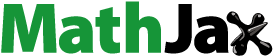
Abstract
Micropollutants (MPs) include organic chemicals, for example, pharmaceuticals and personal care products. MPs have been detected in the aquatic environment at low concentrations (ng/L–µg/L), which may lead to negative impacts on the ecosystem and humans. Phytoremediation is a green clean-up technology, which utilizes plants and their associated rhizosphere microorganisms to remove pollutants. The selection of plant species is important for the effectiveness of the phytoremediation of MPs. The plant species Phragmites australis, Typha angustifolia, and Juncus effuses are often used for MP removal. In this study, batch experiments were conducted to select plant species with an optimal ability to remove MPs, study the effect of temperature on MP removal in plants and the phytotoxicity of MPs. This study also explored the degradation of a persistent MP propranolol in plants in more detail. Data show that all three investigated plant species removed most MPs efficiently (close to 100 %) at both 10 and 21.5 °C. The tested plant species showed a different ability to translocate and accumulate propranolol in plant tissues. Typha angustifolia and Juncus effuses had a higher tolerance to the tested MPs than Phragmites australis. Typha angustifolia and Juncus effuses are recommended to be applied for phytoremediation of MPs.
Novelty statement The novelty of this study is the selection of Typha angustifolia and Juncus effuses as proper plant species for phytoremediation of micropollutants (MPs). These two plant species were selected due to their good ability to remove MPs, tolerate low temperature, and resist the toxicity of MPs. The outcomes from this study can also be applied for constructed wetlands in removing MPs from wastewater. This study demonstrates the uptake and degradation processes of persistent MP propranolol in plants in more detail. Understanding the degradation mechanisms of a MP in plants is significant not only for the application of phytoremediation on MP removal but also for the development of constructed wetland studies.
Introduction
Micropollutants (MPs) have been widely detected in the aquatic environment, which causes stress to water sources and threatens the health of the ecosystem and humans. Various categories of MPs exist, for example, pharmaceuticals, personal care products, steroid hormones, industrial chemicals, and pesticides (Luo et al. Citation2014). MPs are detected in the aquatic environment in concentrations ranging from ng/L to µg/L, in e.g., wastewater, groundwater, surface water, and drinking water (Luo et al. Citation2014). The occurrence of MPs in the aquatic environment leads to potential risks for the ecosystem, such as estrogenic effects on fish (Fent et al. Citation2006) or microorganisms’ resistance to antibiotics (Pruden et al. Citation2006). Thus, the removal of MPs from the aquatic environment is important for improving water quality.
Conventional wastewater treatment plants (WWTPs) are the first barrier to prevent MPs from spreading in the aquatic environment. However, the removal efficiency of MPs differs in WWTPs due to e.g., the physic-chemical properties of a MP and the operational conditions of WWTPs (Luo et al. Citation2014). Tran et al. (Citation2018) reviewed the removal efficiencies of 60 MPs in full-scale municipal WWTPs from Asia, Europe, and North America. They reported that biological WWTPs showed a high removal (median >80 %) of particular MPs, such as caffeine, ibuprofen, naproxen, and amoxicillin, while other MPs were poorly removed (median <40 %), such as metoprolol, propranolol, clofibric acid and carbamazepine. Unremoved MPs in WWTP effluent are continuously discharged into the aquatic environment. Consequently, the discharge of municipal wastewater effluent with MPs becomes the main route leading to MP pollution of surface water (Kasprzyk-Hordern et al. Citation2009).
To improve MP removal in WWTPs, wastewater effluent can be treated by post-treatment technologies. Various physical, chemical, and biological approaches have been developed and used as possible post-treatment technologies. Among them, biological treatments are considered a promising option as they are generally cost-effective and environmental-friendly technologies, such as phytoremediation. Phytoremediation is a green technology in which plants and their associated rhizosphere microorganisms degrade and sequester various organic and inorganic pollutants from solid, liquid, and gaseous phases (Pilon-Smits Citation2005). Selecting plants is important for phytoremediation. Optimal plants for phytoremediation are fast-growing, resistant to cold, easily planted and maintained, have high evapotranspiration, can transform contaminants to non-toxic or less toxic products, have easily harvested above-ground parts, and have a good ability to accumulate contaminants in above-ground biomass (Schnoor Citation1997; Khan et al. Citation2020).
Different plants are used in phytoremediation. Some aquatic plants can remove MPs successfully from water, such as Typha latifolia, Phragmites australis, Iris pseudacorus, and Juncus effuses (Lv et al. Citation2016; Zhang et al. Citation2016; He et al. Citation2017). Although these plants have shown their ability to uptake and degrade MPs, it is impossible to identify a species with optimal MP removal due to different experimental setups and target MPs used in the studies. Moreover, phytoremediation is often applied by means of constructed wetlands (CWs) to treat wastewater (Fletcher et al. Citation2020). The plants Phragmites australis and Typha angustifolia are often used in CW studies, such as Hijosa-Valsero et al. (Citation2010) and He et al. (Citation2018), though the optimal plant species for MP removal in CWs has not yet been determined.
The aim of this study was therefore to select plant species with an optimal ability to remove MPs under well-controlled conditions, among plants species that have a proven functionality in CWs: Phragmites australis, Typha angustifolia, and Juncus effuses (Vymazal Citation2011, Citation2013). For these plants, the effect of temperature on MP removal in plants and the phytotoxicity of MPs was studied. To get the first insight into MP uptake in plants and subsequent in vivo degradation, these processes were studied for propranolol in more detail.
Materials and methods
Chemicals and reagents
Trimethoprim (TRM), metoprolol (MET), benzotriazole (BTA), carbamazepine (CBZ), caffeine (CAF), propranolol (PRO), sulfamethoxazole (SMZ), furosemide (FRS), mecoprop (MCPP), and diclofenac (DFC) were purchased from Sigma–Aldrich (U.S), and irbesartan (IBT) from TOKYO CHEMICAL INDUSTRY (Japan). Internal standards CAF-D9, SMX-D4, FRS-D5, MCPP-D6, DFC-D4 were purchased from LGC (Germany) and PRO-D7 from MERCK (the Netherlands). Details of these chemicals are presented in Table S1. Hydrogen chloride (HCl) was purchased from VWR chemicals (U.S). Methanol was bought from ACTU-ALL CHEMICALS (the Netherlands). Acetonitrile, ultra-water, and formic acid used in liquid chromatography–mass spectrometry (LC-MS) was purchased from ACTU-ALL CHEMICALS (The Netherlands).
Plant growth conditions
Phragmites australis, Typha angustifolia, and Juncus effuses were purchased from the nursery Jörg Petrowsky (Wasserpflanzengärtnerei, Eschede, Germany). All plants were gently washed with tap water and transferred to pots filled with clean gravel. Gravel with a size of 12 mm was purchased from GAMMA (the Netherlands). The gravel was washed with tap water and dried at room temperature before use.
Plants were cultivated under greenhouse conditions (i.e., 16:8 light: dark cycle, 18–25 °C, relative humidity: 43 %) and fed with Hoagland culture medium (Table S2) of 10–50 % strength for 6–9 weeks. After cultivation, well-grown plants with green leaves were transferred to new pots with 500 g clean gravel to start the experiments (Figure S1).
Experimental design
Removal of MPs by plants
Batch experiments were performed to compare MP removal by Phragmits australis, Tyhpha angustifolia, and Juncus effuses. A mixed MP solution (TRM, MET, BTA, CBZ, CAF, PRO, SMZ, FRS, MCPP, DFC, and IBT) with 500 mg/L of each MP was prepared in methanol and diluted to 8 µg/L in Hoagland culture medium. The pots with plants and 500 g gravel were exposed to 300 mL 8 µg/L MP solution (each compound) in triplicate. The studied concentration of 8 µg/L MPs was slightly higher than the concentration of MPs reported in wastewater effluent of Bennekom WWTP, the Netherlands (Lei et al. Citation2021), considering the measurement of MPs in the solution during the experiments. After adding the MP solution of 300 mL to the pots, the solution reached the top of the gravel layer. The pots (except for leaves) were covered with black bags to avoid photodegradation of MPs but still allow airflow (Figure S1). Two controls were studied in duplicate: pots filled with 500 g clean gravel and 300 mL 8 µg/L MP solution, and pots with plants, gravels, and 300 mL Hoagland culture medium without MPs. All pots were placed in the greenhouse for 21 days (Figure S1). This incubation time was selected based on the work of Lv et al. (Citation2016) and He et al. (Citation2017). To compensate for the water loss due to evapotranspiration during the incubation, the evaporated amount was added with deionized water to all pots daily. Liquid samples of 2 mL were collected from all pots on days 0, 1, 3, 7, and 21 for analysis. In those days, pH and dissolved oxygen (DO) were measured in the aqueous phase of all pots with an HQ40d multi-meter (Hach, USA).
Effect of temperature and phytotoxicity of MPs
The effect of temperature on MP removal was tested in plants. A batch of plants was prepared as described in 2.3.1 and incubated at 10 °C in a greenhouse (Figure S1). The phytotoxicity of MPs on the tested plant species was studied according to the method of Trapp et al. (Citation2000) by evaluating the transpiration rate of plants during their incubation at 21.5 °C, All pots were weighed daily on a balance (Sartorius, German) for 21 days. The transpiration rate of each plant species was calculated as:
(1)
(1)
where
is the transpiration rate (g/day),
is the exposure time (day),
is the weight of whole pot (g),
is the average evaporation in the unplanted controls (g) at
Degradation of propranolol in plants
The degradation of propranolol in the three plant species was studied by comparing the distribution and accumulation of propranolol in plant tissues. A 500 mg/L solution of propranolol in ultrapure water was diluted to a solution of 10.6 µg/L in Hoagland culture medium. This concentration of 10.6 µg/L was slightly higher than 8 µg/L used in 2.3.1, due to taking into consideration the measurement of MPs in plant tissues after the experiment. This medium with propranolol was used to incubate plants as described in 2.3.1. Liquid samples of 2 mL were collected from all pots on days 1, 3, 7, and 21. At each sampling time, triplicate pots with plants were harvested, and plants were divided into roots and rhizomes (RR), and leaves and stems (LS). All plant tissues were frozen in liquid nitrogen and stored at −20 °C until extraction.
The bioconcentration factor (BCF) of RR (BCFRR) or LS (BCFLS) was calculated based on the ratio of the final concentration of propranolol in RR or LS to that in the aqueous phase. The translocation factor (TF) of each plant species was calculated as the ratio of the propranolol concentration in LS to RR.
Propranolol extraction
The extraction of propranolol from plant tissue samples was based on a modified method by He et al. (Citation2017). In short, 3 g fresh plant tissues were ground to powder in liquid nitrogen and mixed with 6 mL of 0.1 M HCl/acetonitrile (50/50, v/v). After this, 2 mL supernatant was cleaned and concentrated by solid-phase extraction (SPE). First, an Oasis HLB SPE cartridge (500 mg, 6 mL, Waters, USA) was pre-conditioned with 5 mL methanol and 5 mL equilibrating water. This equilibrating water was prepared with 5 mL deionized water and 50 µL pH 10 buffer (Merck KGaA, Germany). Then, 2 mL samples were added to the cartridge. Finally, the cartridge was washed with 5 mL equilibrating water and eluted with 10 mL 2 % NH4OH in methanol. The elute was evaporated until dryness under a gentle N2 flow and re-dissolved in 500 µL of 3.3 % methanol in ultrapure water. The validation of the propranolol extraction method is shown in Text S1.
Chemical analysis
The concentration of 11 MPs in liquid samples was measured by an Ultra High Performance Liquid Chromatography (UHPLC) ACQUITY UPLC H-class series (Waters, US), equipped with a Waters Acquity autosampler and tandem quadrupole mass spectrometer XEVO TQ-XS (Milford, MA, United States). MPs were ionized by Electro Spry Ionization (ESI) in alternating positive and negative modes to get the best signal for each analyzed MP. Scheduled multiple reaction monitoring (SMRM) was used to quantify each MP based on the intensity of the ion obtained by fragmentation of the ionized molecule (parent ion), see Table S3. The analytic column was a water Acuity UHPLC CSH Phenyl-Hexyl (1.7 μm 2.1*150 mm inline filter, Milford, Massachusetts, United States) coupled with a guard column (Waters, USA) with the same phase. The injection volume was 50 μL for each sample. The composition and flow rate of mobile phases and chromatographic separation program are described by He et al. (Citation2016). The detection range of 11 MPs was between 50 and 900 ng/L. Internal standards were used to correct the matrix effect on the instrumental analysis (Table S1).
The concentration of propranolol in liquid and plant tissues samples was measured by HPLC Ultimate 3000 (ThermoFisher Scientific, USA), equipped with an Orbitrap Mass Spectrometer QExactive (ThermoFisher Scientific, USA). The details of operation parameters and analysis procedures were previously described by Lei et al. (Citation2021).
Results and discussion
MP removal by plants
Batch experiments were performed to compare the removal efficiencies of 11 target MPs in the three selected plant species. All batches showed a stable pH and DO after initial MP exposure (Figures S2, S3). Phragmites australis, Typha angustifolia, and Juncus effuses efficiently removed all target MPs with almost 100 % removal efficiency at 21.5 °C after 21 days of incubation (), with more than 90 % removal after the first 7 days (Figure S4). This indicates that the three plant species had a similar removal performance under the experimental conditions. Furthermore, the presence of plants played an important role in the removal of MPs, as a higher removal efficiency was found compared to batches with the absence of plants, except for IBT, CAF, and SMZ where similar removals were found (). Especially, the value of plant presence was most pronounced for CBZ, MCPP, and DFC, which are often poorly removed by WWTPs as reported by e.g., Morasch et al. (Citation2010) and Luo et al. (Citation2014).
Table 1. Removal efficiency of MPs by the presence and absence of plants at 10 and 21.5 °C after 21 days.
The incubation temperature of 10 °C was used to simulate low-temperature conditions in this study, as it is the minimum ambient temperature from the beginning of summer to the end of autumn in moderate climate countries, such as the Netherlands (Figure S5). With decreasing the temperature from 21.5 to 10 °C, most MPs were still highly removed (>80 %) in the presence of the plants while the removal efficiency of most MPs decreased from max. 98 % to min. 10 % when no plants were present (). This indicates that at a lower temperature, the plants sustained the MP removal strongly, showing that plants are expected to play an essential role in MP removal in CWs at a low temperature. This is in line with the findings of Hijosa-Valsero et al. (Citation2010), who also observed that the planted subsurface flow CW showed a higher removal of ketoprofen, naproxen, salicylic acid, carbamazepine, methyl dihydrojasmonate, galzxolide, and tonalide in wintertime than the unplanted subsurface flow CW. The three plants tested appear to be equally effective in MP removal at both studied temperatures.
Phytotoxicity of MPs
MPs are commonly detected in the aquatic environment in mixtures. Even though the individual MPs are present at low concentrations with limited toxicity, the mixture of MPs can be detrimental for organisms due to additive or synergistic effects (Schwarzenbach et al. Citation2006). Assessing the plants’ ability to tolerate the toxicity of a MP mixture is necessary when selecting a proper plant species for phytoremediation of MPs. The phytotoxicity of our target MPs on Phragmites australis, Typha angustifolia, and Juncus effuses was identified by determining the transpiration rate of plants.
The transpiration rate of Typha angustifolia and Juncus effuses in the pots spiked with MPs was close to that in the pots without MPs at 21.5 °C (). This means that the target MPs had a limited phytotoxic effect on the transpiration of Typha angustifolia and Juncus effuses. However, the transpiration rate of Phragmites australis in the pots spiked with MPs was lower than that in the pots without MPs at 21.5 °C. This indicates that MPs had a phytotoxic effect on Phragmites australis. Thus, among the three tested plant species, Typha angustifolia and Juncus effuses had a higher tolerance toward the phytotoxic effect of MPs.
Degradation of propranolol in plants
In this study, phytoremediation of propranolol was investigated in more detail to get insights into the uptake and in vivo degradation of MPs in plants. Previous studies found that propranolol can be taken up by plants, for example, Carter et al. (Citation2014), Hurtado et al. (Citation2016), and He et al. (Citation2018). However, few studies focus on the influence of various plant species and the degradation processes of propranolol in plants. Propranolol is a good test compound, as it has been widely detected in WWTP effluents and rivers from ng/L to µg/L, and shows persistence in the aquatic environment (Ternes Citation1998; Huggett et al. Citation2003; Thomas and Hilton Citation2004; Bendz et al. Citation2005). Hence, propranolol is an important MP compound that should be removed in wastewater.
Propranolol was almost completely removed (>99 %) from the aqueous phase by Phragmites australis, Typha angustifolia, or Juncus effuses after 21 days of incubation (), with most of its removal (appr.89 %) during the first day. Propranolol was already detected in the roots and rhizomes (RR) and leaves and stems (LS) on day 1 (). This shows that plants immediately took up propranolol from the aqueous solution via RR, and then transported propranolol from RR to LS. This is in line with previous studies that propranolol could be taken up by plants and it was detected in plant tissues (Carter et al. Citation2014; Hurtado et al. Citation2016; He et al. Citation2018).
Figure 2. Concentration of propranolol in the aqueous phase, roots and rhizomes (RR), and leaves and stems (LS). Data are mean value ± standard deviation (n = 3).
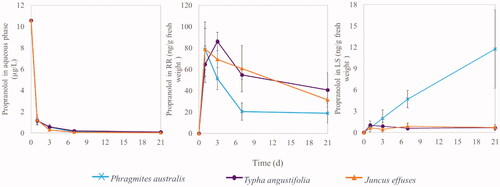
The presence of propranolol in plants is linked to the lipophilicity of propranolol. The lipophilicity of organic chemicals (assessed by log Kow) is an important physic-chemical property to predict their possibility to be taken up and translocated by plants (Briggs et al. Citation1982). In theory, organic chemicals with moderate lipophilicity (i.e., log Kow ranges from 1 to 3.5) are efficiently removed by plants through uptake into the plant tissues (Schröder and Collins Citation2002). This is due to such moderate lipophilicity helping the compounds to not only pass in biomembranes of roots but also provide sufficient water solubility for translocating from the roots to the leaves (Trapp and Karlson Citation2001). Log Kow of propranolol is 3.48 in Table S1, which is in range of the moderate lipophilicity, and therefore explains the presence of propranolol in both RR and LS of the three plant species tested.
After the initial uptake, the concentration of propranolol decreased in RR (), indicating that propranolol was phytodegraded in RR and/or translocated from RR to LS. Moreover, an increasing propranolol concentration was observed in LS of the plant species over time, especially in Phragmites australis (). After 21 days, the highest mass of propranolol accumulated in Typha angustifolia, almost completely in its RR (3.2 ± 1.1 µg) (). Juncus effuses and Phragmite australis accumulated the propranolol in the plants with a total of 1.5 ± 0.3 and 1.4 ± 0.6 µg, respectively. Moreover, like Typha angustifolia, also for Juncus effuses and Phragmites australis, propranolol was mainly accumulated in RR and a relatively small portion in their LS (). These results show that the studied plant species efficiently removed propranolol from the aqueous phase, but varied in accumulation in the plants after uptake, with RR being the main plant organs to store propranolol. These transport and accumulation processes are further characterized in the following using the parameters of .
Table 2. Mass of propranolol accumulated in RR and LS of three plant species and their bioconcentration factors of RR (BCFRR) and LS (BCFLS) as well as translocation factors (TF) after 21 days.
The bioconcentration factor (BCF) and translocation factor (TF) of the plant species are important parameters to identify their ability to uptake propranolol from the aqueous phase and translocate it from RR to LS (). After 21 days, the RR of Juncus effuses had a higher BCF value than the RR of the other two plants. This indicates that under the tested conditions, propranolol moves easier from the aqueous phase and through the cell membranes of RR tissues of Juncus effuses, followed by Phragmites australis and Typha angustifolia. Phragmites australis showed higher BCF of LS and TF values than for the other two plant species. This means that propranolol is more efficiently transported via cell fluids to LS of Phragmites australis and taken up by its LS than other plant species.
In addition, a high standard deviation was observed in the transport and accumulation parameter values from the studied batches (). This may be due to the impact of inhomogeneity of the plant material and varying biological conditions in the plants. This high standard deviation in the data is likely to be overcome by increasing the studied concentration of MPs to mg/L, such as done in the studies of Lv et al. (Citation2016) and Zhang et al. (Citation2016). In our study, the concentration of propranolol (10.6 µg/L) was much lower but more relevant for MP levels in the aquatic environment from ng/L to µg/L. Despite the variability in the data, the tested plant species showed a significant difference in uptake and translocation of propranolol in plant tissues.
Application and conclusions
This paper describes the removal of 11 target MPs by Phragmites australis, Typha angustifolia, and Juncus effuses. The three studied plant species efficiently removed most MPs at both 10 and 21.5 °C. The presence of plants showed a significant positive effect on improving the removal of most MPs at 21.5 °C, especially for persistent MPs CBZ, MCPP, and DFC. At 10 °C, such a positive effect of the plants was even more pronounced for the removal of most MPs. When considering the effect of MP phytotoxicity, Typha angustifolia and Juncus effuses were least affected by the presence of MPs.
The uptake of propranolol was efficient for all three plant species, and its translocation was the highest for Phragmites australis, with the highest accumulation in leaves and stems. It is unclear whether this is linked to the phytotoxic sensitivity of this plant species to MPs. The outcomes from this study will help to understand the degradation mechanisms of propranolol in plants, and be conducive to explore and predict propranolol removal in other plants.
Typha angustifolia and Juncus effuses are suitable plants for phytoremediation of MPs due to their good ability to remove MPs and tolerant low temperature and phytotoxicity of MPs. Since these plant species are often applied in CWs for removing MPs from WWTP effluents, these are also optimal from a practical perspective.
BIJP-2021-0479.R1_SUPPORTING_INFO.docx
Download MS Word (730.6 KB)Acknowledgments
The authors thank the support provided by China scholarship Council (CSC) and Waterschap Vallei en Veluwe (Apeldoorn, the Netherlands) for the research of Yu Lei at Wageningen University and Research. The authors appreciate the contributions of Miriam Verdurmen from Waterschap Vallei en Veluwe (Apeldoorn, the Netherlands), and Rinie Verwoert, Rohan van Genderen, Wilfred Krijnen and Martijn Verweij from Nergena greenhouse in Unifarm of Wageningen University and Research, the Netherlands. The authors also thank Xintong Zhou and Katja Grolle from Environmental Technology of Wageningen University and Research, the Netherlands.
Disclosure statement
The authors report there are no competing interests to declare.
References
- Bendz D, Paxéus N, Ginn T, Loge F. 2005. Occurrence and fate of pharmaceutically active compounds in the environment, a case study: Höje River in Sweden. J Hazard Mater. 122(3):195–204. doi:10.1016/j.jhazmat.2005.03.012.
- Briggs G, Bromilow R, Evans A. 1982. Relationships between lipophilicity and root uptake and translocation of non-ionised chemicals by barley. Pesticide Sci. 13:495–504. doi:10.1002/ps.2780130506.
- Carter L, Harris E, Williams M, Ryan J, Kookana R, Boxall A. 2014. Fate and uptake of pharmaceuticals in soil–plant systems. J Agric Food Chem. 62(4):816–825. doi:10.1021/jf404282y.
- Fent K, Weston A, Caminada D. 2006. Ecotoxicology of human pharmaceuticals. Aquat Toxicol. 76(2):122–159. doi:10.1016/j.aquatox.2005.09.009.
- Fletcher J, Willby N, Oliver D, Quilliam R. 2020. Phytoremediation using aquatic plants. In: Shmaefsky B, editor. Phytoremediation, in-situ applications. Cham: Springer International Publishing; p. 205–260.
- He Y, Langenhoff A, Sutton N, Rijnaarts H, Blokland M, Chen F, Huber C, Schröder P. 2017. Metabolism of ibuprofen by Phragmites australis: uptake and phytodegradation. Environ Sci Technol. 51(8):4576–4584. doi:10.1021/acs.est.7b00458.
- He Y, Sutton N, Lei Y, Rijnaarts H, Langenhoff A. 2018. Fate and distribution of pharmaceutically active compounds in mesocosm constructed wetlands. J Hazard Mater. 357:198–206. doi:10.1016/j.jhazmat.2018.05.035.
- He Y, Sutton N, Rijnaarts H, Langenhoff A. 2016. Degradation of pharmaceuticals in wastewater using immobilized TiO2 photocatalysis under simulated solar irradiation. Appl Catal B. 182:132–141. doi:10.1016/j.apcatb.2015.09.015.
- Hijosa-Valsero M, Matamoros V, Sidrach-Cardona R, Martín-Villacorta J, Bécares E, Bayona JM. 2010. Comprehensive assessment of the design configuration of constructed wetlands for the removal of pharmaceuticals and personal care products from urban wastewaters. Water Res. 44(12):3669–3678. doi:10.1016/j.watres.2010.04.022.
- Huggett D, Khan I, Foran C, Schlenk D. 2003. Determination of beta-adrenergic receptor blocking pharmaceuticals in United States wastewater effluent. Environ Pollut. 121:199–205. doi:10.1016/S0269-7491(02)00226-9.
- Hurtado C, Domínguez C, Pérez-Babace L, Cañameras N, Comas J, Bayona J. 2016. Estimate of uptake and translocation of emerging organic contaminants from irrigation water concentration in lettuce grown under controlled conditions. J Hazard Mater. 305:139–148. doi:10.1016/j.jhazmat.2015.11.039.
- Kasprzyk-Hordern B, Dinsdale R, Guwy A. 2009. The removal of pharmaceuticals, personal care products, endocrine disruptors and illicit drugs during wastewater treatment and its impact on the quality of receiving waters. Water Res. 43:363–380. doi:10.1016/j.watres.2008.10.047.
- Khan M, Cheema S, Anum S, Niazi N, Azam M, Bashir S, Ashraf I, Qadri R. 2020. Phytoremediation of agricultural pollutants. In: Shmaefsky B, editor. Phytoremediation, in-situ applications. Cham: Springer International Publishing; p. 27–81.
- Lei Y, Langenhoff A, Bruning H, Rijnaarts H. 2021. Sorption of micropollutants on selected constructed wetland support matrices. Chemosphere. 275:130050. doi:10.1016/j.chemosphere.2021.130050.
- Luo Y, Guo W, Ngo H, Nghiem L, Hai F, Zhang J, Liang S, Wang X. 2014. A review on the occurrence of micropollutants in the aquatic environment and their fate and removal during wastewater treatment. Sci Total Environ. 473–474:619–641. doi:10.1016/j.scitotenv.2013.12.065.
- Lv T, Zhang Y, Casas ME, Carvalho PN, Arias CA, Bester K, Brix H. 2016. Phytoremediation of imazalil and tebuconazole by four emergent wetland plant species in hydroponic medium. Chemosphere. 148:459–466. doi:10.1016/j.chemosphere.2016.01.064.
- Morasch B, Bonvin F, Reiser H, Grandjean D, De Alencastro L, Perazzolo C, Chèvre N, Kohn T. 2010. Occurrence and fate of micropollutants in the Vidy Bay of Lake Geneva, Switzerland. Part II: micropollutant removal between wastewater and raw drinking water. Environ Toxicol Chem. 29(8):1658–1668. doi:10.1002/etc.222.
- Pilon-Smits E. 2005. Phytoremediation. Annu Rev Plant Biol. 56:15–39. doi:10.1146/annurev.arplant.56.032604.144214.
- Pruden A, Pei R, Storteboom H, Carlson K. 2006. Antibiotic resistance genes as emerging contaminants: studies in northern Colorado. Environ Sci Technol. 40:7445–7450. doi:10.1021/es060413l.
- Schnoor J. 1997. Phytoremediation. Technology evaluation report TE-98-01. Iowa (IA): Ground-Water Remediation Technologies Analysis Center. https://clu-in.org/download/toolkit/phyto_e.pdf
- Schröder P, Collins C. 2002. Conjugating enzymes involved in xenobiotic metabolism of organic xenobiotics in plants. Int J Phytorem. 4:247–265. doi:10.1080/15226510208500086.
- Schwarzenbach RP, Escher BI, Fenner K, Hofstetter TB, Johnson CA, Von Gunten U, Wehrli B. 2006. The challenge of micropollutants in aquatic systems. Science. 313(5790):1072–1077. doi:10.1126/science.1127291.
- Ternes T. 1998. Occurrence of drugs in German sewage treatment plants and rivers. Water Res. 32:3245–3260. doi:10.1016/S0043-1354(98)00099-2.
- Thomas K, Hilton M. 2004. The occurrence of selected human pharmaceutical compounds in UK estuaries. Mar Pollut Bull. 49(5–6):436–444. doi:10.1016/j.marpolbul.2004.02.028.
- Tran N, Reinhard M, Gin K. 2018. Occurrence and fate of emerging contaminants in municipal wastewater treatment plants from different geographical regions-a review. Water Res. 133:182–207. doi:10.1016/j.watres.2017.12.029.
- Trapp S, Karlson U. 2001. Aspects of phytoremediation of organic pollutants. J Soils Sediments. 1:37–43. doi:10.1065/jss2001.04.007.
- Trapp S, Zambrano K, Kusk K, Karlson U. 2000. A phytotoxicity test using transpiration of willows. Arch Environ Contam Toxicol. 39(2):154–160. doi:10.1007/s002440010091.
- Vymazal J. 2011. Plants used in constructed wetlands with horizontal subsurface flow: a review. Hydrobiologia. 674(1):133–156. doi:10.1007/s10750-011-0738-9.
- Vymazal J. 2013. Emergent plants used in free water surface constructed wetlands: a review. Ecol Eng. 61:582–592. doi:10.1016/j.ecoleng.2013.06.023.
- Zhang Y, Lv T, Carvalho P, Arias C, Chen Z, Brix H. 2016. Removal of the pharmaceuticals ibuprofen and iohexol by four wetland plant species in hydroponic culture: plant uptake and microbial degradation. Environ Sci Pollut Res Int. 23(3):2890–2898. doi:10.1007/s11356-015-5552-x.