemicellulose, cellulose and lignin. Peaks at 1744 cm−1 for a CC and 1737 cm−1 for EU were induced by stretching hemicellulose. aromatic C=C ring stretching at 1608 and 1600 cm−1 for CC and EU, respectively. 1650 cm−1 for CC and 1642 cm−1 deformation of H–O–H Lignin or cellulose-associated water. The the peaks in this region confirms the the hemicellulose and celluloseis more in CC the EU the original biomass so CC is more effective pyrolysis than EU.
Table 4. The functional groups identified FTIR spectroscopy in the CC and EU.
Assessment of kinetic parameters
Kinetic parameter for eucalyptus, corncob
Kim and Liou et al (Seo et al. Citation2011) used to evaluate the pyrolysis kinetic parameters from the thermogravimetric data. The rate of mass conversion of biomass, dm/dt, in thermal decomposition was stated as:
(1)
(1) Rate constant k was expressed by the Arrhenius equation as:
(2)
(2) The temperature independent conversion, f (m) was expressed as:
(3)
(3) Equations (2) and (3) substitute in Equations (1) and taking natural logarithm both side, the first Equations (4) yield
(4)
(4) From Equations (4), the energy of activation energy (E) can be determined.
Activation energies analysis
The activation energy of EU and CC were analysed by FWO methods. The activation energies at different conversion for pyrolysis of corncob and Eucalypts were shown in and . The activation energy haphazardly increased for both biomass up to 60% and 50% for CC and EU respectively, it was due to cellulose and hemicellulose degradation. The average activation energy for EU was found 77.21 and 91.29 kJ/mol for CC. Hence, CC having higher thermochemical reactivity as compared to EU.
Table 5. Activation Energies (kJ/mol) for pyrolysis of EU, and CC.
Conclusions
The componential (cellulose, hemicellulose and lignin) behaviour in combustion and devolatisation of eucalyptus and corncob were analysed. The CC contains 40.3 wt.% of cellulose, 28.6 wt.% of hemicellulose, 16.6 wt.% of lignin however, EU contain 41.58 wt.% of cellulose, 32.81 wt.% of hemicellulose and 33.56 wt.% of lignin. The volatile matter of CC and EU was found as 82% and 73.46%, respectively. FTIR spectra confirmed the existence of hydroxyls, aldehydes, aromatics, olefins, ethers. The burn out temperature energy of corncob and eucalyptus were found as 690°C and 544°C, respectively whereas the average activation energy of corncob and eucalyptus was found as 91.29 and 77.21 kJ/mol, respectively. The results also depicts that pyrolysis rate depends on the cellulose and lignin content in the biomass. More the cellulose content results in faster pyrolysis rate whereas more the lignin content results in the lesser pyrolysis.
Disclosure statement
No potential conflict of interest was reported by the author(s).
References
- Abebe, A., H. Pathak, S. D. Singh, A. Bhatia, R. C. Harit, and V. Kumar. 2016. “Growth, yield and quality of maize with elevated atmospheric carbon dioxide and temperature in north–west India | Elsevier Enhanced Reader.” https://reader.elsevier.com/reader/sd/pii/S0167880915301493?token=D43BC56C8CC95E6AF67F962C78C4EB1BB28C6A2D48AD941F8AD96C8A3E86D6B951F45574BB8AE0A780B398614FEA32F1 (accessed Jan. 17, 2021).
- Akhtar, N., D. Goyal, and A. Goyal. May 2016. “Physico-chemical Characteristics of Leaf Litter Biomass to Delineate the Chemistries Involved in Biofuel Production.” Journal of the Taiwan Institute of Chemical Engineers. 62: 239–246. doi:https://doi.org/10.1016/j.jtice.2016.02.011.
- Bach, Q. V., and W. H. Chen. Dec. 01, 2017. “Pyrolysis Characteristics and Kinetics of Microalgae via Thermogravimetric Analysis (TGA): A State-of-the-art Review.” Bioresource Technology 246: 88–100. doi:https://doi.org/10.1016/j.biortech.2017.06.087.
- Bram, S., J. De Ruyck, and D. Lavric. Feb. 2009. “Using Biomass: A System Perturbation Analysis.” Applied Energy 86 (2): 194–201. doi:https://doi.org/10.1016/j.apenergy.2007.12.002.
- Burhenne, L., J. Messmer, T. Aicher, and M. P. Laborie. May 2013. “The Effect of the Biomass Components Lignin, Cellulose and Hemicellulose on TGA and Fixed bed Pyrolysis.” Journal of Analytical and Applied Pyrolysis 101: 177–184. doi:https://doi.org/10.1016/j.jaap.2013.01.012.
- Chen, Z., Q. Zhu, X. Wang, B. Xiao, and S. Liu. Aug. 2015. “Pyrolysis Behaviors and Kinetic Studies on Eucalyptus Residues Using Thermogravimetric Analysis.” Energy Convers. Manag. 105: 251–259. doi:https://doi.org/10.1016/j.enconman.2015.07.077.
- Demirbas, A. Jan. 01, 2004. “Combustion Characteristics of Different Biomass Fuels.” Progress in Energy and Combustion Science 30 (2): 219–230. doi:https://doi.org/10.1016/j.pecs.2003.10.004.
- Ferreira, C. I. A., V. Calisto, E. M. Cuerda-Correa, M. Otero, H. Nadais, and V. I. Esteves. Oct. 2016. “Comparative Valorisation of Agricultural and Industrial Biowastes by Combustion and Pyrolysis.” Bioresource Technology 218: 918–925. doi:https://doi.org/10.1016/j.biortech.2016.07.047.
- Guangyin, Z., and Z. Youcai. 2017. “Sewage Sludge Solidification/Stabilization and Drying/Incineration Process.” In Pollution Control and Resource Recovery, edited by Ken Mc Combs, 101–160. Kidlington, United Kingdom: Elsevier.
- Guimarães, J. L., E. Frollini, C. G. da Silva, F. Wypych, and K. G. Satyanarayana. Nov. 2009. “Characterization of Banana, Sugarcane Bagasse and Sponge Gourd Fibers of Brazil.” Industrial Crops and Products 30 (3): 407–415. doi:https://doi.org/10.1016/j.indcrop.2009.07.013.
- Hosokai, S., K. Matsuoka, K. Kuramoto, and Y. Suzuki. Nov. 2016. “Modification of Dulong’s Formula to Estimate Heating Value of gas, Liquid and Solid Fuels.” Fuel Processing Technology 152: 399–405. doi:https://doi.org/10.1016/j.fuproc.2016.06.040.
- Jaroenkhasemmeesuk, C., and N. Tippayawong. Nov. 2015. “Technical and Economic Analysis of A Biomass Pyrolysis Plant.” Energy Procedia 79: 950–955. doi:https://doi.org/10.1016/j.egypro.2015.11.592.
- Jayaraman, K., M. V. Kok, and I. Gokalp. Oct. 2017. “Pyrolysis, Combustion and Gasification Studies of Different Sized Coal Particles Using TGA-MS.” Applied Thermal Engineering 125: 1446–1455. doi:https://doi.org/10.1016/j.applthermaleng.2017.07.128.
- Jeguirim, M., S. Dorge, and G. Trouvé. Jan. 2010. “Thermogravimetric Analysis and Emission Characteristics of two Energy Crops in air Atmosphere: Arundo Donax and Miscanthus Giganthus.” Bioresource Technology 101 (2): 788–793. doi:https://doi.org/10.1016/j.biortech.2009.05.063.
- Jeguirim, M., and G. Trouvé. Sep. 2009. “Pyrolysis Characteristics and Kinetics of Arundo Donax Using Thermogravimetric Analysis.” Bioresource Technology 100 (17): 4026–4031. doi:https://doi.org/10.1016/j.biortech.2009.03.033.
- Kiang, Y.-H. 2018. “Combustion Process Calculation for Fuels, Biomass, Wastes, Biosludge, and Biocarbons.” In Fuel Property Estimation and Combustion Process Characterization, edited by Raquel Zanol, 235–264. Londan, United Kingdom: Elsevier.
- Miller, B. G. 2011. “Clean Coal Technologies for Advanced Power Generation.” In Clean Coal Engineering Technology, edited by Sarah Hughes, 251–300. Kidlington, United Kingdom: Elsevier.
- Mothé, C. G., and I. C. De Miranda. Aug. 2009. “Characterization of Sugarcane and Coconut Fibers by Thermal Analysis and FTIR.” Journal of Thermal Analysis and Calorimetry 97 (2): 661–665. doi:https://doi.org/10.1007/s10973-009-0346-3.
- Naik, S., V. V. Goud, P. K. Rout, K. Jacobson, and A. K. Dalai. Aug. 2010. “Characterization of Canadian Biomass for Alternative Renewable Biofuel.” Renewable Energy 35 (8): 1624–1631. doi:https://doi.org/10.1016/j.renene.2009.08.033.
- Naqvi, S. R., S. Jamshaid, M. Naqvi, W. Farooq, M. B. K. Niazi, Z. Aman, M. Zubair, et al. Jan. 01, 2018. “Potential of Biomass for Bioenergy in Pakistan Based on Present Case and Future Perspectives.” Renewable and Sustainable Energy Reviews 81: 1247–1258. doi:https://doi.org/10.1016/j.rser.2017.08.012.
- Öhman, M., A. Nordin, H. Hedman, and R. Jirjis. Dec. 2004. “Reasons for Slagging During Stemwood Pellet Combustion and Some Measures for Prevention.” Biomass and Bioenergy 27 (6): 597–605. doi:https://doi.org/10.1016/j.biombioe.2003.08.017.
- Onsree, T., N. Tippayawong, A. Zheng, and H. Li. Sep. 2018. “Pyrolysis Behavior and Kinetics of Corn Residue Pellets and Eucalyptus Wood Chips in a Macro Thermogravimetric Analyzer.” Case Stud. Therm. Eng. 12: 546–556. doi:https://doi.org/10.1016/j.csite.2018.07.011.
- Özçimen, D., and A. Ersoy-Meriçboyu. Jun. 2010. “Characterization of Biochar and bio-oil Samples Obtained from Carbonization of Various Biomass Materials.” Renewable Energy 35 (6): 1319–1324. doi:https://doi.org/10.1016/j.renene.2009.11.042.
- Pasangulapati, V., K. D. Ramachandriya, A. Kumar, M. R. Wilkins, C. L. Jones, and R. L. Huhnke. Jun. 2012. “Effects of Cellulose, Hemicellulose and Lignin on Thermochemical Conversion Characteristics of the Selected Biomass.” Bioresource Technology 114: 663–669. doi:https://doi.org/10.1016/j.biortech.2012.03.036.
- Peters, B. Oct. 2011. “Prediction of Pyrolysis of Pistachio Shells Based on its Components Hemicellulose, Cellulose and Lignin.” Fuel Processing Technology 92 (10): 1993–1998. doi:https://doi.org/10.1016/j.fuproc.2011.05.023.
- Pinto, F., J. Gominho, R. N. André, D. Gonçalves, M. Miranda, F. Varela, D. Neves, J. Santos, A. Lourenço, and H. Pereira. Oct. 2017. “Improvement of Gasification Performance of Eucalyptus Globulus Stumps with Torrefaction and Densification pre-Treatments.” Fuel 206: 289–299. doi:https://doi.org/10.1016/j.fuel.2017.06.008.
- Punnarapong, P., T. Sucharitakul, and N. Tippayawong. Mar. 2017. “Performance Evaluation of Premixed Burner Fueled with Biomass Derived Producer gas.” Case Stud. Therm. Eng. 9: 40–46. doi:https://doi.org/10.1016/j.csite.2016.12.001.
- Seo, D. K., S. S. Park, J. Hwang, and T. U. Yu. Sep. 2010. “Study of the Pyrolysis of Biomass Using Thermo-Gravimetric Analysis (TGA) and Concentration Measurements of the Evolved Species.” Journal of Analytical and Applied Pyrolysis 89 (1): 66–73. doi:https://doi.org/10.1016/j.jaap.2010.05.008.
- Seo, D. K., S. S. Park, Y. T. Kim, J. Hwang, and T. U. Yu. 2011 Sep 1. “Study of Coal Pyrolysis by Thermo-Gravimetric Analysis (TGA) and Concentration Measurements of the Evolved Species.” Journal of Analytical and Applied Pyrolysis 92 (1): 209–216. [Online]. Available: https://www.sciencedirect.com/science/article/pii/S0165237011001057.
- Stefanidis, S. D., K. G. Kalogiannis, E. F. Iliopoulou, C. M. Michailof, P. A. Pilavachi, and A. A. Lappas. Jan. 2014. “A Study of Lignocellulosic Biomass Pyrolysis via the Pyrolysis of Cellulose, Hemicellulose and Lignin.” Journal of Analytical and Applied Pyrolysis 105: 143–150. doi:https://doi.org/10.1016/j.jaap.2013.10.013.
- Tippayawong, N., C. Chaichana, A. Promwungkwa, and P. Rerkkriangkrai. Mar. 2013. “Investigation of a Small Biomass Gasifier–Engine System Operation and Its Application to Water Pumping in Rural Thailand.” Energy Sources, Part A Recover. Util. Environ. Eff. 35 (5): 476–486. doi:https://doi.org/10.1080/15567036.2010.511433.
- White, J. E., W. J. Catallo, and B. L. Legendre. May 01, 2011. “Biomass Pyrolysis Kinetics: A Comparative Critical Review with Relevant Agricultural Residue Case Studies.” Journal of Analytical and Applied Pyrolysis 91 (1): 1–33. doi:https://doi.org/10.1016/j.jaap.2011.01.004.
- Wilk, M., A. Magdziarz, I. Kalemba, and P. Gara. Jan. 2016. “Carbonisation of Wood Residue Into Charcoal During low Temperature Process.” Renewable Energy 85: 507–513. doi:https://doi.org/10.1016/j.renene.2015.06.072.
- Wilson, L., W. Yang, W. Blasiak, G. R. John, and C. F. Mhilu. Jan. 2011. “Thermal Characterization of Tropical Biomass Feedstocks.” Energy Conversion and Management 52 (1): 191–198. doi:https://doi.org/10.1016/j.enconman.2010.06.058.
- Yao, X., Y. Hu, J. Ge, X. Ma, J. Mao, L. Sun, K. Xu, and K. Xu. Sep. 2020. “A Comprehensive Study on Influence of Operating Parameters on Agglomeration of Ashes During Biomass Gasification in a Laboratory-Scale Gasification System.” Fuel 276: 118083. doi:https://doi.org/10.1016/j.fuel.2020.118083.
- Yao, X., Z. Zhao, J. Li, B. Zhang, H. Zhou, and K. Xu. May 2020. “Experimental Investigation of Physicochemical and Slagging Characteristics of Inorganic Constituents in ash Residues from Gasification of Different Herbaceous Biomass.” Energy 198: 117367. doi:https://doi.org/10.1016/j.energy.2020.117367.
- Yao, X., H. Zhou, K. Xu, S. Chen, J. Ge, and Q. Xu. Mar. 2020. “Systematic Study on ash Transformation Behaviour and Thermal Kinetic Characteristics During co-Firing of Biomass with High Ratios of Bituminous Coal.” Renewable Energy 147: 1453–1468. doi:https://doi.org/10.1016/j.renene.2019.09.103.
- Yao, X., H. Zhou, K. Xu, Q. Xu, and L. Li. Jan. 2020. “Investigation on the Fusion Characterization and Melting Kinetics of Ashes from co-Firing of Anthracite and Pine Sawdust.” Renewable Energy 145: 835–846. doi:https://doi.org/10.1016/j.renene.2019.06.087.
- Yao, X., H. Zhou, K. Xu, Q. Xu, and L. Li. Nov. 2019. “Evaluation of the Fusion and Agglomeration Properties of Ashes from Combustion of Biomass, Coal and Their Mixtures and the Effects of K2CO3 Additives.” Fuel 255: 115829. doi:https://doi.org/10.1016/j.fuel.2019.115829.
- Zhang, L., and C. Xu. 2021. “Conversion and management, and undefined 2010, Overview of recent advances in thermo-chemical conversion of biomass,” Elsevier, Accessed: Jan. 12, 2021. [Online]. Available: https://www.sciencedirect.com/science/article/pii/S0196890409004889.