ABSTRACT
Radiative cooling paints offer a sustainable method for reducing energy demand in buildings. This work investigates the effects of particle volume concentration (PVC) of calcium carbonate (CaCO3) and hollow silicon dioxide (SiO2) microparticles in acrylic-based paints on cooling capability. Paint solar reflectance increases as the total PVC increases until a peak PVC is reached. The addition of CaCO3 improves solar reflectance due to an enhancement in near-infrared reflection but decreases thermal emission. The paint, with a total PVC of 0.45 and a CaCO3:SiO2 PVC ratio of 1:1, offers the right balance between solar reflection and thermal emissivity and achieves the best cooling performance. This work demonstrates that the CaCO3-SiO2 paint system allows tuning of the radiative cooling performance.
1. Introduction
Space cooling is a significant part of the global energy demand. In 2020, 16% of the total worldwide electricity consumption was used for cooling in buildings alone (International Energy Agency Citation2021). As the contemporary climate change accelerates a rise in the average global temperature, the demand for this energy usage will further increase in a near future. Without meaningful improvements in cooling technologies, it is projected that energy globally used for cooling will surpass that for heating by 2060 (Isaac and van Vuuren Citation2009). Especially in southeast Asian countries, the electricity demand from cooling sectors is predicted to increase by 200% by 2040 (International Energy Agency Citation2019). Radiative cooling, a long-discovered passive cooling mechanism, is one of the key technologies that can provide sustainable solutions to this global problem. Two mechanisms responsible for radiative cooling phenomena are reflection of incident solar irradiation and emission of thermal radiation in the infrared region. A surface’s temperature decreases as long as heat loss by thermal radiation exceeds heat received from absorbed sunlight and other sources. Therefore, structures with high solar reflectance and high infrared emissivity are preferred for implementing radiative cooling technology.
Solar irradiation is an important source of heat that affects the temperature of any exposed surface. While most of the intensity of the sunlight is from photons in the visible region, ultraviolet (UV) and near-infrared (NIR) photons also have a meaningful contribution to solar irradiation. While specular reflectance can be used for sunlight rejection (Wang et al. Citation2021; Mourou et al. Citation2022), most research on radiative cooling employs diffuse reflection for this purpose (Li et al. Citation2021; Zhai Citation2017; Mandal Citation2018; Chae et al. Citation2021; Li Citation2019). Diffuse reflection generally occurs in media with contrast in the refractive indices of their components and with a degree of disorder that causes incident light to be scattered many times within the structure. Paints that consist of pigment and binders are a simple way to achieve light scattering, since the arrangement of pigment in paints is naturally disordered.
While solar reflection leads to a reduction in the heat absorption of the paints, thermal emission is the primary mechanism responsible for heat loss. Particularly, thermal emission in a wavelength range of 8–13 µm is very effective for cooling below ambient (Wijewardane and Goswami Citation2012; Zhao Citation2019; Hossain and Gu Citation2016), because atmospheric transparency in this range allows heat exchange between cooling structures and extremely cold deep space. High emissivity outside of this wavelength is often detrimental to cooling performance if the temperature of the paint is below the atmosphere’s temperature. Therefore, selective emitters with very high emissivity only in the 8–13 µm range are highly desired in radiative cooling research. Nevertheless, the transparency of the sky is affected by local weather conditions and climate regions (Karn, Chintala, and Kumar Citation2019; Centeno Citation1982). For example, high precipitable water in the atmosphere of tropical regions reduces the transparency significantly (Karn, Chintala, and Kumar Citation2019; Hanif et al. Citation2014; Al-Obaidi, Ismail, and Abdul Rahman Citation2014) and hinders applications of sub-ambient radiative cooling. Consequently, in such regions, above-ambient cooling is a more feasible deployment of radiative cooling and can still reduce energy consumption used for heat management. At a paint temperature above ambient, the atmosphere serves as a heat sink, and thus paints with strong broadband emission, with high emissivity from 2.5 to 25 µm, are preferred. Thermal emissivity, a ratio of the total thermal emission from a surface to that from a blackbody, can be used to quantify this broadband emission. Therefore, this work explores above-ambient radiative cooling paints with high thermal emissivity.
Since a seminal work by Raman et al. (Citation2014), various materials, from nanostructures (Zhu, Raman, and Fan Citation2015; Lu Citation2017; Rephaeli, Raman, and Fan Citation2013) to bulk building components (Li Citation2019), have been successfully engineered to have strong cooling capability. For their versatility and scalability, pigmented paints remain a widely used and often commercialised form of radiative cooling technology in buildings. Pigment and binders are essential components of paints and are responsible for the strong solar reflection and thermal emissivity of the cooling paints. Pigment types and particle volume concentration (PVC) dictate paint optical properties. In coatings or materials that employ a solar-reflective silver layer (Zhai Citation2017; Xinyuan et al. Citation2022), particles are incorporated at a low PVC value, typically lower than 0.1, to enhance infrared emission. Pigmented paints, on the other hand, are typically coated on surfaces with low solar reflectance, and thus pigments must be included at higher PVC values (Li et al. Citation2021; Tao et al. Citation2021) to provide both strong solar reflection and thermal emission. The pigment that has been selected for radiative cooling paints includes titanium dioxide (TiO2) (Harrison and Walton Citation1978; Orel, Gunde, and Krainer Citation1993), silicon dioxide (SiO2) (Gentle and Smith Citation2010; Zhang Citation2017), calcium carbonate (CaCO3) (Tao et al. Citation2021; Li Citation2020), barium sulfate (BaSO4) (Tong Citation2022; Li et al. Citation2020), organic polymer (Alden et al. Citation2019), and hollow/core–shell particles (Huang, Li, and Fan Citation2021; Atiganyanun Citation2021). These selections of pigment offer different benefits and disadvantages. While TiO2 pigment is the most popular choice for commercialised cooling paints due to its high refractive index, its strong UV absorption reduces cooling performance. Both SiO2 and BaSO4 have strong emission in the atmospheric window region but often require high PVC to achieve high solar reflectance. CaCO3 pigment is a popular and inexpensive paint filler that can yield high solar reflectance but has weak thermal emission. The optical properties of organic polymer pigment and hollow/core–shell particles can be controlled by synthesis techniques, which in turn increase material costs. The main function of binders in paints is to form a continuous matrix that holds pigment in place. Nevertheless, binder optical properties are important to radiative cooling paints since the volume of binders in paints is comparable to, if not greater than, the pigment volume. The most common binder in cooling paints is acrylic resin, because of its versatility, toughness, suitable optical properties, and price. Specialty polymers such as fluorinated binders (Fan, Sun, and Li Citation2019) can also be used to further improve NIR reflectance.
In most research on pigmented radiative cooling paints, only one type of pigment is included in the paint formulation. However, two-pigment paint systems can potentially allow fine tuning of the paint’s optical properties and provide a balance between the advantages and disadvantages of each pigment. Indeed, commercially available cooling paints often include two or more types of pigment (Chaiyosburana et al. Citation2013), but there is relatively less research on two-pigment paint formulations for radiative cooling applications. Orel, Gunde, and Krainer (Citation1993) studied the use of TiO2 and BaSO4 pigment in radiative cooling paints and found that adding BaSO4 increased the thermal emissivity of the paints, while TiO2 provided the high solar reflectance. Gentle and Smith (Citation2010) found that SiO2 and silicon carbide nanoparticle pigment acted as complementary emitters in the wavelength ranges of 8–10 and 10.5–13 µm, respectively. The addition of both particles allowed full thermal emission through the atmospheric window. Despite significant work in two-pigment paint systems, gaps in knowledge in the formulation of radiative cooling paints with multiple pigment types remain. This is because multiple-pigment type formulations have significantly more formulation parameters to consider than single-pigment systems.
In this work, we explore acrylic-based paints that incorporate both CaCO3 and hollow SiO2 microparticles. The CaCO3 microparticles are added to increase the solar reflectance of the paint samples, while the hollow SiO2 microparticles are used for improving broadband thermal emission. The hollow particles were selected instead of solid SiO2 microparticles, because they were found to provide better cooling performance (Atiganyanun Citation2021). The effects of the total PVC and the ratio between the CaCO3 and the hollow SiO2 microparticles on solar reflectance and thermal emissivity are investigated. Paint samples are tested in a daytime outdoor setup, and their above-ambient cooling capability is measured and compared. Both the optical characterisation and the field test provide a guideline for tuning the radiative cooling performance via PVC adjustment in the two-pigment paint formulation.
2. Materials and methods
2.1. Synthesis of microparticles
CaCO3 microparticles are fabricated via an ionic-exchange, solution method described in a previous work (Febrida Citation2021) with minor adjustments. Sodium carbonate (Na2CO3) and calcium nitrate tetrahydrate (Ca(NO3)2·4H2O), both analytical grade, were purchased from KemAus. 50 ml of a 0.5M Na2CO3 aqueous solution was added to 50 ml of a 0.5M Ca(NO3)2·4H2O aqueous solution under a stirring speed of 1000 rpm and at a temperature of 25°C. The reaction time was 30 min, and the product was then filtered with a Whatman filter paper (No.1) placed in a Buchner funnel. The precipitate was washed with deionised water and ethanol at least four times and then dried in the open air at 50°C for 24 h.
Hollow SiO2 microparticles are synthesised by a modified method adapted from two previous works (Lei Citation2014; Zhang Citation2009). The method consisted of the formation of solid SiO2 particles via the Stöber sol–gel process and the etching of SiO2 to induce an air gap inside the microparticles. In the formation step, a mixture of 0.013 g of potassium chloride (KCl) (analytical grade, KemAus), 14.1 ml of deionised water, 52.92 ml of absolute ethanol (Merck), and 7.94 ml of a 28% ammonium hydroxide solution (Merck) was prepared and added to a glass reactor. Inside the reactor, a stirring rate and a temperature were maintained at 250 rpm and 35°C, respectively. Another solution containing 2.1 ml of tetraethyl orthosilicate (TEOS) (98%, Sigma-Aldrich) and 30.86 ml of absolute ethanol was separately prepared. A batch of 5 ml of the TEOS-containing solution was gently poured into the reactor every 30 min, for a total of 7 batches. Then the reaction continued for another 24 h. The precipitate was washed in ethanol and deionised water several times and then dried in the open air at 50°C for 24 h. In the etching step, 3 g of the solid SiO2 microparticles were ground by a mortar and pestle and dispersed in a solution containing 2.5 g of polyvinylpyrrolidone (K-30, Sigma-Aldrich) and 100 ml of deionised water. The suspension was stirred at 250 rpm and heated to 45°C. 6 g of sodium borohydride (NaBH4) (98%, Loba Chemie) was added to the suspension, and the reaction proceeded for 24 h. The white precipitate was washed in deionised water until the suspension was at a neutral pH and dried in the open air at 50°C for 24 h.
2.2. Pigment characterisation
A scanning electron microscope (SEM), Hitachi S-3400N, with an accelerating voltage of 5 kV, was used to study the morphology of the CaCO3 and the hollow SiO2 pigment. A liquid pycnometer was used to determine the density of the pigment and the volume ratio of the air gap in the hollow microparticles. Water, ethanol, and dichloromethane were used as solvents in determining the density of the hollow pigment to investigate possible liquid infiltration into the particles. The crystal structures of the CaCO3 pigment were characterised by X-ray diffraction (XRD; Rigaku TTRAX III).
2.3. Paint formulation
The components of the white paint samples in this study were the CaCO3 and the hollow SiO2 microparticles as pigment, and an acrylic-based emulsion (SETAQUA 6160, Allnex) as both a binder and a solvent. Volume ratios of the CaCO3 and the hollow SiO2 pigment and total pigment volume concentrations were varied to study the influence of the pigment usage on the optical properties of the paint samples. The total PVC of the samples ranged from 20 to 55 percent. This range was below a critical PVC value and was chosen to prevent the formation of void volume between the pigment and the binder. A commercial paint shaker was used to homogenise the paint samples. The white paints were applied onto square iron plates with a thickness of 1 mm and a width of 5 cm. All the dried paints had a thickness of ∼100 μm.
2.4. Optical characterisation of paints
The total reflectance of the paint samples was measured with a spectrophotometer (Cary 5000, Agilent) and an integrating sphere, with a spectral range of 0.2 to 2.5 µm. The reflectance spectra and A.M. 1.5 solar irradiance standard were used to calculate solar-average reflectance. An emissometer (Model AE1, Devices and Services Co.) was used to measure the thermal emissivity of the paints.
2.5. Outdoor testing for radiative cooling performance
An outdoor testing setup was built to study the cooling performance of the samples. A sample chamber, located at the top of the setup, was made of aluminum composite. The chamber was coated with a white paint to minimise the absorption of sunlight. The four vertical sides of this chamber consisted of several double-louvre slats that blocked sunlight but allowed heat convection. A top cover of the chamber had four 5-cm-wide square openings so that sunlight could reach the samples and thermal radiation from the paint could be emitted toward the sky. This construction ensured that only the top surface of the samples was exposed to solar irradiation. Inside the sample chamber, four transparent polycarbonate platforms were erected to hold the samples close to the openings of the top cover. A K-type thermocouple was attached to the bottom of each sample and connected to a temperature reader. To prevent hot air from the ground from reaching the samples, the distance between the samples and the ground was set to 1.6 m. A pyranometer (SMP21, Kipp & Zonen) was placed adjacent to the sample chamber to measure solar irradiance intensity. A weather station was also installed near the sample chamber to record ambient temperature and humidity during the experiment. The setup was placed on a rooftop to maximise sunlight exposure during the day. With the setup, the outdoor cooling performance of the samples was tested for 12 h in November at Pathum Thani, Thailand.
3. Results and discussions
The SEM images of the microparticles, shown in , indicate that the CaCO3 particles (a) have an average size of 3.4 ± 0.3 µm, as measured by the ImageJ programme, and their shape is polyhedral. Each CaCO3 particle appears to consist of smaller cuboids whose sizes range from 0.5 to 2.0 µm. The XRD pattern, shown in Figure S1, indicates that the CaCO3 particles are calcite. However, because crystallinity has little impact on the refractive index of CaCO3 in the solar wavelength range, the crystallinity of the CaCO3 particles does not affect solar reflectance and is not investigated further in this study. On the other hand, particle size and size distribution have a strong influence on the light scattering of the particles. According to Mie theory (Mie Citation1908; Wriedt Citation2012), incident light can be effectively scattered by a particle if its characteristic size is close to the wavelength of the light. The scattering of light by a group of particles follows this principle, though other factors such as particle arrangement also have a significant impact on the scattering properties. The synthesised CaCO3 microparticles have two characteristic sizes: primary and secondary, which correspond to the overall shape of the particles and their crystalline cuboid components, respectively. Thus, it is surmised that CaCO3 microparticles can effectively scatter light in the solar region, especially in its longer wavelength part. The CaCO3 particles also have a coefficient of variation of 9%, indicating a moderate degree of polydispersity. While a broad particle size distribution can decrease the efficiency of light scattering at a wavelength corresponding to the average particle size, it can also improve light scattering at wavelengths that are mismatched with the average size (Aragón and Pecora Citation1976). Because the solar irradiation spectrum ranges from ultraviolet to near-infrared light, particles with some degree of polydispersity can scatter sunlight more efficiently when averaging over the whole solar spectrum (Li Citation2020; Peoples Citation2019). Therefore, the CaCO3 particles as synthesised are expected to have a strong solar rejection capability.
Figure 1. SEM images of (a) the solid calcium carbonate and (b) the hollow silica microparticles. The CaCO3 microparticles are constituted by smaller, box-shaped crystalline components and have an average size of 3.4 ± 0.3 µm. The hollow SiO2 microparticles are spherical and uniform with an average size of 1.11 ± 0.04 µm. The scales of both images correspond to 10 µm.
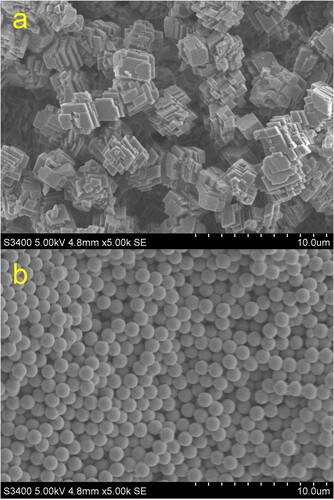
The hollow SiO2 microparticles are spherical and have an average diameter of 1.11 ± 0.04 µm. Unlike the CaCO3 particles, the hollow SiO2 pigment is smooth and has no secondary components. Because its average size is within the visible light spectrum and its size distribution is slightly polydisperse, the SiO2 pigment should be beneficial for solar reflection when deployed in a paint. However, due to the lower contrast in refractive index of SiO2 (∼1.46) and acrylic (∼1.49) than that of CaCO3 (∼1.59) and acrylic, the CaCO3 microparticles might be more suitable in this regard. Density measurement with a pycnometer indicates that the particle void volume fraction of SiO2 is 0.4. The particle density does not change when measured with different solvents, implying that liquid does not infiltrate into the air gap. The purpose of the air gap is to increase the refractive index contrast between pigment and binder to improve the scattering of solar irradiation. Additionally, the air volume decreases the effective refractive index in the mid-IR region, reduces internal reflection of the radiation in this wavelength range, and potentially enhances the thermal emission of SiO2 and acrylic, which is already high. Therefore, the use of hollow SiO2 microparticles as paint pigment is projected to improve both the scattering of sunlight and the thermal emission of the paint.
Pigment volume concentration has a significant impact on the optical properties of the paint. By averaging the measured reflectance spectra with A.M. 1.5 solar irradiation, the solar-average reflectance of the paint samples with different total PVCs and ratios of the CaCO3 and the hollow SiO2 PVC is calculated and displayed on . In general, an increase in the total PVC improves solar reflectance, until at a certain PVC value where solar reflectance begins to decrease. This is a well-known phenomenon, the optical crowding effect (Diebold Citation2014), where multiple light scattering sites become very close to one another and act as a single scattering site, effectively reducing scattering cross section. At the peak PVC value, just before the set-in of the optical crowding effect, the solar reflectance is maximised. The peak PVC value depends on various factors, such as paint composition. According to , paints with the CaCO3:SiO2 PVC ratio of 1:0, 3:1, and 1:3 have a peak PVC of ∼0.5, while the 1:1 paint has a peak PVC of ∼0.45, and the 0:1 paint has a peak PVC greater than or equal to 0.5. These peak PVC values indicate a paint formulation that would yield the highest solar reflectance for a fixed value of the PVC ratio.
Figure 2. Solar-average reflectance, as a function of a total particle volume concentration, of the paint samples with the ratio between CaCO3 and SiO2 PVC of 1:0 (black solid squares), 3:1 (blue circles), 1:1 (green triangles), 1:3 (orange stars), and 0:1 (red hollow squares). An increase in the concentration of CaCO3 generally improves solar reflectance. Solar reflectance also increases as the total PVC increases, up to a critical total PVC. The critical total PVC is dependent on the PVC ratio.
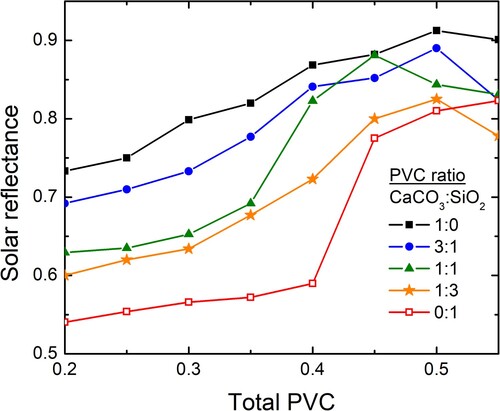
By comparing solar reflectance as a function of PVC ratios at a fixed total PVC, it is observed that the addition of the solid CaCO3 pigment is more beneficial to solar reflectance than the addition of the hollow SiO2 pigment. Two notable exceptions occur in the 1:1 formulation at the total PVC of 0.45, which is the peak PVC, and in the 0:1 formulation at the total PVC of 0.55. In the first case, the 1:1 paint formulation achieves solar reflectance of 0.881, which is very close to the solar reflectance (0.882) of the 1:0 formulation at the same total PVC. The second case also occurs near the peak PVC of the 0:1 formulation. These two exceptions show that formulating paints at the peak PVC might be able to increase solar reflectance beyond a value that is extrapolated solely from the relationship between PVC ratios and solar reflectance. This suggests that the peak PVC should be searched for during the formulation of solar reflective paints to maximise solar reflectance and to potentially reduce the amount of pigment needed for achieving high reflectance.
To elucidate how the CaCO3:SiO2 PVC ratios affect solar reflectance, the measured reflectance spectra of the paint samples with CaCO3:SiO2 PVC ratios of 3:1, 1:1, and 1:3 are displayed on . Higher CaCO3 PVC leads to a significant increase in reflectance in the near infrared region. This is due to the high refractive index of CaCO3 and the CaCO3 particle size, which is close to the wavelength. CaCO3 also improves the reflectance of the paints in the visible wavelength region, thanks to the CaCO3 particle’s secondary size. At a wavelength range of 700 to 450 nm, the reflectance of the 1:3 CaCO3:SiO2 paint increases and surpasses that of the 3:1 CaCO3:SiO2 paint at a wavelength of 500 nm. This indicates that the hollow SiO2 microparticles are more efficient at scattering light in this short wavelength range. However, at a wavelength below 450 nm, the reflectance of all three samples drops sharply due to the intrinsic UV light absorption of acrylic. This implies that improvement in UV light scattering by the hollow SiO2 is limited by this absorption. Thus, the CaCO3 and the hollow SiO2 microparticles are more suitable for improving reflectance in the near infrared-visible and the visible-UV regions, respectively, than vice versa. Nevertheless, according to , the overall enhancement of solar reflectance by the addition of CaCO3 appears to be superior to that of hollow SiO2.
Figure 3. Solar reflectance of the paint samples with a total PVC of 0.5 and CaCO3:SiO2 PVC ratios of 3:1 (solid blue line), 1:1 (dotted green line), and 1:3 (dashed orange line). The addition of the CaCO3 microparticles significantly enhances reflectance in the infrared region. The 1:3 CaCO3:SiO2 formulation shows a slight improvement in reflectance at a wavelength of ∼460 nm. Below a wavelength of 450nm, reflectance of the paints sharply decreases due to the intrinsic UV absorption of the acrylic binder.
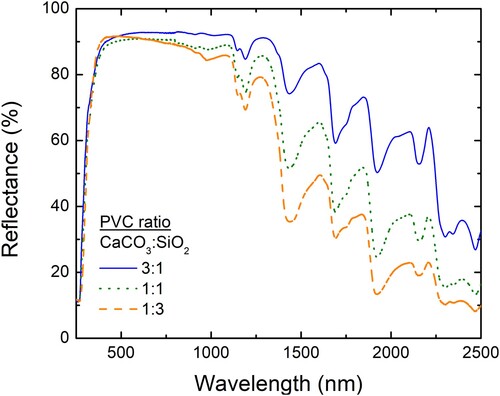
The solar reflectance of the hollow SiO2 microparticles could also be further improved by adjusting the size of the particles and the void volume. In other works that explore porous structures (Ma Citation2021; Hsu Citation2016; Huang Citation2022) for radiative cooling, an increase in the size of the pores embedded in a polymer matrix can be employed to enhance near-infrared reflectance. While introducing pores into a binder or between a binder and pigment is not recommended for pigment paints due to its negative impact on substrate adhesion, the void volume inside hollow pigment can be adjusted. In our previous work (Atiganyanun Citation2021), we varied the size of the hollow particles, while maintaining the void fraction. It was observed that an increase in the size of the hollow particles and thus the size of the void volume can improve solar reflection until the size exceeds a threshold. Beyond this size threshold, the solid shell of the hollow particles becomes too thick and disrupts efficient light scattering, which would occur between the void volume and other paint components. To get the most out of the void volume, like in those porous systems, an increase in the void volume should be accomplished without increasing the solid shell thickness. However, to incorporate such particles in liquid paints, the solid shell should still be able to prevent infiltration of binders, solvents, and other paint components into the hollow pigment.
Infrared irradiation is a mechanism that allows heat exchange between paints and the environment. Most research in radiative cooling focuses on achieving selectively high emissivity in the wavelength range of 8–13 µm for sub-ambient cooling. On the contrary, this work aims to achieve high broadband emissivity, i.e. thermal emissivity, which is suitable for above-ambient cooling. In this case, the atmosphere serves as a heat sink, and high thermal emissivity indicates efficient heat loss from the surface to the sky. The measured thermal emissivity of the paints is shown in . According to the thermal emissivity of the 0:1 CaCO3:SiO2 paint, the addition of hollow SiO2 at the expense of acrylic resin is beneficial to thermal emissivity. This is due to two reasons. First, at a wavelength of 9.5–9.8 µm where the thermal radiation of a blackbody at a room temperature is strongest, the extinction coefficient of SiO2 is ∼1.0 (Kitamura, Pilon, and Jonasz Citation2007), whereas that of acrylic is less than 0.1 (Jitian and Bratu Citation2012). High extinction coefficients confer high absorptivity. By Kirchhoff’s law of thermal radiation, absorptivity is equal to emissivity at a thermal equilibrium. Second, the void volume of the SiO2 microparticles reduces the internal reflection of the infrared irradiation by reducing the effective refractive index of the coating in the IR region. Conversely, according to the thermal emissivity of the 1:0 CaCO3:SiO2 paint, the addition of the CaCO3 microparticles at the expense of acrylic resin reduces the paint’s thermal emissivity. While CaCO3 has a small, similar extinction coefficient to that of acrylic in the wavelength range of 9.5–9.8 µm, CaCO3 has a greater refractive index (∼1.9) (Jarzembski et al. Citation2003) than acrylic (∼1.56) (Jitian and Bratu Citation2012) at this wavelength. This leads to greater internal reflection of IR emission and less thermal emissivity when CaCO3 is added.
Figure 4. Thermal emissivity, as a function of total particle volume concentration, of paint samples with ratios between CaCO3 and SiO2 PVC of 1:0 (black solid squares), 3:1 (blue circles), 1:1 (green triangles), 1:3 (orange stars), and 0:1 (red hollow squares). The addition of the CaCO3 microparticles alone reduces the thermal emissivity, while the addition of the hollow SiO2 improves it and counteracts the negative effect of the CaCO3 addition. Increasing the SiO2 concentration above the 1:3 CaCO3:SiO2 formulation has a minimal impact on the thermal emissivity.
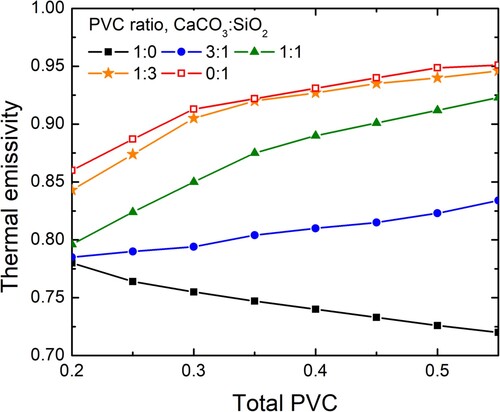
According to , at a fixed total PVC value, an increase in the hollow SiO2 PVC (while decreasing the CaCO3 PVC) improves paint thermal emissivity. This trend agrees with the previous comparison of SiO2, acrylic, and CaCO3 optical constants. However, the improvement in thermal emission is minimal when the hollow SiO2 PVC ratio increases from 1:3 (75%) to 0:1 (100%) at a fixed total PVC. We surmise that this is related to a very high refractive index (∼2.9) (Kitamura, Pilon, and Jonasz Citation2007) of SiO2 at a wavelength of 9.5–9.8 µm. As more SiO2 microparticles are added, internal reflection due to the very high refractive index becomes more significant. While the void volume in the SiO2 pigment helps reduce internal reflection, the CaCO3 pigment, which has a significantly lower refractive index than SiO2, can lower internal reflection as well. This benefit of the CaCO3 microparticles may lessen the impact of adding the hollow SiO2 on improving thermal emissivity when the SiO2 PVC ratio is above 75%.
From the optical data, the addition of the solid CaCO3 microparticles and the increase in the total PVC up to the peak PVC improve solar reflectance. At the same time, an increase in the total PVC and the hollow SiO2 PVC enhances thermal emission. This suggests that the most suitable formulation for cooling paints should have a high total PVC. The remaining question is at what CaCO3:SiO2 PVC ratio and total PVC the paint should be formulated for the best cooling performance. In consideration of its radiative cooling properties, paint performance in the harshest environment should be prioritised since it is a condition where cooling is most needed. Therefore, a case where sunlight is strong is considered. In this case, solar reflectance should be maximised, and thus a paint formulation at its peak total PVC for each of the five CaCO3:SiO2 ratios is selected. Specifically, the chosen paint formulations have the peak total PVCs of 0.5 (for 1:0 CaCO3:SiO2), 0.5 (for 3:1 CaCO3:SiO2), 0.45 (for 1:1 CaCO3:SiO2), 0.5 (for 1:3 CaCO3:SiO2), and 0.55 (for 0:1 CaCO3:SiO2). The last two paint formulations have very similar solar reflectance and thermal emissivity, and thus only the formulation with a total PVC of 0.5 is chosen of the two due to its lower pigment usage. Therefore, the first four paints will be tested in the outdoor setup.
The outdoor field test setup is shown in . The four samples, metal plates coated with the selected paints, were placed at the top of the four transparent polycarbonate pillars (inset) such that the sample top surface was at the same level as the top cover. Thus, only the top surface of the samples was exposed to sunlight, and thermal radiation only from this top surface was not blocked by the setup. The total area of the polycarbonate that touched each sample was minimal compared to the area of the sample top surface, and thus the heat conduction between the polycarbonate towers and the samples was small. By design, heat convection from the environment was allowed via the louvre side walls. Because an iron substrate constituted ∼99% of the thermal mass of each sample, all four samples had approximately equal thermal masses, and the dependence of heat convection on mass for each sample was similar. Therefore, while heat conduction affected the cooling performance of the paints, the difference in the sample temperature was largely due to the optical properties of the paints.
Figure 5. Outdoor experiment setup. Four samples are placed on four clear polycarbonate towers (insets) and can be simultaneously tested. The cover has four square openings that allow sunlight to reach the samples. Each of the four sides of the test chamber is made of two sets of angled slats that allow air flow while blocking direct sunlight. The sides are coated in white to reduce heat from light absorption. A weather station and a pyranometer are attached to the setup to measure sunlight intensity and ambient temperature.
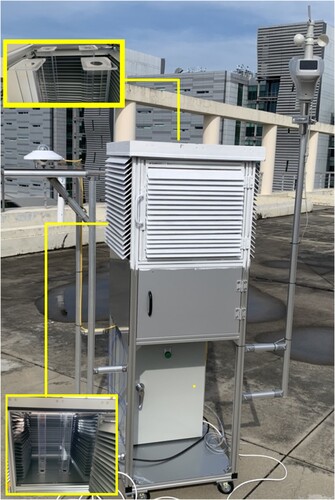
The temperature of the four paint samples, from 6am to 6pm, as a function of time, is described in . During this time, the sky is slightly cloudy. The humidity from 7am to 9am and from 9am to 6pm is 70% and 60%, respectively. The solar irradiation intensity from 9am to noon and from noon to 3pm is approximately 700 and 900 W/m2, respectively. The paint sample with a total PVC of 0.45 and a CaCO3:SiO2 PVC ratio of 1:1 has the best cooling performance throughout the day. Particularly, this paint sample can achieve sub ambient cooling in the early morning as well. The sample is the only paint in this study that has a lower temperature than ambient for a duration after 9am None of the samples can cool below the ambient temperature in the afternoon because of the strong solar irradiation during this time. However, despite the high sunlight intensity, the paint with a total PVC of 0.5 and a CaCO3:SiO2 ratio of 1:0, which has the highest solar reflectance, performs the worst. This suggests that the thermal emission of the paints has a critical role and that the sky acts as a crucial source of heat sink. In the afternoon, the second-worst performing paint sample is the one with a total PVC of 0.5 and a CaCO3:SiO2 PVC ratio of 1:3. This result exemplifies a case of improving thermal emission while excessively compromising solar reflectance.
Figure 6. Measured temperatures of the selected paint samples and the ambient (solid light-blue line) during the daytime. The paint samples are formulated with different total PVCs and CaCO3:SiO2 PVC ratios: 0.5, 1:0 (solid black line); 0.5, 3:1 (dotted dark-blue line); 0.45, 1:1 (dashed green line); and 0.5, 1:3 (dotted orange line). The 0.5, 3:1 formulation shows the best performance while having the least total PVC among the four and is the only paint in this study that achieves sub-ambient cooling at 8–10 am.
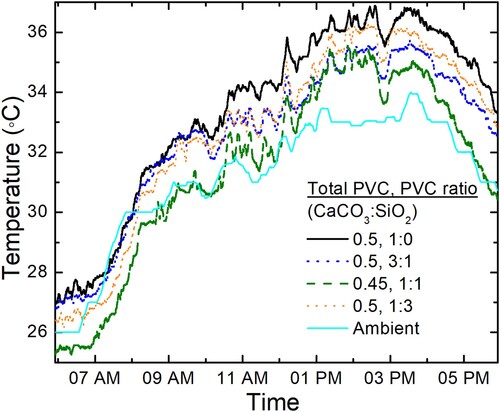
In the morning before 11am, sunlight is relatively weak, and thus the two paints (total PVC = 0.45, PVC ratio = 1:1, and total PVC = 0.5, PVC ratio = 1:3) with higher thermal emissivity perform better than the other two paints with less thermal emissivity. Nevertheless, solar irradiation remains an important factor that causes the temperature of the paint with a total PVC of 0.5 and a PVC ratio of 1:3 to rise above that of the 0.45, 1:1 paint. From noon to 2pm, the temperatures of the 0.45, 1:1 paint and the 0.5, 3:1 paint are quite similar and lower than those of the other two paints, which have either the highest solar reflectance or the highest emissivity. However, in the late afternoon, when the sunlight is weaker, the 0.45, 1:1 paint outperforms the 0.5, 3:1 paint. Therefore, the paint formula with a total PVC of 0.45 and a CaCO3:SiO2 PVC ratio of 1:1 offers the best balance between solar reflection and thermal emission for the scenario. Furthermore, the best performance in this study is achieved with less pigment than in the other samples. Balancing thermal emission and solar irradiation can provide cost-saving benefits as well.
This work has shown that careful adjustment of PVC in two-pigment paint systems can be used to tune the radiative cooling properties of the paints. It is important to note that a paint formulation that provides the best cooling performance is dependent on environmental factors. The paint formula, with a total PVC of 0.45 and a CaCO3:SiO2 PVC ratio of 1:1, which achieves the best cooling here, may not be the most suitable choice in different environments. Studies similar to this work can provide a guideline for a paint formulation that is most suitable for a specific application. Furthermore, since most paints are to be used for many years, the cooling performance of the paints for practical use should be evaluated for at least a year. This is to ensure that the cooling paint formulation provides the most benefits throughout its lifetime. Accelerated weathering tests, according to the ASTM G154 standard, can be used to study the long-term performance of the coatings and will be systematically explored in future works.
4. Conclusion
The effects of the volume concentration of CaCO3 and hollow SiO2 microparticles on the radiative cooling capability of acrylic-based paints were investigated. The solid calcium carbonate particles with an average size of 3.4 µm were synthesised by an ionic exchange method, while the hollow SiO2 microparticles with an average diameter of 1.1 µm were fabricated via sol–gel and etching processes. An acrylic binder was used as a paint vehicle. Paint samples are formulated with PVCs ranging from 0.2 to 0.55. Optical characterisation shows that solar reflectance increases as the total PVC increases until it reaches a peak PVC value. The CaCO3 microparticles are more efficient at scattering sunlight than the hollow SiO2 microparticles. This significant improvement from the addition of CaCO3 is due to its strong rejection of near-infrared light. The addition of the hollow SiO2 is a better choice for improving light scattering at wavelengths around 450 nm, but the paints still suffer from UV absorption of the acrylic resin. The inclusion of the CaCO3 microparticles reduces the thermal emissivity of the paints, while incorporating the hollow SiO2 microparticles improves it. However, as the CaCO3:SiO2 PVC ratio reaches 1:3, further addition of the hollow SiO2 provides diminishing improvements in thermal emission. Four paints, formulated at a peak total PVC for each CaCO3:SiO2 PVC ratio, were selected for field testing based on the measured optical properties. Under sunlight irradiation, the paint with a total PVC of 0.45 and a CaCO3:SiO2 PVC ratio of 1:1 has the best cooling performance throughout the day. The paint also achieves sub-ambient cooling when the solar irradiation is not very strong. This paint formulation offers an optimal tradeoff between solar reflectance and thermal emissivity. This work provides a useful guideline for tuning radiative cooling performance via adjustments in PVC for two-pigment paint systems. Further work on the systematic PVC adjustment of radiative cooling paints in other environments and with different pigment choices could expand the applicability of pigmented paint for practical daytime cooling applications.
Acknowledgements
The authors are also grateful to School of Energy, Environment and Materials, King Mongkut’s University of Technology Thonburi, Thailand, for allowing use of the outdoor testing setup.
Disclosure statement
No potential conflict of interest was reported by the author(s).
Additional information
Funding
References
- Al-Obaidi, K. M., M. Ismail, and A. M. Abdul Rahman. 2014. “Passive Cooling Techniques Through Reflective and Radiative Roofs in Tropical Houses in Southeast Asia: A Literature Review.” Frontiers of Architectural Research 3 (3): 283–297. doi:10.1016/j.foar.2014.06.002.
- Alden, J. D., S. Atiganyanun, R. Vanderburg, S. H. Lee, J. B. Plumley, O. K. Abudayyeh, S. M. Han, and S. E. Han 2019. “Radiative Cooling by Silicone-Based Coating with Randomly Distributed Microbubble Inclusions.” Journal of Photonics for Energy 9: 032705. doi:10.1117/1.JPE.9.032705.
- Aragón, S. R., and R. Pecora. 1976. “Theory of Dynamic Light Scattering from Polydisperse Systems.” The Journal of Chemical Physics 64 (6): 2395–2404. doi:10.1063/1.432528.
- Atiganyanun, S. 2021. “Use of Hollow Silica and Titanium Dioxide Microparticles in Solar Reflective Paints for Daytime Radiative Cooling Applications in a Tropical Region.” Journal of Photonics for Energy 11: 022103. doi:10.1117/1.JPE.11.022103.
- Centeno, M. 1982. “New Formulae for the Equivalent Night sky Emissivity.” Solar Energy 28 (6): 489–498. doi:10.1016/0038-092X(82)90320-6.
- Chae, D., S. Son, H. Lim, P.-H. Jung, J. Ha, and H. Lee 2021. “Scalable and Paint-Format Microparticle–Polymer Composite Enabling High-Performance Daytime Radiative Cooling.” Materials Today Physics 18: 100389. doi:10.1016/j.mtphys.2021.100389.
- Chaiyosburana, W., W. Rakwichian, S. Vaivudh, and N. Ketjoy. 2013. “Optimizing High Solar Reflective Paint to Reduce Heat Gain in Building.” International Journal of Renewable Energy 8: 15–23.
- Diebold, M. P. 2014. “Scattering by Groups of Particles.” In Application of Light Scattering to Coatings: A User’s Guide, edited by M. P. Diebold, 31–58. Springer International Publishing. doi:10.1007/978-3-319-12015-7_2.
- Fan, D., H. Sun, and Q. Li. 2019. “Thermal Control Properties of Radiative Cooling Foil Based on Transparent Fluorinated Polyimide.” Solar Energy Materials and Solar Cells 195: 250–257. doi:10.1016/j.solmat.2019.03.019.
- Febrida, R., A. Cahyanto, E. Herda, V. Muthukanan, N. Djustiana, F. Faizal, C. Panatarani, and I. M. Joni 2021. “Synthesis and Characterization of Porous CaCO3 Vaterite Particles by Simple Solution Method.” Materials 14: 4425. doi:10.3390/ma14164425.
- Gentle, A. R., and G. B. Smith. 2010. “Radiative Heat Pumping from the Earth Using Surface Phonon Resonant Nanoparticles.” Nano Letters 10 (2): 373–379. doi:10.1021/nl903271d.
- Hanif, M., T. M. I. Mahlia, A. Zare, T. J. Saksahdan, and H. S. C. Metselaar. 2014. “Potential Energy Savings by Radiative Cooling System for a Building in Tropical Climate.” Renewable and Sustainable Energy Reviews 32: 642–650. doi:10.1016/j.rser.2014.01.053.
- Harrison, A. W., and M. R. Walton. 1978. “Radiative Cooling of TiO2 White Paint.” Solar Energy 20 (2): 185–188. doi:10.1016/0038-092X(78)90195-0.
- Hossain, M. M., and M. Gu. 2016. “Radiative Cooling: Principles, Progress, and Potentials.” Advanced Science 3: 1500360. doi:10.1002/advs.201500360.
- Hsu, P.-C., A. Y. Song, P. B. Catrysse, C. Liu, Y. Peng, J. Xie, S. Fan, and Y. Cui 2016. “Radiative Human Body Cooling by Nanoporous Polyethylene Textile.” Science 353 (6303): 1019–1023. doi:10.1126/science.aaf5471.
- Huang, J., M. Li, and D. Fan. 2021. “Core-shell Particles for Devising High-Performance Full-day Radiative Cooling Paint.” Applied Materials Today 25: 101209. doi:10.1016/j.apmt.2021.101209.
- Huang, M., X. Yu, J. Wan, M. Du, X. Wang, Q. Sun, and G. H. Tang 2022. “All-day Effective Radiative Cooling by Optically Selective and Thermally Insulating Mesoporous Materials.” Solar Energy 235: 170–179. doi:10.1016/j.solener.2022.02.015.
- International Energy Agency. 2019. The Future of cooling in Southeast Asia – Analysis. IEA https://www.iea.org/reports/the-future-of-cooling-in-southeast-asia.
- International Energy Agency. 2021. Cooling – Analysis. IEA https://www.iea.org/reports/cooling.
- Isaac, M., and D. P. van Vuuren. 2009. “Modeling Global Residential Sector Energy Demand for Heating and air Conditioning in the Context of Climate Change.” Energy Policy 37 (2): 507–521. doi:10.1016/j.enpol.2008.09.051.
- Jarzembski, M., M. Norman, K. Fuller, V. Srivastava, and D. Cutten. 2003. “Complex Refractive Index of Ammonium Nitrate in the 2-20-Micrometer Spectral Range.” Applied Optics 42 (6): 922–930. doi:10.1364/AO.42.000922.
- Jitian, S., and I. Bratu. 2012. “Determination of Optical Constants Of Polymethyl Methacrylate Films from IR Reflection-Absorption Spectra.” AIP Conference Proceedings 1425: 26–29. doi:10.1063/1.3681958.
- Karn, A., V. Chintala, and S. Kumar. 2019. “An Investigation Into sky Temperature Estimation, its Variation, and Significance in Heat Transfer Calculations of Solar Cookers.” Heat Transfer-Asian Research 48 (5): 1830–1856. doi:10.1002/htj.21459.
- Kitamura, R., L. Pilon, and M. Jonasz. 2007. “Optical Constants of Silica Glass from Extreme Ultraviolet to far Infrared at Near Room Temperature.” Applied Optics 46 (33): 8118–8133. doi:10.1364/AO.46.008118.
- Lei, X., B. Yu, H.-L. Cong, C. Tian, Y.-Z. Wang, Q.-B. Wang, and C.-K. Liu 2014. “Synthesis of Monodisperse Silica Microspheres by a Modified Stöber Method.” Integrated Ferroelectrics 154 (1): 142–146. doi:10.1080/10584587.2014.904651.
- Li, X., J. Peoples, Z. Huang, Z. Zhao, J. Qiu, and X. Ruan 2020. “Full Daytime Sub-Ambient Radiative Cooling in Commercial-Like Paints with High Figure of Merit.” Cell Reports Physical Science 1 (10): 100221. doi:10.1016/j.xcrp.2020.100221.
- Li, X., J. Peoples, P. Yao, and X. Ruan. 2020. Remarkable Daytime Sub-ambient Radiative Cooling in BaSO4 Nanoparticle Films and Paints. ArXiv201101161 Phys.
- Li, X., J. Peoples, P. Yao, and X. Ruan. 2021. “Ultrawhite BaSO4 Paints and Films for Remarkable Daytime Subambient Radiative Cooling.” ACS Applied Materials & Interfaces 13 (18): 21733–21739. doi:10.1021/acsami.1c02368.
- Li, T., Y. Zhai, S. He, W. Gan, Z. Wei, M. Heidarinejad, D. Dalgo, et al. 2019. “A Radiative Cooling Structural Material.” Science 364 (6442): 760–763. doi:10.1126/science.aau9101.
- Lu, Y., Z. Chen, L. Ai, X. Zhang, J. Zhang, J. Li, W. Wang, R. Tan, N. Dai, and W. Song 2017. “A Universal Route to Realize Radiative Cooling and Light Management in Photovoltaic Modules.” Solar RRL 1 (10): 1700084. doi:10.1002/solr.201700084.
- Ma, H., L. Wang, S. Dou, H. Zhao, M. Huang, Z. Xu, X. Zhang, et al. 2021. “Flexible Daytime Radiative Cooling Enhanced by Enabling Three-Phase Composites with Scattering Interfaces Between Silica Microspheres and Hierarchical Porous Coatings.” ACS Applied Materials & Interfaces 13 (16): 19282–19290. doi:10.1021/acsami.1c02145.
- Mandal, J., Y. Fu, A. C. Overvig, M. Jia, K. Sun, N. N. Shi, H. Zhou, X. Xiao, N. Yu, and Y. Yang 2018. “Hierarchically Porous Polymer Coatings for Highly Efficient Passive Daytime Radiative Cooling.” Science 362 (6412): 315–319. doi:10.1126/science.aat9513.
- Mie, G. 1908. “Beiträge zur Optik trüber Medien, speziell kolloidaler Metallösungen.” Annalen der Physik 330 (3): 377–445. doi:10.1002/andp.19083300302.
- Mourou, C., M. Zamorano, D. P. Ruiz, and M. Martín-Morales. 2022. “Cool Surface Strategies with an Emphasis on the Materials Dimension: A Review.” Applied Sciences 12: 1893. doi:10.3390/app12041893.
- Orel, B., M. K. Gunde, and A. Krainer. 1993. “Radiative Cooling Efficiency of White Pigmented Paints.” Solar Energy 50 (6): 477–482. doi:10.1016/0038-092X(93)90108-Z.
- Peoples, J., X. Li, Y. Lv, J. Qiu, Z. Huang, and X. Ruan 2019. “A Strategy of Hierarchical Particle Sizes in Nanoparticle Composite for Enhancing Solar Reflection.” International Journal of Heat and Mass Transfer 131: 487–494. doi:10.1016/j.ijheatmasstransfer.2018.11.059.
- Raman, A. P., M. A. Anoma, L. Zhu, E. Rephaeli, and S. Fan. 2014. “Passive Radiative Cooling Below Ambient air Temperature Under Direct Sunlight.” Nature 515 (7528): 540–544. doi:10.1038/nature13883.
- Rephaeli, E., A. Raman, and S. Fan. 2013. “Ultrabroadband Photonic Structures To Achieve High-Performance Daytime Radiative Cooling.” Nano Letters 13 (4): 1457–1461. doi:10.1021/nl4004283.
- Tao, Y., Z. Mao, Z. Yang, and J. Zhang. 2021. “CaCO3 as a new Member of High Solar-Reflective Filler on the Cooling Property in Polymer Composites.” Journal of Vinyl and Additive Technology 27 (2): 275–287. doi:10.1002/vnl.21801.
- Tong, Z., J. Peoples, X. Li, X. Yang, H. Bao, and X. Ruan 2022. “Electronic and Phononic Origins of BaSO4 as an Ultra-Efficient Radiative Cooling Paint Pigment.” Materials Today Physics 24: 100658. doi:10.1016/j.mtphys.2022.100658.
- Wang, J., S. Liu, X. Meng, W. Gao, and J. Yuan. 2021. “Application of Retro-Reflective Materials in Urban Buildings: A Comprehensive Review.” Energy and Buildings 247: 111137. doi:10.1016/j.enbuild.2021.111137.
- Wijewardane, S., and D. Y. Goswami. 2012. “A Review on Surface Control of Thermal Radiation by Paints and Coatings for new Energy Applications.” Renewable and Sustainable Energy Reviews 16 (4): 1863–1873. doi:10.1016/j.rser.2012.01.046.
- Wriedt, T. 2012. “Mie Theory: A Review.” In The Mie Theory: Basics and Applications, edited by W. Hergert, and T. Wriedt, 53–71. Springer. doi:10.1007/978-3-642-28738-1_2.
- Xinyuan, Z., J. Wang, Y. Li, R. Yang, and Y. Zhan. 2022. “Spectral Decoupling of Cooperative Emissivity in Silica-Polymer Metamaterials for Radiative Cooling.” Optics Letters 47 (10): 2506–2509. doi:10.1364/OL.455302.
- Zhai, Y., Y. Ma, S. N. David, D. Zhao, R. Lou, G. Tan, R. Yang, and X. Yin 2017. “Scalable-manufactured Randomized Glass-Polymer Hybrid Metamaterial for Daytime Radiative Cooling.” Science 355 (6329): 1062–1066. doi:10.1126/science.aai7899.
- Zhang, X. 2017. “Metamaterials for Perpetual Cooling at Large Scales.” Science 355 (6329): 1023–1024. doi:10.1126/science.aam8566.
- Zhang, T., Q. Zhang, J. Ge, J. Goebl, M. Sun, Y. Yan, Y. Liu, C. Chang, J. Guo, and Y. Yin 2009. “A Self-Templated Route to Hollow Silica Microspheres.” The Journal of Physical Chemistry C 113 (8): 3168–3175. doi:10.1021/jp810360a.
- Zhao, D., A. Aili, Y. Zhai, S. Xu, G. Tan, X. Yin, and R. Yang 2019. “Radiative Sky Cooling: Fundamental Principles, Materials, and Applications.” Applied Physics Reviews 6: 021306. doi:10.1063/1.5087281.
- Zhu, L., A. P. Raman, and S. Fan. 2015. “Radiative Cooling of Solar Absorbers Using a Visibly Transparent Photonic Crystal Thermal Blackbody.” Proceedings of the National Academy of Sciences 112 (40): 12282–12287. doi:10.1073/pnas.1509453112.