ABSTRACT
Many different pulsed electromagnetic field (PEMF) devises have been clinically used to stimulate healing processes, but many procedures are still without supporting basic research data. The aim of this study was to investigate a new modified pulsed electromagnetic field therapy: electromagnetic transduction therapy (EMTT). EMTT is technically based on high-intensive PEMFs with a magnetic field strength between 80 and 150 mT. The effect of EMTT for a 10-min session three times a week on human bone marrow mesenchymal stem cells (MSCs) was evaluated by assessing cell viability, gene expression of bone regenerative factors and VEGF-A (vascular endothelial growth factor) secretion after 7 and 14 days of treatment. No negative or toxic effects of EMTT on MSCs in vitro were observed in the applied test frame. The VEGF-ELISA at day 7 of EMTT treatment with 80 mT showed a significant higher VEGF concentration compared to untreated control group. In conclusion, high-intensive electromagnetic impulses showed no harmful effects on MSC cultures in our study. The enhancement of the proangiogenic factor VEGF in MSCs on day 7 indicates a substantial role in cell-stimulating effect of EMTT. Further in vitro and in vivo studies should differentiate specific stimulating and regenerating effects of EMTT impulses in soft tissue engineering. Specific electromagnetic characteristics have to be determined to optimize electromagnetic treatment options in orthopedic surgery and traumatology and soft tissue treatment options.
introduction
Short-acting electromagnetic impulses are increasingly used for non-invasive treatment of soft tissue injuries, musculoskeletal disorder and degeneration without any ionizing or thermal effect (Rubik Citation1997). Previous in vitro and in vivo studies have shown that biophysical stimulation with pulsed electromagnetic field (PEMF) plays a significant role in bone tissue by stimulating cell proliferation and extracellular matrix production and inhibiting inflammatory activities (Fu et al. Citation2014; Ongaro et al. Citation2014). The Food and Drug Administration (FDA) has approved PEMF as an effective and a safe therapy for the following health and body conditions in respective years: 1979 for the healing of fractures, 1998 for muscle stimulations and urinary problems, 2004 for cervical fusion patients, 2006 for the treatment of depression and anxiety, 2011 for the treatment of brain cancer and finally for osteogenesis stimulation, bone fractures, and non-unions (Bassett Citation1989; Waldorff et al. Citation2017). At that time, the FDA suggested that stimulation of PEMF requires a minimum of 10 h per day. Recent in vitro trials have approved the effectiveness of significant shorter treatment durations per day by stimulation of osteoblast and MSC growth, increased ALP activity (Li et al., Citation2007) and activating mTOR pathways, leading to transcription of growth factors such as BMP-4 (Patterson et al. Citation2006). Until now, a large variety of protocols exist for the use of PEMF.
In the last decade, different PEMF devices and technologies were designed, but most clinical trials failed to prove the efficacy in bone healing. The magnetic power of a single electromagnetic impulse measured in millitesla (mT), the fast increasing time of the impulse and the impulse frequency are the most important parameters to create biological effects. shows the oscillating circuit of a EMTT device. Electromagnetic field strength is probably the most important parameter of PEMF therapy besides stimulation duration. The relevant physical parameter is called effective transduction power (kT/sec). Electromagnetic transduction therapy (EMTT) is a promising new form of electromagnetic treatment using high-intensive PEMFs with a magnetic field strength between 80 and 150 mT, effective transduction power >60 kT/s and an oscillating frequency of >100 KHz with exponential decay ensuring a tissue penetration of up to 18 cm. A pulse repetition frequency (PRF) of 3 Hz and single-pulse train duration of 140 µs with no temperature increase in tissue (Krath et al. Citation2017; Varani et al. Citation2021; Waldorff et al. Citation2017). In this context, the extracorporeal magnetotransduction therapy (EMTT) is a promising new form of electromagnetic treatment using high-intensive PEMFs with a central magnetic field strength between 80 and 150 mT and an oscillating frequency of >100 kHz.
In this experimental study, we investigated the effect of EMTT on human bone marrow mesenchymal stem cells (MSCs) simulating normal outpatient treatment by using a treatment of 10 min in duration three times a week over a period of two weeks in total. We analyzed the effect of PEMFs on mRNA expression of the early osteogenic markers collagen I (Owen et al. Citation1990) and alkaline phosphatase (Jaiswal et al. Citation1997) and on later markers, such as osteocalcin (Marom et al. Citation2005), at two defined time points during treatment. In addition, vascular endothelial growth factors (VEGFs) and bone morphogenic proteins (BMPs) are important inductors of osteogenesis and angiogenesis during bone healing procedure (Samee et al. Citation2008; Urist and Strates Citation1971). Bone is a highly vascularized tissue, and reconstructing local microcirculation is prerequisite for effective bone regeneration. Thus, the mRNA expression of VEGF and BMP-2 was analysed as well.
The aim of this study was to evaluate whether short biophysical stimulation with high-intensive electromagnetic impulses by EMTT favors osteogenic stimulation and differentiation of MSCs.
Materials and methods
MSC cultures
The femoral heads were obtained as an excess material during total hip operation by the Department of Orthopedic Surgery and Traumatology, University Schleswig Holstein, Campus Kiel, with informed consent from all patients. The use of human bone material was approved by the local ethical advisory boards. Human MSCs were obtained from femoral heads of four different patients as previously described (Kolbe et al., Citation2011). At the time of surgery, all patients were aged from 65 to 71 years and suffered from primary osteoarthritis of the hip. NSAID intake was recorded only for medication. Bone fragments were washed several times with phosphate buffered saline (PBS; PAA Laboratories, Pasching, Austria) to collect the loosely associated cell fractions. The washing solution containing bone marrow residues was filtered by cell strainer (BD Falcon, Franklin Lakes, NJ) and centrifuged, and cell pellets were cultivated in collagen-coated T-175 tissue culture flasks (Sarstedt, Nümbrecht, Germany) at the density of 2 × 106 cells/cm2 in DMEM/ Ham’s F.12 (Biochrom, Berlin, Germany) supplemented with 20% FBS (PAA Laboratories) and 1% penicillin (PAA Laboratories). The cells were incubated in a humidified atmosphere at 37°C in 5% CO2, and the medium was refreshed every two to three days. When reaching 80–90% confluence, the cells were transferred into the next phase. At phase two, MSCs were stimulated by osteogenic differentiation medium (ODM) (Langenbach and Handschel, Citation2013). ODM consists of DMEM/ Ham’s F.12 (Biochrom) supplemented with 0.1 μM dexamethasone (Sigma-Aldrich, St. Louis, MO), 10 mM β-glycerol phosphate (Sigma-Aldrich), 50 μM ascorbate-2-phosphate (Sigma-Aldrich), 10% FBS, and 1% penicillin. At phase three, MSCs were used for the experiments by seeding 5 × 104 cells/well in 24-well plates. MSCs were cultured for 14 days in 1 ml of ODM culture medium per well. The medium was changed three times a week.
Electromagnetic transduction therapy (EMTT) device
High-intensive pulsed electromagnetic fields were generated by an CE-labeled medical device of class IIa called TheraCell (Guth MediTec, Schechen, Germany). The technical components are integrated in a robust plastic housing with dimensions 127 × 50 × 50 cm. The activated device delivers EMTT impulses with a magnetic field strength between 80 and 150 mT in the middle of a current-carrying twisted loop with a diameter of about 20 cm. The impulse has a release frequency between 1 and 3 Hz and a magnetic field intensity between 64,000 and 120,000 A/m in the middle of the loop. The resonant base frequency is 120 kHz. The temporal change of the magnetic field strength is defined with the effective transduction power which is above 60 kT/s for EMTT devices (Knobloch Citation2022).
Cell treatment
To find the probably most effective intensity configuration, MSCs were treated either with a magnetic field strength of 80 mT (group A) or 150 mT (group B) and compared to the control group (group C), containing untreated MSC cultures. If high-intensive PEMFs with shorter treatment duration also have a positive effect on cell activity like bone regeneration and angiogenesis, this might enhance the practicality of these PEMF devices in clinical practice immensely.
To follow the osteogenic differentiation and cell stimulation in response to EMTT, MSCs were assigned in three treatment groups: treatment with 80 mT EMTT (group A), treatment with 150 mT EMTT (group B) and no treatment with EMTT (group C). During two weeks, cells of groups A and B were treated in total six times for 10 min each time with a frequency of 3 Hz and an intensity of 80 mT or 150 mT ().
Therefore, the 24-well plates of groups A and B were positioned in the center of the TheraCell device loop under standard conditions using a template (). The cell cultures were seeded on day 0 of the treatment protocol in six defined central wells of each 24-well plate to create equal magnetic field conditions for MSCs of groups A and B during treatment. To ensure same environments for all three groups, group C was also taken each time out of the incubator for a 10-min duration.
Cell proliferation assay
Cellular viability and metabolic activity were analyzed using an MTS (3-(4,5-Dimethylthiazol-2-yl)-5-(3-carboxy-methoxyphenyl)-2-(4-sulfophenyl)-2 H-tetrazolium) assay (CellTiter 96® AQueous One Solution Cell Proliferation Assay; Promega Corporation, Fitchburg, WI). The MTS assay was used to measure the metabolic activity of the MSCs on day 1, day 7 and day 14 of the culture. A solution of MTS and ODM in a 1:5 dilution was added to the designated cell cultures of the 24-well plates and incubated for 90 min at 37°C and 5% CO2. Subsequently, the OD was measured by spectrophotometry in six 96-well plates with 120 µL per well, using a multimode microplate reader (Apollo LB 911, Berthold Technologies, Germany) at 490 nm. To yield the correct absorbance, a triplicate set of control wells without cells was used to determine and subtract background absorbance.
Real-time PCR assay
Gene-specific primers for collagen I, alkaline phosphatase (ALPL), osteocalcin (BGLAP), vascular endothelial growth factor A (VEGF A) and bone morphogenic protein-2 (BMP-2) were used to detect the relative mRNA expression of bone regenerative factors. The MSCs were collected from groups A, B and C on days 7 and 14 of treatment. Total RNA was isolated with peqGOLD Total RNA Kit (VWR; Erlangen, Germany) from each cell sample, and the cDNA was synthesized from total RNA by High-Capacity RNA-to-cDNA™-Kit (ThermoFisher, Waltham, MA). 12.5 µl of QuantiTectTM SYBR® Green PCR Master Mix (Qiagen, Hilden, Germany), 2.5 µl of QuantiTectTM SYBR® Green primer assay (Qiagen), 6 µl of RNase free water and 4 µl of cDNA were used for single reaction with the following conditions: 95°C for 5 min followed by 40 cycles at 95°C for 5 s and 60°C for 10 s. The cycle threshold (Ct) was defined as the number of cycles required for the fluorescent signal to cross the threshold in real-time PCR. Determination of relative gene expression was accomplished by using the ΔΔCt method. Gene expression was compared by setting control cultures to 1 (reference value). Primers were ordered as indicated in .
VEGF ELISA assay
To evaluate the temporal pattern of VEGF levels during the culture period, the supernatant obtained from MSCs was analyzed using Human VEGF DuoSet® ELISA (R&D Systems, Wiesbaden, Germany). The supernatant was obtained from 24-well plates on day 7 and day 14 after EMTT treatment of groups A and B before changing the medium. ELISA was performed according to the instruction from the supplier.
Statistical analysis
All data are expressed as mean ± SD. All experiments were performed in triplicate to confirm the reproducibility of the results. Data were analyzed by Student’s t test or one-way ANOVA with appropriate post hoc testing. Comparisons between control and treatment groups were analyzed using one-way ANOVA Tukey’s multiple comparison test. p values <0.05 were considered statistically significant. Analysis was performed using the GraphPad Prism 4 software (GraphPad Software, San Diego, CA).
RESULTS
Cell proliferation assay
The optical density of MTS assay measured by spectrophotometry increased continously from day 1 to day 7 and day 14 in both the untreated control group C and the EMTT-treated groups A (80 mT) and B (150 mT) (). Every group is based on MSCs from four different human donors, with three cell cultures in each case. MTS assay was used to measure the cell viability. The optical density of MTS increased significantly in all three groups from day 1 to day 7 and from day 7 to day 14 (n = 4, p < .05).
Figure 5. Group-specific MSC viability measured by MTS assay (n = 4; group A: 80 mT, group B: 150 mT, group C: control group). The optic absorption of all three groups increased significantly from day 1 to day 7 and day 14. *p < .05, n = 4; Tukey’s multiple comparison test; data are means ± SD.
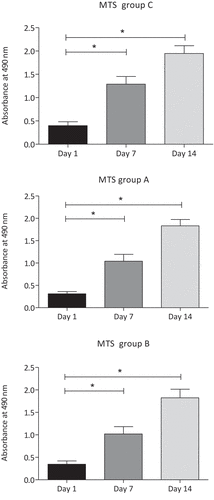
The comparison between group C (control group) and both EMTT-treated groups A and B with different magnetic field strengths shows no significant difference in optical density of MTS assay neither on day 7 nor on day 14 ().
Figure 6. (a) and (b) Show relative cell viability measured by MTS assay of group A (80 mT) and group B (150 mT) compared with control group C (n = 4; group C = 100% cell viability) after 7 and 14 days of culture. No significant difference of optical density is measured between untreated group C and EMTT-stimulated groups A and B; Tukey’s multiple comparison test; data are means ± SD.
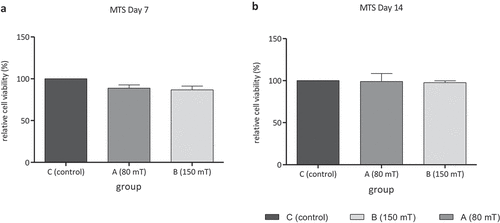
Real-time PCR assay
On day 7 of treatment, the mRNA expression of bone regenerative factors measured by real-time PCR showed no significant differences between untreated and treated groups (). After two weeks of treatment, a tendency towards a higher expression of pro-osteogenic factors is seen in group B treated with 150 mT compared to group C. Expression of collagen I, alkaline phosphatase, osteocalcin, VEGF and BMP-2 after six times of EMTT treatment did not change significantly ().
Figure 7. Relative expression of pro-osteogenic marker collagen I (Col I), alkaline phosphatase (ALPL), osteocalcin (BGLAP), vascular endothelial growth factor A (VEGF A) and bone morphogenic protein-2 (BMP-2) on day 7 (a) and day 14 (b) in group C (control group), group A (80 mT) and group B (150 mT) measured by real-time PCR. No significant difference of relative expression; n = 4, Tukey’s multiple comparison test.
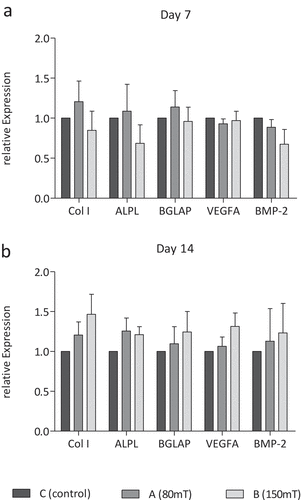
VEGF ELISA assay
The VEGF-ELISA assay on day 7 of treatment showed a significant higher relative VEGF concentration in the supernatant of group A treated with 80 mT EMTT compared with untreated control group C (; p = 0.028; n = 3). The mean VEGF concentration of group A is 43.01% higher than that in group C. Between group A and group B with 150 mT EMTT treatment, no significant difference in VEGF concentration was recognized on day 7. On day 14, no statistically significant differences were measured between these three groups.
DISCUSSION
Since the implementation of pulsed electromagnetic fields in medicine, experimental studies have shown efficacy for the treatment of different orthopedic disorders such as nonunion of fractures, osteoarthritis (Trock et al. Citation1994), osteoporosis (Chang and Chang Citation2003) and osteonecrosis of proximal femur (Bassett et al. Citation1989).
Nevertheless, optimized treatment duration and PEMF strength still remain unclear. These are the main drawbacks of pulsed electromagnetic field therapy in medicine.
Recent studies have clearly identified two effects of pulsed electromagnetic fields. Electroporation describes how magnetic-induced electric effects interact biologically. Electroporation is a process in which brief electrical pulses create transient pores in the plasma membrane that allow nucleic acids to enter the cellular cytoplasm (Kumar et al. Citation2019). The other identified effect of pulsed electromagnetic fields is called piezoelectric effect (Dong et al. Citation2021). The PEMF-induced mechanical effect by piezoelectricity suggests that the effects of PEMF on osteogenesis provide a promising alternative strategy for electrically augmented osteoinduction. The piezoelectric response of collagen-structured piezosensitive tissue by PEMF, which could provide mechanical strain, is particularly interesting as it could deliver local mechanical stimulation to osteogenic cells or other cytoskeletal collagen structures (Dong et al. Citation2021). Both effects, electroporation as well as piezoelectric effects, are related to the magnetic-inducted local electricity. As fast magnetic fields increase as much local electricity is induced. Short increasing time as well as impulse magnetic impulse size are significant physical parameter to create biological relevant reaction . These physical basics were well defined and descried by Maxwell’s equation. Maxwell’s equations are partial differential equations that relate the electric and magnetic fields to each other and to the electric charges and currents. Often, the charges and currents are themselves dependent on the electric and magnetic fields via the Lorentz force equation and the constitutive relations. These all form a set of coupled partial differential equations which are often very difficult to solve: the solutions encompass all the diverse phenomena of classical electromagnetism (Wang Citation2021).
Primatel studies suggested the requirement of PEMF therapy for several hours per day to stimulate tissue or cell cultures biophysically. Newer trials prove effectiveness of PEMF in significant shorter treatment durations per day by activation of early signal transduction pathways (Patterson et al. Citation2006) and stimulation of enzyme activities (Li et al., Citation2007). The underlying working mechanism is based on piezoelectric and electroporation effects. These effects can only be induced if the applied impulses are highly energetic (>10 mT) and are very short acting with a high frequency.
This study investigated the effect of a new form of PEMF devices called TheraCell (Guth MediTec, Schechen, Germany) which generates high-intensive pulsed electromagnetic fields. We hypothesized that EMTT might stimulate healing processes in musculoskeletal disorders. We have chosen EMTT treatment duration of 10 min three times a week for two weeks in total according to standard clinical practice. EMTT has a stronger magnetic field strength and much faster frequency than most PEMF devices. Some reports indicated that high intensity of PEMF may cause harmful effects to humans (Zmyslon Citation2006). However, previous studies have shown that magnetic field stimulation up to 7 tesla and frequencies up to 100 Hz for 1–24 h did not influence cycle progression in tumor cell lines in vitro (Schenck Citation2000; Schiffer et al. Citation2003). Even long-term exposure (4 days) to strong static magnetic fields up to 10 tesla did not affect cell growth rate or cell cycle distribution in hamster ovary cells (Nakahara et al. Citation2002). In the same way, this study with EMTT treatment of human MSCs in vitro between 80 and 150 mT three times a week for 10 min does not affect cell viability measured by MTS assay. The optical density of MTS increased significantly in both EMTT-treated groups and the control group C during the time period of two weeks. Our results suggest no cytotoxic effect of EMTT on MSC cultures. Nevertheless, further experimental and clinical studies need to prove and confirm biological safety. Furthermore, analyses of cancer-related genes and stress response genes are important to evaluate the possible adverse effects or even supportive therapeutic effects based on receptor-modifying effects by electroporation and piezoelectric effects.
Yin-Chih Fu et al. postulate an enhancement of callus formation around necrotic bone in vivo by single-pulsed electromagnetic fields (1 tesla, 30 pulses per day) after 7 and 25 days of treatment (Fu et al. Citation2014). Several in vitro trials document significant enhancement of ALP activity and mRNA expression of pro-osteogenic markers like collagen I, alkaline phosphatase, osteocalcin, vascular endothelial growth factors and bone morphogenic proteins (Fu et al. Citation2014; Li et al., Citation2007). In our in vitro study, we see a tendency toward higher expression of collagen I, alkaline phosphatase and vascular endothelial growth factors A in group A after 14 days of treatment with 80 mT. However, the number of specimens is too small for significant statistical analysis.
Vascular endothelial growth factors (VEGFs) are important inductors of osteogenesis and angiogenesis during bone healing procedure (Samee et al. Citation2008; Urist and Strates Citation1971). Bone is a highly vascularized tissue, and reconstructing local microcirculation is prerequisite for effective bone regeneration (Ferrara et al. Citation2003). Gerber et al. have shown that both vascular invasion and bone formation were suppressed by inhibiting VEGF in 24-day-old mice (Gerber et al. Citation1999). Both BMP-2 and VEGF are involved in chondrogenic and osteogenic differentiation of MSCs as well as enchondral ossification (Xiao et al. Citation2011). MSCs from bone marrow used in this study provide several advantages such as the potential to differentiate to osteoblasts and to support the neovascularization process by the release of proangiogenic factors (Boomsma and Geenen Citation2012; Kolbe et al., Citation2011). In this context, Sun et al. indicate that mesenchymal stem cells in sonication-induced silk fibroin-based hydrogels support the formation of functional endothelial tubes and vascularization networks (Sun et al. Citation2016). Taylor et al. suggested that PEMF enhances the healing of complicated fractures to increase vascularity, rather than to directly enhance osteogenesis (Taylor et al. Citation2006). These findings are supported by our results from analyzing VEGF levels during the culture period on day 7 and day 14. The VEGF-ELISA assay on day 7 of treatment showed a significant higher relative VEGF concentration in the supernatant of group A treated with 80 mT EMTT compared with untreated control group C. The qPCR analyses, however, did not show significant differences between groups A, B and C after 7 and 14 days. This might be caused by too small number of cases. Further in vitro studies with more human subjects have to clarify this. Perhaps, high-intensive PEMF therapy promotes proangiogenic activity of MSCs only in early stage of their development. This might be a reason for still increased VEGF concentrations after 7 days of treatment with already normalized mRNA expression. After 7 days, Goto et al. had shown a significantly higher expression level of angiopoietin-2 and fibroblast growth factor-2 in bone marrow of mice treated with PEMF compared with the control group (Goto et al. Citation2010). They postulated an angiogenesis-promoting function of PEMF. This proangiogenic effect of MSCs may also play a key role in the effective mechanism of EMTT. In this context, several studies have demonstrated that PEMF effectively promotes wound healing (Kim et al. Citation2002) and improves microcirculation in diabetic disorders (Delle Monache et al. Citation2008; Pan et al. Citation2013). Another well-discussed effect was described by Varani et al. (Citation2002, Citation2021). They suggest that PEMFs may act as modulators that are able to enhance adenosine agonist activity. When energy demand rises during inflammation or in hypoxic/ischemic conditions, extracellular adenosine concentration increases. Adenosine functions were mediated by the interaction with four G-protein-coupled receptors (GPCRs), namely A1, A2A, A2B, and A3ARs. This effect was abrogated by using selective A2A and A3AR antagonists, confirming that the observed effect was due to the activation of A2A and A3ARs. The PEMF treatment influences also the cellular growth of bovine chondrocytes and fibroblast-like synoviocytes. Other studies showed that A2A and A3AR stimulation, in the presence of PEMFs, has antiinflammatory effects, decreasing PGE2 release and cyclooxygenase type 2 (COX-2) expression in bovine synovial fibroblast (Varani et al. Citation2021).
The small number of femoral head donors could be a limitation of this trial. Further studies should retrieve more specimens in order to have more different sources of MSC from different patients. Another limitation aspect is the discussion of the unknown specific magnetic field geometry and wave duration and characteristics. It was mentioned that the same six central wells were selected within each 24-well plate to ensure treatment conditions between groups. However, each of these six locations could still have an unknown large range of received PEMF amplitudes due to the variance in the radial direction.
In conclusion, EMTT might be an effective and time-saving device for the treatment of several fundamental orthopedic diseases like fracture healing, early-stage osteonecrosis, tendinous and muscular disorders and potentially even for bony tissue engineering. A magnetic field strength of 80 mT and a frequency of 3 Hz are seemed to be most effective for MSC stimulation.
Acknowledgments
The study was supported by Guth MediTec GmbH (Schechen, Germany) by providing the treatment devices within the study time. The sponsor did not have influence on handling of subjects, data collection, data analysis or preparation of the manuscript.
We thank Sabine Fuchs, chair of the cell laboratory from the Department of Orthopaedic Surgery and Traumatology to provide materials and laboratory access.
Disclosure statement
No potential conflict of interest was reported by the authors.
Additional information
Funding
References
- Bassett, C. A., M. Schink-Ascani, and S. M. Lewis. 1989. Effects of pulsed electromagnetic fields on Steinberg ratings of femoral head osteonecrosis. Clin. Orthop. Relat. Res 246:172–85.
- Bassett, C. A. 1989. Fundamental and practical aspects of therapeutic uses of pulsed electromagnetic fields (PEMFs). Crit. Rev. Biomed. Eng. 17:451–529.
- Boomsma, R. A., and D. L. Geenen. 2012. Mesenchymal stem cells secrete multiple cytokines that promote angiogenesis and have contrasting effects on chemotaxis and apoptosis. PloS one 7:e35685. doi:10.1371/journal.pone.0035685.
- Chang, K., and W. H. Chang. 2003. Pulsed electromagnetic fields prevent osteoporosis in an ovariectomized female rat model: A prostaglandin E2-associated process. Bioelectromagnetics 24:189–98. doi:10.1002/bem.10078.
- Delle Monache, S., R. Alessandro, R. Iorio, G. Gualtieri, and R. Colonna. 2008. Extremely low frequency electromagnetic fields (ELF-EMFs) induce in vitro angiogenesis process in human endothelial cells. Bioelectromagnetics 29:640–48. doi:10.1002/bem.20430.
- Dong, Y., L. Suryani, X. Zhou, P. Muthukumaran, M. Rakshit, F. Yang, F. Wen, A. M. Hassanbhai, K. Parida, D. T. Simon, et al. Synergistic effect of PVDF-coated PCL-TCP scaffolds and pulsed electromagnetic field on osteogenesis. Int J Mol Sci. 2021 June 16. 22:6438. 10.3390/ijms22126438
- Ferrara, N., H. P. Gerber, and J. LeCouter. 2003. The biology of VEGF and its receptors. Nat. Med. 9:669–76. doi:10.1038/nm0603-669.
- Fu, Y. C., C. C. Lin, J. K. Chang, C. H. Chen, I. C. Tai, G. J. Wang, and M. L. Ho. 2014. A novel single pulsed electromagnetic field stimulates osteogenesis of bone marrow mesenchymal stem cells and bone repair. PloS one 9:e91581. doi:10.1371/journal.pone.0091581.
- Gerber, H. P., T. H. Vu, A. M. Ryan, J. Kowalski, Z. Werb, and N. Ferrara. 1999. VEGF couples hypertrophic cartilage remodeling, ossification and angiogenesis during endochondral bone formation. Nat. Med. 5:623–28. doi:10.1038/9467.
- Goto, T., M. Fujioka, M. Ishida, M. Kuribayashi, K. Ueshima, and T. Kubo. 2010. Noninvasive up-regulation of angiopoietin-2 and fibroblast growth factor-2 in bone marrow by pulsed electromagnetic field therapy. J. Orthop. Sci. Off. J. Jpn. Orthop. Ass 15:661–65. doi:10.1007/s00776-010-1510-0.
- Jaiswal, N., S. E. Haynesworth, A. I. Caplan, and S. P. Bruder. 1997. Osteogenic differentiation of purified, culture-expanded human mesenchymal stem cells in vitro. J. Cell. Biochem. 64:295–312. doi:10.1002/(SICI)1097-4644(199702)64:2<295::AID-JCB12>3.0.CO;2-I.
- Kim, S. S., H. J. Shin, D. W. Eom, J. R. Huh, Y. Woo, H. Kim, S. H. Ryu, P. G. Suh, M. J. Kim, and J. Y. Kim, others. 2002. Enhanced expression of neuronal nitric oxide synthase and phospholipase C-gamma1 in regenerating murine neuronal cells by pulsed electromagnetic field Exp. Mol. Med. 34: 53–59. doi: 10.1038/emm.2002.8.
- Knobloch, K. 2022. Bone stimulation 4.0-Combination of EMTT and ESWT in humeral nonunion: A case report. Der Unfallchirurg 125:323–26. Kolbe M, Xiang Z, Dohle E, Tonak M, Kirkpatrick CJ, Fuchs S. 2011. Paracrine effects influenced by cell culture medium and consequences on microvessel-like structures in cocultures of mesenchymal stem cells and outgrowth endothelial cells. Tissue engineering. Part A 17:2199-212. doi:10.1007/s00113-021-01025-3.
- Kolbe, M., Z. Xiang, E. Dohle, M. Tonak, C.J. Kirkpatrick, and S. Fuchs. 2011. Paracrine effects influenced by cell culture medium and consequences on microvessel-like structures in cocultures of mesenchymal stem cells and outgrowth endothelial cells. Tissue Eng Part A 17:2199–212.
- Krath, A., T. Kluter, M. Stukenberg, P. Zielhardt, H. Gollwitzer, N. Harrasser, J. Hausdorf, M. Ringeisen, and L. Gerdesmeyer. 2017. Electromagnetic transduction therapy in non-specific low back pain: A prospective randomised controlled trial. J. Orthop 14:410–15. doi:10.1016/j.jor.2017.06.016.
- Kumar, P., A. Nagarajan, and P. D. Uchil. 2019 July 1. Electroporation. Cold Spring Harb Protoc 2019:153–65. Li JK, Lin JC, Liu HC, Chang WH. 2007. Cytokine release from osteoblasts in response to different intensities of pulsed electromagnetic field stimulation. 10.1101/pdb.top096271
- Langenbach, F., and J. Handschel 2013. Effects of dexamethasone, ascorbic acid and β-glycerophosphate on the osteogenic differentiation of stem cells in vitro. Stem Cell Res Ther 4:117. PMID: 24073831; PMCID: PMC3854789. doi:10.1186/scrt328.
- Li, J.K., J.C. Lin, H.C. Liu, and W.H. Chang 2007. Cytokine release from osteoblasts in response to different intensities of pulsed electromagnetic field stimulation. Electromagn Biol Med 26:153–65. PMID: 17886003. doi:10.1080/15368370701572837.
- Marom, R., I. Shur, R. Solomon, and D. Benayahu. 2005. Characterization of adhesion and differentiation markers of osteogenic marrow stromal cells. J. Cell. Physiol. 202:41–48. doi:10.1002/jcp.20109.
- Nakahara, T., H. Yaguchi, M. Yoshida, and J. Miyakoshi. 2002. Effects of exposure of CHO-K1 cells to a 10-T static magnetic field. Radiology 224:817–22. doi:10.1148/radiol.2243011300.
- Ongaro, A., A. Pellati, L. Bagheri, C. Fortini, S. Setti, and M. De Mattei. 2014. Pulsed electromagnetic fields stimulate osteogenic differentiation in human bone marrow and adipose tissue derived mesenchymal stem cells. Bioelectromagnetics 35:426–36. doi:10.1002/bem.21862.
- Owen, T. A., M. Aronow, V. Shalhoub, L. M. Barone, L. Wilming, M. S. Tassinari, M. B. Kennedy, S. Pockwinse, J. B. Lian, and G. S. Stein. 1990. Progressive development of the rat osteoblast phenotype in vitro: Reciprocal relationships in expression of genes associated with osteoblast proliferation and differentiation during formation of the bone extracellular matrix. J. Cell. Physiol. 143:420–30. doi:10.1002/jcp.1041430304.
- Pan, Y., Y. Dong, W. Hou, Z. Ji, K. Zhi, Z. Yin, H. Wen, and Y. Chen. 2013. Effects of PEMF on microcirculation and angiogenesis in a model of acute hindlimb ischemia in diabetic rats. Bioelectromagnetics 34:180–88. doi:10.1002/bem.21755.
- Patterson, T. E., Y. Sakai, M. D. Grabiner, M. Ibiwoye, R. J. Midura, M. Zborowski, and A. Wolfman. 2006. Exposure of murine cells to pulsed electromagnetic fields rapidly activates the mTOR signaling pathway. Bioelectromagnetics 27:535–44. doi:10.1002/bem.20244.
- Rubik, B. 1997. Bioelectromagnetics & the future of medicine. Administrative. J. Radiol: AR 16:38–46.
- Samee, M., S. Kasugai, H. Kondo, K. Ohya, H. Shimokawa, and S. Kuroda. 2008. Bone morphogenetic protein-2 (BMP-2) and vascular endothelial growth factor (VEGF) transfection to human periosteal cells enhances osteoblast differentiation and bone formation. J. Pharmacol. Sci. 108:18–31. doi:10.1254/jphs.08036FP.
- Schenck, J. F. 2000. Safety of strong, static magnetic fields. J. Magn. Reson. Imaging: JMRI 12:2–19. doi:10.1002/1522-2586(200007)12:1<2::AID-JMRI2>3.0.CO;2-V.
- Schiffer, I. B., W. G. Schreiber, R. Graf, E. M. Schreiber, D. Jung, D. M. Rose, M. Hehn, S. Gebhard, J. Sagemuller, and H. W. Spiess, others. 2003. No influence of magnetic fields on cell cycle progression using conditions relevant for patients during MRI Bioelectromagnetics 24: 241–50. doi: 10.1002/bem.10097.
- Sun, W., A. Motta, Y. Shi, A. Seekamp, H. Schmidt, S. N. Gorb, C. Migliaresi, and S. Fuchs. 2016. Co-culture of outgrowth endothelial cells with human mesenchymal stem cells in silk fibroin hydrogels promotes angiogenesis. Biomed. Mater. 11:035009. doi:10.1088/1748-6041/11/3/035009.
- Taylor, K. F., N. Inoue, B. Rafiee, J. E. Tis, K. A. McHale, and E. Y. Chao. 2006. Effect of pulsed electromagnetic fields on maturation of regenerate bone in a rabbit limb lengthening model. J. Orthop. Res. Offi. Publ. Orthop. Res. Soc 24:2–10. doi:10.1002/jor.20014.
- Trock, D. H., A. J. Bollet, and R. Markoll. 1994. The effect of pulsed electromagnetic fields in the treatment of osteoarthritis of the knee and cervical spine. Report of randomized, double blind, placebo controlled trials. J. Rheumatol. 21:1903–11.
- Urist, M. R., and B. S. Strates. 1971. Bone morphogenetic protein. J. Dent. Res. 50:1392–406. doi:10.1177/00220345710500060601.
- Varani, K., S. Gessi, S. Merighi, V. Iannotta, E. Cattabriga, S. Spisani, R. Cadossi, and P. A. Borea. 2002. Effect of low frequency electromagnetic fields on A2A adenosine receptors in human neutrophils. Br. J. Pharm 136:57–66. doi:10.1038/sj.bjp.0704695.
- Varani, K., F. Vincenzi, S. Pasquini, I. Blo, S. Salati, M. Cadossi, and M. De Mattei. 2021. Pulsed electromagnetic field stimulation in osteogenesis and chondrogenesis: Signaling pathways and therapeutic implications. Int J Mol Sci 22:809. doi:10.3390/ijms22020809.
- Waldorff, E. I., N. Zhang, and J. T. Ryaby. 2017. Pulsed electromagnetic field applications: A corporate perspective. J. Orthop. Transl 9:60–68. doi:10.1016/j.jot.2017.02.006.
- Wang, Z. L. 2021 September 7. From contact electrification to triboelectric nanogenerators. Rep. Prog. Phys. 84: 10.1088/1361-6633/ac0a50
- Xiao, C., H. Zhou, G. Liu, P. Zhang, Y. Fu, P. Gu, H. Hou, T. Tang, and X. Fan. 2011. Bone marrow stromal cells with a combined expression of BMP-2 and VEGF-165 enhanced bone regeneration. Biomed. Mater. 6:015013. doi:10.1088/1748-6041/6/1/015013.
- Zmyslon, M. 2006. Biophysical mechanisms of electromagnetic fields interaction and health effects. Med Pr 57:29–39.