ABSTRACT
Glomeruloid vascular proliferation (GVP) is a diagnostic hallmark and links to aggressive behavior, therapy resistance and poor prognosis in glioblastoma (GBM). It lacks clinical approaches to predict and monitor its formation and dynamic change. Yet the mechanism of GVPs also remains largely unknown. Using an in situ GBM xenograft mouse model, combined clinical MRI images of pre-surgery tumor and pathological investigation, we demonstrated that the inhibition of tissue factor (TF) decreased GVPs in Mouse GBM xenograft model. TF shRNA reduced microvascular area and diameter, other than bevacizumab. TF dominantly functions via PAR2/HB-EGF-dependent activation under hypoxia in endothelial cells (ECs), resulting in a reduction of GVPs and cancer cells invasion. TF expression strongly correlated to GVPs and microvascular area (MVA) in GBM specimens from 56 patients, which could be quantitatively evaluated in an advanced MRI images system in 33 GBM patients. This study presented an approach to assess GVPs that could be served as a MRI imaging biomarker in GBM and uncovered a molecular mechanism of GVPs.
Introduction
Glioblastoma (GBM) is the most common and lethal brain tumor. The median survival is only 12 to 15 months.Citation1 Aggressive progression of GBM is associated with severe hypoxia,Citation2 large areas of necrosis and extensive hyperpermeable vasculature.Citation3,Citation4 The angiogenesis in GBM has a unique feature termed glomeruloid vascular proliferations (GVPs) that are consisted of multiple layers of endothelial cells (ECs) with a potential activity for proliferation, pericytes and smooth muscle cells to form numerous enlarged vessel bodies. It is an important diagnostic criterion for GBM that linked to aggressive behavior and poor prognosis.Citation5-7 Emerging evidence indicates that tumors with intensive GVPs are more resistant to anti-VEGF therapy than these with predominant classic angiogenesis.Citation8 Bevacizumab, an inhibitor of VEGFR tyrosine kinase, could reduce tumor growth by inhibiting tumor vascularization in GBM. The formation of a plexus composed of enlarged GVPs with the massive necrosis and hypoxia is believed as a major cause for relapse after anti-VEGF therapy.Citation9,Citation10 Thus, the mechanism of those GVPs formation remains poorly defined. Therefore, to illustrate molecular pathways that involved in the hypoxia-driven GVPs becomes a preference. The development of methods to evaluate and monitor its occurring and dynamic changes will bring tremendous benefits to the future anti-angiogenic therapy in GBM.
It has been long-time known that tissue factor (TF), a major initiator of coagulation-dependent protease activated receptors (PAR) signaling, is substantially induced by hypoxia in cancers including glioma.Citation11 The levels of TF expression in glioma correlated with the histological grade of malignancy and vascularityCitation12,13 and contributed to tumor growth, metastasis and angiogenesis.Citation14,Citation15 Its overexpression is usually seen in tumor cells around the necrotic foci in glioma,Citation16,Citation17 where GVPs are predominantly located.Citation18 It has been known that TF both directly upregulate VEGF expression and indirectly induces VEGF signaling via protease-activated receptor (PAR)-1 pathway in GBM. The inhibition of TF decreases VEGF levels and microvascular density (MVD).Citation19 Although MVD poorly characterizes the morphometric complexity of GVPs,Citation6,Citation7 thereby TF-initiated signaling plays a very important role in the context of hypoxia-driven GVPs.
It has been widely accepted that GVP serves as a predicative marker for evaluation of tumor aggressiveness, prognosis and therapeutic responses, the precisely assessing TF expression and vascular parameters in GBM in vivo will greatly improve accuracy and efficacy in treatment for GBM. Magnetic resonance imaging (MRI) is a powerful and noninvasive diagnostic imaging tool that allows global assessment of vascular parameters and variable expression of angiogenesis-related proteins.Citation20,Citation21 Dynamic contrast enhanced (DCE)-MRI derived Ktrans provides the information of permeability of vessels,Citation22 while Vessel Size Index (VSI)-MRI presents the average vessel size voxel by voxel.Citation23,Citation24 Combining these two neuroimaging parameters may hold promise in the accurate assessment of both structure and function of vasculature and expression of angiogenesis-related proteins, which may be potential imaging biomarkers for the prediction of prognosis in GBM.
In the present study, we successfully established an image model to measure TF expression and identified TF could be used as a clinical imaging biomarker in GBM and demonstrated that TF induced GVPs by up-regulating PAR-2-dependent HB-EGF pathway in ECs and promote tumor cells invasion. TF expression positively correlated with GVPs, especially microvascular area (MVA).
Results
Silence of TF expression inhibits growth of GBM and capillary permeability in vivo
The primary initiator of coagulation, the transmembrane receptor tissue factor (TF), has gained considerable attention as a determinant of tumor progression. Existing evidences showed TF is highly expressed in more aggressive tumor and promotes tumor growth and angiogenesis.Citation14 To investigate the role of TF in tumor growth in vivo, we successfully established an in situ glioblastoma xenograft model in mice by injecting a GBM cell line with silenced TF expression, which was generated with a lentiviral transduction of shRNAs in U87-MG cells (TFsh group) (Fig. S1), into animal brain (n = 16). The parental cells (Parental group), cells with mock shRNA (NS group) served as negative control. U87-MG glioblastoma cell line has been repeatedly reported to constitutively express TF,Citation19,Citation25 and widely used for studies in tumor angiogenesis and anti-angiogenic therapy.Citation26,Citation27 All animals survived from surgical procedures and developed tumor after two weeks. Brain tumors were identified and monitored with MRI scanning every 5 days (). Images showed that TF shRNA was markedly retarded tumor growth comparing to parental and NS groups () in tumor volume. Ktrans value, a measure of capillary permeability obtained using dynamic contrast-enhanced (DCE) MR perfusion, also was much lower with inhibition of TF (, ). Loss of TF expression significantly prolonged survival period (). It indicated that TF is a major factor to promote tumor growth and capillary permeability in GBM in vivo.
Figure 1. TF inhibition decreased glomeruloid vessel proliferations (GVPs) in Mouse GBM xenograft model. (A) Experimental scheme for in situ GBM xenograft in mice. (B) Quantitative analysis of tumor growth by MR imaging in these 3 groups were analyzed. (C) Representative axial T2WI (top) and DCE-MRI derived Ktrans maps (bottom) of mice with glioma on 20 days post-transplantation. Glioma was the region with high intensity in T2WI. In the corresponding Ktrans maps, red and blue represent relative higher- and lower-value, respectively. (D) Statistic analysis of neovascularization. Changes of Ktrans (DCE-MRI) value in these 3 groups were analyzed. (E) Kaplan-Meier plot for the animal survival rate.
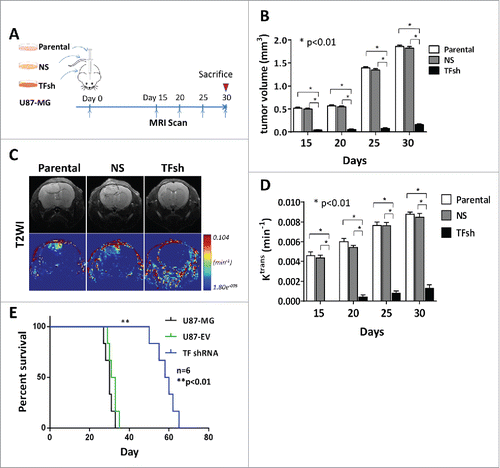
TF promotes GVPs formation via PAR2/HB-EGF signaling in ECs
The presence of morphologically abnormal and dysfunctional vasculature form glomeruloid body structure with potential proliferation is a typical diagnosis for glioblastoma. It creates hypoxic conditions in the tumor, as these vessels are poorly perfused. The hypoxia is a potent inducer for tumor progression through metabolic reprogramming, resistance to cell death, immunosuppression, inflammation and epithelial-mesenchymal transition of cancer cells. It has been known that hypoxia induced PAR-2 signaling in ECs in a TF-dependent manner in cancer cells including GBM cells in vitro.Citation17 To test whether inhibition of TF in cancer cells change GVP formation in vivo, we did series investigations on xenograft tumors. Immunochemistry staining showed that followed TF knockdown, the number of CD34 positive cells were abolished and MVD, MVA and diameter were decreased by 60%, 82% and 65%. In contrast to treatment with silence of TF, a VEGF inhibition drug bevacizumab presented a weaker effect to reduce MVA and vessel diameter (17%, -15%, p < 0.05), but not for MVD (50%) (, ). Although recent studies demonstrate that the inhibitory effect of TF suppression on tumor vascularization was associated with downregulation of VEGF, these above results indicated that TF suppression inhibited GVPs dose not only dependent on VEGF.
Figure 2. Inhibition of TF decreased microvascular parameters and HB-EGF, VEGF signaling. (A) Immunohistochemistry staining (IHC) for CD34 in xenograft tumor. TF suppression decreased GVPs, while VEGF inhibition decreased classic sprout angiogenesis. Scale bar: 100 μm. (B) Statistic analysis for microvascular parameters, including MVD, MVA, diameter in glioma of different groups. (C) IHC and (D) western blot analysis of TF and VEGF, PAR and HB-EGF expression in glioma. β-actin blot served as loading control. (E) IHC for HB-EGF and CD105.
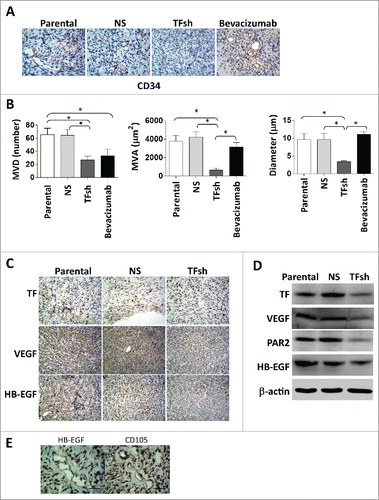
To illustrate that the factors involved the effect of TF in GVPs, we also looked at other known specific proangiogenic growth factors. Our results validated that heparin-binding EGF-like growth factor (HB-EGF), VEGF and PAR2 were down-regulated in xenografic tumors (, ). ECs inside of GVPs expressed either CD105 or HB-EGF (). It suggested that the formation of GVPs in hypoxia condition may dominantly rely on TF-driven HB-EGF pathway.
To further test the TF is key factor to promote ECs proliferation, we cultured ECs with CMs from U87-MG cells with transduced TF shRNAs, parental or NS cells were used as negative controls. U87-CM accelerated ECs wound closure (, ), transwell migration (Fig. S2 A, B), and vascularization in vitro (, ), while CM from cells with TF shRNA failed to have these stimulations. Meanwhile, a significant induction of PAR2 expression was observed in hypoxic ECs comparing to normoxic (data not show). However, we did not see a significant difference among ECs with different CMs cultured under hypoxia condition. Immunofluorescences and western blot analysis showed that TFsh-CM lost the upregulation of HB-EGF expression in ECs (, ). As expected, MTT assay demonstrated that TFsh-CM treatment also decreased ECs proliferation (Fig. S2C).
Figure 3. TF inhibition in tumor cells decreased ECs migration and vascularization in vitro. (A, B) Wound healing assay. Migration of endothelial cells (ECs) cultured with conditioned medium (CM) was measured by closure of scratched wound. Quantification of migration was expressed as the ratio of wound closure at 24 hours to 0 hour (HPF; × 200). (C, D) Tube formation assay of ECs cultured with CMs demonstrated that CM from TF expression cells induced ECs vascularization in vitro. Data represented at mean ± SD from 5 independent experiments. (E) Representative images and (F) protein levels of HB-EGF, PAR2 in ECs. ECs were cultured for 24 h with various CM, including CMs. β-actin served as loading control for western blotting.
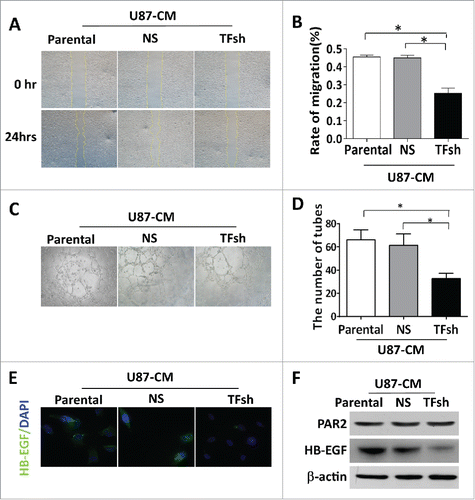
TF enhances GBM invasion to facilitate GVPs formation
GVPs formation is usually a landmark of tumor aggressiveness in GBM. Both of ECs proliferationCitation28 and tumor cell invasionCitation29 are believed to contribute to GVPs. Thereby, our next question is if TF could directly regulate the invasion of GBM cells. MRI images showed clear edge in situ xenograft mice with U87 cell with TF shRNAs, while a diffused boundary was observed in tumors with control cells. HE staining was confirmed that inhibition of TF expression could reduce tumor invasion (, ). Further, Pearson correlation revealed invasive area of U87 glioblastoma correlated well with MVA of GVPs (r = 0.875, p < .001) (). Invasion assay in vitro showed much less number of cells invaded over a Transwell coated with matrigel after TF inhibition (, ). An antibody to against TF in culture medium inhibited wound closure (Fig S3A, B). These data demonstrated that TF suppression not only down-regulated PAR2/HB-EGF in ECs, but also reduced GBM cells invasion during inhibiting GVPs formation.
Figure 4. TF inhibition decreased the tumor cells invasion. (A) H.E. staining exhibited circumscribed boundary of the tumor was more limited comparing to diffusion in control tumors. (B) Quantification of the tumor invasive area. Scale bar: 100 μm. (C) Statistical analysis of relation between MVA of GVPs and invasiveness of tumors. (D, E) Transwell invasive assay. Quantification of invasion was expressed as the number of invasive cells per high-power field (HPF; × 200) and the rate of migration (%), *p < 0.05.
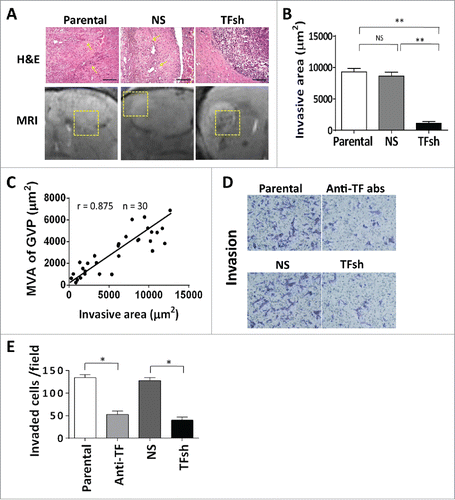
TF expression was correlated to GVPs in patients with GBM
To determine if TF expression is associated with GVPs in patients with GBM, we performed an immunohistochemistry staining of TF and parallel with measurement of GVP parameters in surgical specimens from 56 GBM patients. Highly expressed TF was mainly located close to the necrosis lesions. VEGF mainly expressed at hyper-cellular regions. GVPs with CD34 positive staining were mainly localized in same region with TF, but not overlapped (). While, other than GVPs, another distinct vascular type classic angiogenesis, located similar to the region of VEGF (). Notably, MVA of GVPs was significant positively associated with area of TF expression (r = 0.546, p < .001) (B, ), and MVD showed moderate with TF (r = 0.329, p = .013) (). MVD of classic angiogenesis presented a strong correlation with area of VEGF expression (r = 0.692, p < 0.001) (, ) . ECs in GVPs have a strong staining with CD34, HB-EGF and TF ().
Figure 5. TF expression and GVPs formation in clinical GBM. (A) IHC displayed TF expression locating near necrosis. Serial section reveals prevailing GVPs with CD34 positive staining. VEGF mainly expressed at hyper-cellular regions consistent with classic sprouting-like vessels location. TF and HB-EGF co-expression in ECs of GVPs, Scale bar: 50 μm. (B, C) Pearson correlation analysis revealed percentage area of TF expression at 200 × magnification correlated with microvascular area of GVPs (r = 0.515, p < 0.001) and VEGF exhibits strong correlation with microvessel density of classic sprouting like vessels (r = 0.692, p < 0.001) in 56 GBM patients.
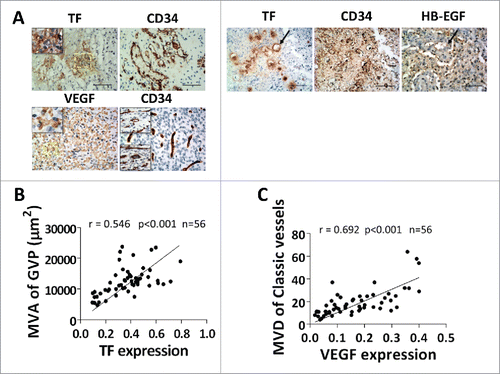
MRI evaluation of TF expression to reflect GVPs in patients with GBM
To translate to molecular image diagnosis in future, we comparably evaluated the MRI images to the levels of TF expression to reflect GVPs in GBM. DCE-MRI and VSI-MRI revealed significant difference consistent with the levels of TF-expression in GBMs. Ktrans map derived from DCE-MRI and VSI map showed high permeability and large microvessel size, consistent with the characteristics of GVPs, in tumor with high level of TF, while vice versa in lower levels of TF (). Ktrans and VSI were calculated using preoperative DCE-MRI and VSI-MRI data from the human GBM participants. As shown, Ktrans hot-spot value and VSI hot-spot value showed well positive correlations with TF expression (r = 0.655, p < .001 for Ktrans; r = 0.556, p < .001 for VSI). These results suggest that Ktrans hot-spot value based on Extended Tofts Linear model and VSI hot-spot value, which can be used to assess functional and structural changes of tumor vascularization, could be surrogate imaging biomarkers for TF expression to reflect GVPs in GBM patients. But, the anatomic MR did not showed differences in characteristics in GBM patients bearing either high or low TF expression.
Figure 6. MRI pre-evaluation of TF expression reflected GVPs in GBM. (A) Different axial MR images showed the lesion of a 52-year-old female patient with TF strongly expressed GBM (top) and the lesion of a 53-year-old male patient with TF weakly expressed GBM (bottom). In both tumors, T1WI after intravenous administration of a contrast agent (CE-T1WI) demonstrates a region of bright signal corresponding to GBM, with necrosis inside tumor. T2WI and T2-FLAIR well differentiated the cyst and peri-tumor edema from tumor parenchyma. In TF strongly expressed GBM, the corresponding slice of VSI map showed relative large microvessel size. The same slice of Ktrans map, revealed high permeability of contrast across the BBB into EES in tumor region. And vice versa in TF weekly expressed GBM. (B) Significant positive correlation between Ktrans hot-spot value and percentage area of TF expression in 20 GBM patients (r = 0.655, p < .001), and (C) between VSI hot-spot value and percentage area of TF expression in 19 GBM patients (r = 0.556, p < .001).
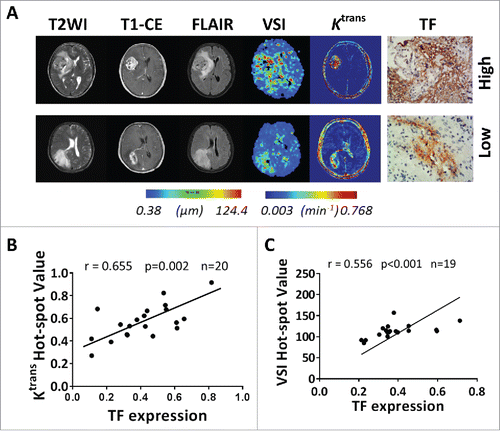
Discussion
GVPs, a hot-spot microvessel density (MVD) and vascular patterns for GBM, have been reported as a pathological landmark to influence prognosis in malignant gliomas. Limited researches focusing on microvascular patterns (MVPs) in human GBM and their impact has linked to aggressive behavior and poor prognosis,Citation5-7 more resistant to anti-VEGF therapy.Citation8 Angiogenesis is vital for tumor growth. In GVPs, the vessels are immature, convoluted with excessive branching, a tortuous or disorganized pattern, with extravasation of blood and plasma proteins. Microvascular proliferation is an established feature of malignant gliomas, in particular, GBM. Establishing diagnostic approaches to monitor or evaluate its development and responses to anti-angiogenesis and other chemotherapy drug will benefit greatly to improvement of survival rate of GBM. Molecular image technology such as MRI has been greatly advanced to integrate molecular mechanism of cancers into clinical diagnosis. The molecular regulation underlying GVPs formation is of central importance.Citation30 Here, we provided in vivo evidence that TF is essential for the formation of GVPs via PAR2/HB-EGF pathway in ECs and cancer cells invasion for Mouse GBM in situ xenograft model. TF expression positively correlated with GVPs parameters in clinical GBM specimens, which can be identified by advanced MRI images. Therefore, our results provided a clinical imaging biomarker to assess the dynamic changes of GVPs in GBM driven by TF expression.
It has been reported that both of ECs proliferationCitation28 and GBM cells invasionCitation29 contribute to GVPs formation. Recent studies demonstrated that TF was cohered with a disorder in balance between anti- and pro-angiogenic factors, which thus favored to increase tumor vascularity.Citation19 In current study, although TF suppression reduced vascularization and downregulated VEGF expression, TF and VEGF might function in separated pathways in the glioma. The treatment with Bevacizumab failed to reproduce same levels of decrease in MVA and diameter induced by TF shRNA. In a recent in vitro study found that hypoxia triggered cancer cells releases TF to up regulate PAR2 and HB-EGF expression in hypoxic vascular ECs.Citation17 Our data confirmed that TF and HB-EGF, a well-known proangiogenic growth factor,Citation31 were co-expressed in ECs that integrated into GVPs in tumors. Emerging with other evidences indicated that TF-enriched microvesicles secreted from hypoxic glioma cells trigger the PAR2/HB-EGF-dependent activation of hypoxic ECs in vitro.Citation17 We further evaluated the secretion of TF is a key step to activate PAR2/HB-EGF signaling in ECs in culture medium containing CM from U87 cells. Meanwhile, CM enhances ECs proliferation, migration, and angiogenesis in vitro. More importantly, it can be the reason why GVPs are resistant to anti-VEGF therapy. It is consistent with findings in clinical GBM tissues that TF is highly expressed in GVPs, while VEGF is dominantly located in classic angiogenesis. The efficacy of anti-VEGF therapy in GBM may be determined by the degree of classic angiogenesis rather than GVPs.Citation8 Additionally, TF suppression decreases the cancer cell invasion lead to minimize the development of GVPs. It has found that anti-VEGF treatment could increase the possibility of invasion of GBM cells into adjacent normal tissues to form satellite lesions.Citation32-34 Therefore, current studies imply that anti-TF or anti-TF combining bevacizumab will be a better choice for glioma therapy.
Owing to the importance of TF in GVPs, TF can serve as an independent biomarker for design therapy and prediction of clinical outcome for GBM patients. Assessment of TF expression and its relative vascular parameters in radiological images will be first step. Advanced MRI, i.e. DCE-MRI (Ktrans) combined with VSI-MRI (VSI) is currently ideal tool for GVPs formation that serves as an indicator for TF expression in GBM patients. Combination of Ktrans and VSI may hold a promise in the non-invasive assessment of TF expression and the dynamic changes of GVPs. Nevertheless, this hypothesis-proofing study still needs to be validated in a large clinical trial in GBM patients.
In summary, this study provides insight into the underlying molecular mechanism of GVPs formation and translation into clinical biomarker guiding therapeutic choices and prediction of clinical outcome. We showed that the TF-dependent activation of PAR2/HB-EGF signaling in ECs in GVPs and cancer cell invasion, and identified the clinical imaging biomarkers to evaluate TF expression and monitor the dynamic changes of GVPs in GBM patients. Our findings suggest that target of TF could overcome antiangiogenic resistance in GBM, and the GVPs image could serve as biomarker for evaluation of therapeutic efficacy.
Materials and methods
Clinical GBM Samples
Eighty-eight consecutive patients with histologically confirmed GBM between July 2012 and April 2015 were enrolled in the current retrospective study (). 32 patients were excluded, 23 of them for either poor imaging quality or insufficient volume of tumor tissue block acquired from pathological specimen bank of Daping Hospital, 6 recurrent GBM, 3 with multiple intracranial lesions. 33 in 56 patients underwent preoperatively intracranial advanced MR imaging. 20 patients were received DCE-MRI, 19 patients were received VSI-MRI scanning, six patients were received both separately at 24 to 48 hours intervals, prior to surgery. Use of archival surgical pathology specimens for immunohistochemical studies was approved by the Institutional Review Board at our institution (IRB #201216), and all patients underwent MRI scanning completed a written consent form.
Table 1. Baseline characteristics of patients.
Table 2. Correlations between expressions of angiogenesis related factors and microvascular parameters.
Cell culture and treatment
A human glioblastoma cell line, U87-MG, and human umbilical vein ECs were obtained from cell bank of Chinese Academy of Sciences (Shanghai, China). The mycoplasma, DNA-Fingerprinting, isozyme and cell vitality have been detected by provider. The cells were cultured in Dulbecco's Modified Eagle's Medium (DMEM) containing 10% and 20% fetal bovine serum. After almost confluence, U87-MG cells were treated with anti-TF antibody (TF9–10H10, Calbiochem) at a final concentration of 10 μg/mL. ECs were treated with various conditional mediums (CMs), 24-hours cultured medium from U87-MG cells including Parental-CM, NS-CM, or TFsh-CM in hypoxia or normoxia, for 24 h.
TF shRNAs and lentiviral transduction
Three shRNAs against TF were designed and inserted into a lentiviral vector pRedPuro, to generate pRedPuro-TFsh-1, pRedPuro-TFsh-2 and pRedPuro-TFsh-3. The target sequences are: pRedPuro-TFsh-1, 5′-ATTAGTCACTTTGAAATGTAAC-3′; pRedPuro-TFsh-2, 5′-CCTTCCTAATATGCTTTACAAT-3′; pRedPuro-TFsh-3, 5′-GGACATTGATCAAAGCCTTCG-3′. Viruses were packaged in a HEK293 cell line and transduced into U87-MG cells following a selection with puromycin for two weeks to generate stable cell lines.
Establishment of in situ mouse xenograft model
The use of laboratory animals was in compliance with the guideline of National Institute of Health. All animal experiments were performed according to a protocol approved by the Animal Use Subcommittee. Fifty-four healthy 4–5-week-old male BALB/c athymic nu/nu weighting 12–14 g (obtained from the Experimental Animal Center of Daping Hospital, Chongqing, China) were anesthetized and placed in a stereotaxic frame (RWD Life Science). The in situ brain glioblastoma model was established as previously described.Citation35 A skull burr hole was drilled 1 mm anterior to the bregma and 3 mm lateral to the midline. 1 × 106 U87-MG cells suspended in 10μL DPBS were injected. Animals were randomly divided into TFsh group (n = 16), NS group (n = 16), Parental group (n = 16), and bevacizumab group (n = 6), bevacizumab were intra-venous injected on 20 days post xenograft. Others were administrated equivalent volume of DPBS.
MR imaging protocol and analysis
Clinical DCE-MRI and VSI-MRI studies were as described previously.Citation36,Citation37 Imaging analysis was performed by two experienced, board-certified neuro-radiologists with more than 7-year working seniority. Average value of measurements by the two neuro-radiologists was employed as the final value. A hand-drawn region of interest (ROI), corresponding to the greatest visualized regions of perfusion (high Ktrans value and VSI value), i.e. hot-spot areas, was achieved as described previously.Citation36,Citation37 For mice, MRI was performed with a Bruker BioSpec 7 T/20 cm system (Bruker). The sequences used to screen the mice for tumors including the following: (1)T2-weighted imaging [RARE, echo time (TE) = 30 ms, repetition time (TR) = 4000 ms, matrix size = 256 × 256, field of view (FOV) = 30 × 30 mm, slice thickness = 0.5 mm, interslice distance = 0 mm, rare factor = 4] (2) T1 maps (FLASH, TE = 1.765 ms, TR = 5.823 ms, matrix size = 128 × 128 , FOV = 35 × 35 mm, slice thickness = 0.5 mm, interslice distance = 0 mm, flip angles of 5°, 10°, 15°, 20° and 25°); (3) DCE-MRI (FLASH, same geometry as T1 maps, TE = 1.765 ms, TR = 5.823 ms, evolution cycles = 100, time resolution = 6.4 s, flip angles of 20°) with contrast agent (Omniscan 0.5 mmol/ml) injected i.v. (0.1 mmol/kg body weight). DCE-MRI data were processed by DCE post-processing software (Omni Kinetics software, GE HealthCare). Extended Tofts linear model (two compartments model) was used to calculate the volume transfer constant(Ktrans) of clinical cases and animal models.
Pathological analysis
Hematoxylin/eosin staining on Paraffin sections were histologically reviewed. For immunochemistry, Vectastain Elite ABC Kit (Vector Laboratories) and DAB Peroxidase Substrate Kit (Vector Laboratories) were used with primary antibodies include anti-human TF, anti-human or anti-mouse VEGF (Santa Cruz Biotechnology Inc.), anti-human or anti-mouse CD34 (Abcam), anti-human or anti-mouse CD105 (Abcam), and anti-human or anti-mouse HB-EGF (Santa Cruz Biotechnology Inc.), followed by Biotinylated goat anti-rabbit and anti-mouse IgG were used as secondary antibody (Vector Laboratories). The diagnosis on surgical specimens were made following the 2007 World Health Organization classification.Citation38 To quantify tumor invasion, five sections from each mouse brain were used, and every five fields per section were captured under the microscope (100 × ) at random. The invasive front of the tumor was defined as the area in which isolated glioma cells were found mixed with surrounding normal brain tissue. To evaluate tumor invasion, we measured the invasive area as the parameter. Analyzing anti-CD34 immunostained sections, we classified vascular patterns as follows: 1. GVPs: clustered vessels ensheathed by connective stroma (); 2. Microvascular sprouting: delicate capillary-like microvessels resembling classic angiogenesis, distributed evenly throughout major parts of vital tumor tissue (). Microvascular parameters, including microvessels density (MVD), microvessels area (MVA), and mean smallest microvessel diameter were defined as previously described on the basis of CD34/CD105-stained profiles. Hot spot regions of whole-section quantitative microvascular analysis, tumor invasive area and protein expressions were performed by using Image-Pro Plus 6.0 (Media Cybernetics).
Western blot analysis
Western blots were performed as described previously.Citation35 Briefly, proteins lysates were extracted from cells cultured in various conditions and glioma tissues. The protein separation on a SDS/PAGE gel and transfer to polyvinylidene difluoride membranes followed standard procedure. TF, HB-EGF, VEGF and β-actin (all from Santa Cruz Biotechnology Inc.) were detected, respectively, with enhanced chemiluminescence methods and quantified with Gel Doc 2000 Imager (Bio-Rad).
Generation of U87-MG cells conditioned medium
The CMs from parental U87-MG cells (Parental-CM), U87-MG cells with Empty pRedPuro (NS-CM), and pRedPuro-TFsh (TFsh-CM) were collected as described previously.Citation35
Immunofluorescent staining
Immunofluorescent staining was performed as described previously.Citation35 Cells grown in a 4-well Lab-Tek chamber slide (Nunc, Thermo Scientific) were fixed with 4% paraformaldehyde at room temperature for 20 min. After blocking with 10% normal goat serum for 10 min, the cells were probed with anti-TF antibody (Santa Cruz Biotechnology Inc.) or anti-HB-EGF antibody. After three times washing with DPBS, the cells were incubated with fluorescent dye-labeled secondary antibody at room temperature for 30 min. Digital images were acquired using a TE200-U Nikon eclipse microscope.
Proliferation assay
The proliferation of U87-MG cells, ECs in various conditions, were determined by 3-(4,5-dimethylthiazol-2-yl)-2,5-diphenyltetrazolium bromide (MTT; Sigma-Aldrich) assay as described previously.Citation39 Briefly, cells were incubated at DMEM as above in a 24-well plate for 24 hours, MTT in Dulbecco's Phosphate Buffered Saline (DPBS) was added at a final concentration of 5 m\g/ml to continue incubation for 4 hours. The reaction was terminated by adding 200 μL dimethyl sulfoxide (DMSO; Sigma–Aldrich) each well. The absorbance was measured at wavelength of 570 nm on a microplate reader.
In vitro migration assay
Wound healing and transwell assays were performed as described previously.Citation40 For wound healing assay, U87-MG cells were cultured in DMEM/F12, while ECs were left treated with various U87-MG cells CMs for 24 h at 37℃ after scratches were created. Wound closure was measured by the distance of the gap under a low power magnification (40 × ). The extent of wound closure was calculated as (Distance of original wound gap − Distance of remaining wound gap)/Distance of original wound gap × 100%. In transwell invasion assay, U87-MG cells (5 × 103) and ECs (2 × 104) were suspended in 100μl serum-free DMEM containing 0.1% bovine serum albumin, seeded in the upper chamber with matrigel coated membrane in 24-well transwell plate (Corning, NY). In the bottom chamber, DMEM with 20% FBS (600μl) were added for U87-MG cells, while CMs and DMEM with 20% FBS at 1:1 ratio for ECs. After overnight incubation at 37℃ with 5% CO2, the transwell membranes were fixed with 4% paraformaldehyde for 10 min in room temperature prior to staining with crystal violet (Sigma Aldrich). The cells on the upper side of membrane and residual matrigel were removed using cotton swabs. Cells invaded onto low side of membrane were counted under microscope at high power fields (100×) and photographed for each chamber and each experiment was replicated 5 times.
In vitro angiogenesis assay
In vitro angiogenesis assay was done as described previously.Citation35 ECs (1 × 104 cells/well) were seeded on a 96-well plate precoated with Matrigel (BD Biosciences). After incubation for 24 h, the formation of tubule-like structures was examined and counted using phase contrast microscopy (Leica, Heerbrugg, Switzerland). The mean number (±SD) of tubules was assessed by counting five random low power (100 × ) fields per well, and each experiment was replicated five times.
Statistical analysis
Data are presented as mean ± standard deviation (SD). Kolmogorov–Smirnov and Levene test were used for assessment of normal distribution and homogeneity of variance. Pearson correlation was performed between TF or VEGF expression and microvascular indexes, DCE parameters, VSI parameters. Animal statistical analysis included t test for two groups, one-way ANOVA or two-way ANOVA for multiple groups. All analyses were performed with SPSS 19.0 (IBM). Two-tailed p values were calculated with the significance level at 0.05.
Disclosure of potential conflicts of interest
No potential conflicts of interest were disclosed.
Supp_mat_1423924_KCBT.zip
Download Zip (2.7 MB)Additional information
Funding
References
- Wen PY, Kesari S. Malignant gliomas in adults. N Engl J Med. 2008;359:492–507. doi:10.1056/NEJMra0708126. PMID:18669428.
- Evans SM, Judy KD, Dunphy I, Jenkins WT, Hwang WT, Nelson PT, Lustig RA, Jenkins K, Magarelli DP, Hahn SM, et al. Hypoxia is important in the biology and aggression of human glial brain tumors. Clin Cancer Res: An official journal of the American Association for Cancer Research. 2004; 10:8177–84. doi:10.1158/1078-0432.CCR-04-1081. PMID:15623592.
- Stupp R, Hegi ME, Mason WP, van den Bent MJ, Taphoorn MJ, Janzer RC, Ludwin SK, Allgeier A, Fisher B, Belanger K, et al. Effects of radiotherapy with concomitant and adjuvant temozolomide versus radiotherapy alone on survival in glioblastoma in a randomised phase III study: 5-year analysis of the EORTC-NCIC trial. Lancet Oncol. 2009;10:459–66. doi:10.1016/S1470-2045(09)70025-7. PMID:19269895.
- Brat DJ, Van Meir EG. Vaso-occlusive and prothrombotic mechanisms associated with tumor hypoxia, necrosis, and accelerated growth in glioblastoma. Lab Invest; A Journal of Technical Methods and Pathology. 2004;84:397–405. doi:10.1038/labinvest.3700070. PMID:14990981.
- Hu LS, Eschbacher JM, Dueck AC, Heiserman JE, Liu S, Karis JP, Smith KA, Shapiro WR, Pinnaduwage DS, Coons SW, et al. Correlations between perfusion MR imaging cerebral blood volume, microvessel quantification, and clinical outcome using stereotactic analysis in recurrent high-grade glioma. AJNR Am J Neuroradiol. 2012;33:69–76. doi:10.3174/ajnr.A2743. PMID:22095961.
- Birner P, Piribauer M, Fischer I, Gatterbauer B, Marosi C, Ambros PF, Ambros IM, Bredel M, Oberhuber G, Rossler K, et al. Vascular patterns in glioblastoma influence clinical outcome and associate with variable expression of angiogenic proteins: evidence for distinct angiogenic subtypes. Brain Pathol. 2003; 13:133–43. doi:10.1111/j.1750-3639.2003.tb00013.x. PMID:12744467.
- Korkolopoulou P, Patsouris E, Kavantzas N, Konstantinidou AE, Christodoulou P, Thomas-Tsagli E, Pananikolaou A, Eftychiadis C, Pavlopoulos PM, Angelidakis D, et al. Prognostic implications of microvessel morphometry in diffuse astrocytic neoplasms. Neuropathol Appl Neurobiol. 2002;28:57–66. doi:10.1046/j.1365-2990.2002.00367.x. PMID:11849564.
- Chen L, Lin ZX, Lin GS, Zhou CF, Chen YP, Wang XF, Zheng ZQ. Classification of microvascular patterns via cluster analysis reveals their prognostic significance in glioblastoma. Human Pathol. 2015;46:120–8. doi:10.1016/j.humpath.2014.10.002. PMID:25455996.
- Rivera LB, Bergers G. CANCER. Tumor angiogenesis, from foe to friend. Science. 2015;349:694–5.
- Hlushchuk R, Riesterer O, Baum O, Wood J, Gruber G, Pruschy M, Djonov V. Tumor recovery by angiogenic switch from sprouting to intussusceptive angiogenesis after treatment with PTK787/ZK222584 or ionizing radiation. Am J Pathol. 2008;173:1173–85. doi:10.2353/ajpath.2008.071131. PMID:18787105.
- Rak J, Yu JL, Luyendyk J, Mackman N. Oncogenes, trousseau syndrome, and cancer-related changes in the coagulome of mice and humans. Cancer Res. 2006;66:10643–6. doi:10.1158/0008-5472.CAN-06-2350. PMID:17108099.
- Hamada K, Kuratsu J, Saitoh Y, Takeshima H, Nishi T, Ushio Y. Expression of tissue factor correlates with grade of malignancy in human glioma. Cancer. 1996;77:1877–83. doi:10.1002/(SICI)1097-0142(19960501)77:9%3c1877::AID-CNCR18%3e3.0.CO;2-X. PMID:8646688.
- Guan M, Jin J, Su B, Liu WW, Lu Y. Tissue factor expression and angiogenesis in human glioma. Clin Biochem. 2002;35:321–5. doi:10.1016/S0009-9120(02)00312-0. PMID:12135696.
- van den Berg YW, Osanto S, Reitsma PH, Versteeg HH. The relationship between tissue factor and cancer progression: insights from bench and bedside. Blood. 2012; 119:924–32. doi:10.1182/blood-2011-06-317685. PMID:22065595.
- Kasthuri RS, Taubman MB, Mackman N. Role of tissue factor in cancer. J Clin Oncol: Official Journal of the American Society of Clinical Oncology 2009;27:4834–8. doi:10.1200/JCO.2009.22.6324. PMID:19738116.
- Magnus N, D'Asti E, Garnier D, Meehan B, Rak J. Brain neoplasms and coagulation. Semin Thromb Hemost. 2013;39:881–95. doi:10.1055/s-0033-1357483. PMID:24108471.
- Svensson KJ, Kucharzewska P, Christianson HC, Skold S, Lofstedt T, Johansson MC, Morgelin M, Bengzon J, Ruf W, Belting M. Hypoxia triggers a proangiogenic pathway involving cancer cell microvesicles and PAR-2-mediated heparin-binding EGF signaling in endothelial cells. Proc Natl Acad Sci U S A. 2011;108:13147–52. doi:10.1073/pnas.1104261108. PMID:21788507.
- Jain RK, di Tomaso E, Duda DG, Loeffler JS, Sorensen AG, Batchelor TT. Angiogenesis in brain tumours. Nat Rev Neurosci. 2007; 8:610–22. doi:10.1038/nrn2175. PMID:17643088.
- Carneiro-Lobo TC, Lima MT, Mariano-Oliveira A, Dutra-Oliveira A, Oba-Shinjo SM, Marie SK, Sogayar MC, Monteiro RQ. Expression of tissue factor signaling pathway elements correlates with the production of vascular endothelial growth factor and interleukin-8 in human astrocytoma patients. Oncol Rep. 2014;31:679–86. doi:10.3892/or.2013.2880. PMID:24297570.
- Jain R, Poisson L, Narang J, Gutman D, Scarpace L, Hwang SN, Holder C, Wintermark M, Colen RR, Kirby J, et al. Genomic mapping and survival prediction in glioblastoma: molecular subclassification strengthened by hemodynamic imaging biomarkers. Radiology. 2013;267:212–20. doi:10.1148/radiol.12120846. PMID:23238158.
- Barajas RF, Jr., Hodgson JG, Chang JS, Vandenberg SR, Yeh RF, Parsa AT, McDermott MW, Berger MS, Dillon WP, Cha S. Glioblastoma multiforme regional genetic and cellular expression patterns: influence on anatomic and physiologic MR imaging. Radiology. 2010;254:564–76. doi:10.1148/radiol.09090663. PMID:20093527.
- Jain R. Measurements of tumor vascular leakiness using DCE in brain tumors: Clinical applications. NMR Biomed. 2013;26:1042–9. doi:10.1002/nbm.2994. PMID:23832526.
- Farrar CT, Kamoun WS, Ley CD, Kim YR, Kwon SJ, Dai G, Rosen BR, di Tomaso E, Jain RK, Sorensen AG. In vivo validation of MRI vessel caliber index measurement methods with intravital optical microscopy in a U87 mouse brain tumor model. Neuro-oncology. 2010;12:341–50. doi:10.1093/neuonc/nop032. PMID:20308312.
- Emblem KE, Mouridsen K, Bjornerud A, Farrar CT, Jennings D, Borra RJ, Wen PY, Ivy P, Batchelor TT, Rosen BR, et al. Vessel architectural imaging identifies cancer patient responders to anti-angiogenic therapy. Nat Med. 2013;19:1178–83. doi:10.1038/nm.3289. PMID:23955713.
- Carneiro-Lobo TC, Konig S, Machado DE, Nasciutti LE, Forni MF, Francischetti IM, Sogayar MC, Monteiro RQ. Ixolaris, a tissue factor inhibitor, blocks primary tumor growth and angiogenesis in a glioblastoma model. J Thromb Haemost: JTH. 2009;7:1855–64. doi:10.1111/j.1538-7836.2009.03553.x. PMID:19624457.
- Jacobs VL, Valdes PA, Hickey WF, De Leo JA. Current review of in vivo GBM rodent models: emphasis on the CNS-1 tumour model. ASN Neuro. 2011;3:e00063. doi:10.1042/AN20110014. PMID:21740400.
- de Vries NA, Beijnen JH, van Tellingen O. High-grade glioma mouse models and their applicability for preclinical testing. Cancer Treat Rev. 2009;35:714–23. doi:10.1016/j.ctrv.2009.08.011. PMID:19767151.
- Sundberg C, Nagy JA, Brown LF, Feng D, Eckelhoefer IA, Manseau EJ, Dvorak AM, Dvorak HF. Glomeruloid microvascular proliferation follows adenoviral vascular permeability factor/vascular endothelial growth factor-164 gene delivery. Am J Pathol. 2001;158:1145–60. doi:10.1016/S0002-9440(10)64062-X. PMID:11238063.
- Dome B, Timar J, Paku S. A novel concept of glomeruloid body formation in experimental cerebral metastases. J Neuropathol Exp Neurol. 2003;62:655–61. doi:10.1093/jnen/62.6.655. PMID:12834110.
- Straume O, Chappuis PO, Salvesen HB, Halvorsen OJ, Haukaas SA, Goffin JR, Begin LR, Foulkes WD, Akslen LA. Prognostic importance of glomeruloid microvascular proliferation indicates an aggressive angiogenic phenotype in human cancers. Cancer Res. 2002;62:6808–11. PMID:12460889.
- Higashiyama S, Abraham JA, Miller J, Fiddes JC, Klagsbrun M. A heparin-binding growth factor secreted by macrophage-like cells that is related to EGF. Sci. 1991;251:936–9. doi:10.1126/science.1840698. PMID:1840698.
- Keunen O, Johansson M, Oudin A, Sanzey M, Rahim SA, Fack F, Thorsen F, Taxt T, Bartos M, Jirik R, et al. Anti-VEGF treatment reduces blood supply and increases tumor cell invasion in glioblastoma. Proc Natl Acad Sci U S A. 2011;108:3749–54. doi:10.1073/pnas.1014480108. PMID:21321221.
- Sennino B, Ishiguro-Oonuma T, Wei Y, Naylor RM, Williamson CW, Bhagwandin V, Tabruyn SP, You WK, Chapman HA, Christensen JG, et al. Suppression of tumor invasion and metastasis by concurrent inhibition of c-Met and VEGF signaling in pancreatic neuroendocrine tumors. Cancer Discov. 2012; 2:270–87. doi:10.1158/2159-8290.CD-11-0240. PMID:22585997.
- Lu KV, Chang JP, Parachoniak CA, Pandika MM, Aghi MK, Meyronet D, Isachenko N, Fouse SD, Phillips JJ, Cheresh DA, et al. VEGF inhibits tumor cell invasion and mesenchymal transition through a MET/VEGFR2 complex. Cancer Cell. 2012;22:21–35. doi:10.1016/j.ccr.2012.05.037. PMID:22789536.
- Chen X, Fang J, Wang S, Liu H, Du X, Chen J, Li X, Yang Y, Zhang B, Zhang W. A new mosaic pattern in glioma vascularization: exogenous endothelial progenitor cells integrating into the vessels containing tumor-derived endothelial cells. Oncotarget. 2014;5:1955–68. doi:10.18632/oncotarget.1885. PMID:24722469.
- Kang HY, Xiao HL, Chen JH, Tan Y, Chen X, Xie T, Fang JQ, Wang S, Yang Y, Zhang WG. Comparison of the Effect of Vessel Size Imaging and Cerebral Blood Volume Derived from Perfusion MR Imaging on Glioma Grading. AJNR Am J Neuroradiol. 2016;37:51–7. doi:10.3174/ajnr.A4477. PMID:26381565.
- Li X, Zhu Y, Kang H, Zhang Y, Liang H, Wang S, Zhang W. Glioma grading by microvascular permeability parameters derived from dynamic contrast-enhanced MRI and intratumoral susceptibility signal on susceptibility weighted imaging. Cancer Imaging: The Official Publication of the International Cancer Imaging Society 2015;15:4. doi:10.1186/s40644-015-0039-z. PMID:25889239.
- Louis DN, Ohgaki H, Wiestler OD, Cavenee WK, Burger PC, Jouvet A, Scheithauer BW, Kleihues P. The 2007 WHO classification of tumours of the central nervous system. Acta Neuropathol. 2007;114:97–109. doi:10.1007/s00401-007-0243-4. PMID:17618441.
- Fang J, Chen X, Zhang L, Chen J, Liang Y, Li X, Xiang J, Wang L, Guo G, Zhang B, et al. P2 × 7R suppression promotes glioma growth through epidermal growth factor receptor signal pathway. Int J Biochem Cell Biol. 2013;45:1109–20. doi:10.1016/j.biocel.2013.03.005.
- Fang J, Chen X, Wang S, Xie T, Du X, Liu H, Li X, Chen J, Zhang B, Liang H, et al. The expression of P2X(7) receptors in EPCs and their potential role in the targeting of EPCs to brain gliomas. Cancer Biol Ther. 2015;16:498–510. doi:10.1080/15384047.2015.1016663.