ABSTRACT
Centromere protein F (CENP-F) is a component of the kinetochore and a regulator of cell cycle progression. CENP-F recruits the dynein transport machinery and orchestrates several cell cycle-specific transport events, including transport of the nucleus, mitochondria and chromosomes. A key regulatory step for several of these functions is likely the G2 phase-specific export of CENP-F from the nucleus to the cytosol, where the cytoplasmic dynein transport machinery resides; however, the molecular mechanism of this process is elusive. Here, we have identified 3 phosphorylation sites within the bipartite classical nuclear localization signal (cNLS) of CENP-F. These sites are specific for cyclin-dependent kinase 1 (Cdk1), which is active in G2 phase. Phosphomimetic mutations of these residues strongly diminish the interaction of the CENP-F cNLS with its nuclear transport receptor karyopherin α. These mutations also diminish nuclear localization of the CENP-F cNLS in cells. Notably, the cNLS is phosphorylated in the −1 position, which is important to orient the adjacent major motif for binding into its pocket on karyopherin α. We propose that localization of CENP-F is regulated by a cNLS, and a nuclear export pathway, resulting in nuclear localization during most of interphase. In G2 phase, the cNLS is weakened by phosphorylation through Cdk1, likely resulting in nuclear export of CENP-F via the still active nuclear export pathway. Once CENP-F resides in the cytosol, it can engage in pathways that are important for cell cycle progression, kinetochore assembly and the faithful segregation of chromosomes into daughter cells.
Introduction
Centromere protein F (CENP-F, also known as mitosin) recruits the large motor protein complex cytoplasmic dynein via adaptor proteinsCitation1,2 to a diverse set of cargo and thus orchestrates several cell cycle-specific transport events in the cell. Cargo that is transported in a CENP-F dependent manner includes the cell nucleus in G2 phase,Citation3-5 mitochondria in anaphaseCitation6 and chromosomes from G2 phase to anaphase, during which CENP-F is assembled into the kinetochore.Citation1,2,7-9 CENP-F is a very large protein (350 kDa) with a complex localization pattern during the cell cycle. It resides in the nucleus during most of interphase and is exported to the cytosol in G2 phase.Citation7,9,10 Export of CENP-F from the nucleus to the cytosol in G2 phase is likely a key regulatory step for the dynein-dependent transport processes it orchestrates,Citation11 as it allows CENP-F to recruit dynein, which also resides in the cytosol. Although this nuclear export is dependent on cyclin-dependent kinase 1 (Cdk1),Citation11 which is active in G2/prophase and is a key regulator for cell cycle progression, the underlying mechanism is unknown.
CENP-F recruits cytoplasmic dynein to its cargo via dynein adaptor proteins NudE and NudEL (nuclear distribution genes E and EL).Citation1,8 Cytoplasmic dynein is the predominant motor complex that mediates transport of cargo toward the minus end of microtubules and therefore orchestrates transport of a diverse set of cargo, including RNA/protein complexes, vesicles and even organelles as large as the nucleus.Citation12 These transport processes are often cell cycle-dependent, and a key regulatory step in these events is the recognition of cargo by adaptor proteins such as CENP-F which link the dynein machinery and its cargo.Citation12 Dynein adaptors loaded with cargo are required to activate the dynein machinery for processive transport. In the absence of cargo, dynein is not capable of processive transport.Citation13-15 Several dynein adaptor proteins recognize distinct sets of cargoes,Citation12 however, it remains largely unknown how cargo selectivity is regulated during the cell cycle to switch between different cargoes.
CENP-F recognizes a diverse set of cargo for dynein-dependent transport, including the cell nucleus, mitochondria and chromosomes.Citation1,3,4,6 Thus, CENP-F has important functions in initial stages of spindle assembly, where the nucleus is oriented with respect to the centrosome in a CENP-F/NudE/NudEL/dynein dependent manner.Citation3-5 Furthermore, CENP-F is assembled into the outer plate of kinetochores starting in G2 phase and during mitosis until anaphase, where it interacts with other kinetochore proteins, such as CENP-E (Centromere protein E)Citation16 and recruits dynein via adaptor proteins to the kinetochore.Citation1,9,17 CENP-F also contains 2 microtubule binding domains at its N- and C-termini and is important for the formation of proper kinetochore-microtubule attachments.Citation17,18 Finally, CENP-F has a role in segregating mitochondria into daughter cells during anaphase.Citation6 These transport events contribute to faithful segregation of chromosomes into daughter cells. However, despite the important functions of the transport events that are initiated by cell cycle-specific nuclear export of CENP-F in G2 phase, the underlying molecular mechanism responsible for its dramatic relocalization is elusive.
The importance of these transport events for physiological functions is also underlined by the fact that missense mutations and overexpression of CENP-F cause human disease. Missense mutations and truncations of CENP-F cause a devastating brain development disease, autosomal recessive primary microcephaly, which is correlated with small brain size and severe cognitive disability.Citation19,20 Other CENP-F mutations cause human ciliopathy.Citation20 Furthermore, CENP-F is overexpressed in many cancers including breast cancers and is associated with high risk and poor prognosis. CENP-F is used as a marker for diagnostic purposes and it is also a target for cancer therapy.Citation21-25 Since farnesylation of the C-terminus of CENP-F is required for its assembly in the kinetochore,Citation26 farnesylation inhibitors are tested for treatment of cancers with elevated expression of CENP-F.Citation27
The cellular localization of CENP-F changes during the cell cycle. During most of interphase, it localizes to the nuclear matrix and is exported to the cytosol in G2 phase. The selective exchange of macromolecules between the nucleus and the cytosol is facilitated by the nuclear pore complex (NPC). Nuclear localization of CENP-F is mediated by a classical bipartite nuclear localization signal (bipartite cNLS) that is located in the C-terminal region of the protein.Citation10 The C-terminal region of CENP-F with the bipartite cNLS is sufficient and essential for nuclear localization, and the cNLS has been mapped to residues 3026–3056.Citation10 Bipartite cNLSs are recognized by the nuclear transport factor karyopherin α, which associates with karyopherin β (for a review seeCitation28). Bipartite cNLSs consist of 2 basic clusters, the minor and major motif, which bind on 2 distinct pockets on the transport receptor karyopherin α, and are spaced by a linker of at least 10 residuesCitation29,30 (for a review seeCitation28). Bipartite cNLSs simultaneously interact with these 2 binding regions on the karyopherin α surface and the major site contributes the larger amount of binding energy.Citation31 The cNLS binds to the karyopherin α binding sites in an extended conformation. Once the cNLS is bound to the karyopherin α, a ternary complex with karyopherin β is formed, which is selectively imported from the cytosol to the nucleus through the NPC in a RanGTP-dependent manner.Citation28 The localization of many proteins is regulated during the cell cycle. A known regulatory mechanism for nuclear import is phosphorylation of residues adjacent to the basic cluster of a bipartite cNLS. Phosphorylation introduces 2 negative charges and is therefore well-suited to silence a cNLS, which consists of 2 positively charged clusters.Citation32
At the G2/prophase transition, CENP-F is exported from the nucleus to the cytosol by an unknown mechanism. Like nuclear import, nuclear export is mediated by specific nuclear export factors. The most versatile nuclear export factor is Crm1 (chromosome region maintenance 1; also called exportin1 or Xpo1)Citation33-36 which recognizes classical nuclear export signals (NESs).Citation37-39 Crm1 recognizes cargo such as ribosomal subunits, mRNPs and many proteins. It has an important role in maintaining distinct proteomes in the nucleus and the cytosol. The permeability barrier of the NPC is inherently leaky; about 20% of all nucleoplasmic or cytoplasmic proteins contain NES and are actively exported from the nucleus by Crm1.Citation40 However, it remains to be established whether CENP-F is a cargo for Crm1.
While the underlying mechanism remains to be established, it is known that G2 phase-specific nuclear export of CENP-F is dependent on mitotic kinase Cdk1, which is active during G2/prophase. Cdk1 inhibitors promote retention of CENP-F in the nucleus during G2 phase.Citation11 However, it is unknown whether Cdk1 controls nuclear export of CENP-F directly or indirectly via an unknown pathway. CENP-F is also heavily phosphorylated during mitosis, but the functional role of the cell cycle-specific phosphorylation of CENP-F is not known.Citation7,41 Cytosolic localization of CENP-F is a prerequisite for any dynein/CENP-F dependent transport processes to occur, as the dynein machinery is in the cytosol. The relocalization of CENP-F to the cytosol is therefore important for cell cycle progression, kinetochore assembly and the faithful segregation of chromosomes into daughter cells.
After nuclear export, CENP-F is first recruited to the surface of the cell nucleus, which is a specific cargo of dynein.Citation3-5,42 Cell cycle-specific recruitment of dynein to the nucleus has emerged as a general and important phenomenon in mitotic progression and brain development, yet the trigger mechanism for dynein recruitment to the nucleus remains to a large degree unknown. CENP-F is recruited to the nuclear envelope by nucleoporin Nup133, which is a protein component of the NPC. In late G2 phase, Nup133 recruits CENP-F to the nuclear envelope, which in turn recruits dynein via NudE and NudEL.Citation3-5 In addition, a second pathway exists that recruits dynein to the NPC through a distinct dynein adaptor protein.Citation42 Both pathways are essential for a fundamental process in brain development.Citation5 During apical nuclear migration, cell nuclei of brain progenitor cells migrate in a dynein-powered movement along microtubule tracks, and enter mitosis once they reach their destination. This process is required for the differentiation of these progenitors into neurons and other cell types and contributes to the formation of the complex architecture of the brain.Citation43 In all cells, dynein recruitment is required for positioning the nucleus in the cell with respect to the centrosome in initial stages of mitosis, and therefore needed for proper spindle assembly.Citation4,42
CENP-F is released from the nuclear envelope in G2/prophase in a mechanism that depends on the small GTPase Rab5,Citation44 and is subsequently targeted to maturing kinetochores, where it resides in the outer plate until anaphase.Citation9 At the kinetochore, CENP-F has a structural role and interacts with other kinetochore proteins, such as CENP-E.Citation16 It also colocalizes with Nup133, which is recruited together with other nups to the kinetochore after mitotic disassembly of the NPC.Citation3,4 Farnesylation of the C-terminus of CENP-F is required for its assembly into the kinetochore.Citation26 Furthermore, CENP-F recruits dynein to the kinetochore via adaptor proteins.Citation1,9,17 It also contains 2 microtubule binding domains and is important to the formation of proper kinetochore-microtubule attachments.Citation17,18 Due to these important roles, silencing of CENP-F by RNAi leads to misaligned chromosomes, premature chromosome decondensation before anaphase onset, and mitotic cell death.Citation17 In anaphase, CENP-F is recruited to mitochondria by the transmembrane GTPase Miro and functions to segregate mitochondria to daughter cells.Citation6 After anaphase, CENP-F is rapidly degraded by the anaphase promoting complex.Citation45 A prerequisite for several of these processes is likely the relocalization of CENP-F into the cytosol,Citation11 where it can recruit cytoplasmic dynein and assemble into the kinetochore. However, the underlying molecular mechanism of G2-specific nuclear export of CENP-F is elusive.
Here, we identify 3 Cdk1-specific phosphorylation sites, which are located within the cNLS of CENP-F. Notably, CENP-F is phosphorylated at the −1 position of the major motif of the bipartite cNLS, which is important for recognition of the cNLS by karyopherin α.Citation32,46,47 To test the effect of phosphorylation on nuclear import, we created phosphomimetic variants of a CENP-F fragment that contained the cNLS. These mutations virtually abolished binding to the transport factor karyopherin α and diminished nuclear localization of the CENP-F fragment in cells. Thus, the cNLS of CENP-F is likely modulated by Cdk1-dependent phosphorylation in G2 phase, slowing down nuclear import rates and diminishing nuclear localization. CENP-F is also actively exported into the cytosol, which would result in cytosolic localization once the cNLS is weakened by phosphorylation. These results yield insights into the molecular mechanism of nuclear export of CENP-F in G2 phase, which is likely an important regulatory step for cell cycle progression. Nuclear export brings CENP-F into vicinity of the cytoplasmic dynein machinery, and likely enables transport events that are essential for differentiation of certain brain progenitor cells and for faithful chromosome segregation.
Results
Identification of putative Cdk1-dependent phosphorylation sites in the bipartite cNLS of CENP-F
CENP-F has a complex localization pattern during the cell cycle. During most of interphase, nuclear localization of CENP-F is mediated by a bipartite cNLS that is located in the C-terminal region of the protein.Citation10 At G2 phase however, CENP-F is exported from the nucleus to the cytosol, and it has been established that this process is dependent on the kinase Cdk1.Citation11 However, the underlying molecular mechanism is elusive, and it is unknown whether Cdk1 acts directly on CENP-F or regulates this process indirectly by an unknown pathway.
Therefore, we analyzed the protein sequence of CENP-F, to identify potential mechanisms that could regulate its cell cycle-specific localization (). With a molar mass of 350kDa, CENP-F is among the largest proteins in eukaryotes. Most of the sequence is predicted to assume a coiled coil structure with high probability (∼0.5) (), apart from a region around residues 1400–1600. Strikingly, the C-terminal region of CENP-F (residues 2987 to 3210) is devoid of coiled coil structure (). Additional secondary structure predictions reveal that this region of the protein (residues 2987–3210) is unstructured, except for 2 very short helices (residues 2992–3001 and 3077–3081). Since the C-terminal region of CENP-F is unstructured, linear signals such as cNLS, cNES, or Cdk1-dependent phosphorylation sites are expected to be accessible for recognition and not buried.
Figure 1. Prediction of structural motifs from the CENP-F sequence. (A) Prediction of coiled coils from the sequence of CENP-F with the COILS server.Citation55 Coiled coil formation probability is plotted versus the residue number. Predictions using window width of 14 (green), 21 (blue) and 28 (red) are shown. Note that no coiled coils are predicted in the C-terminal region of CENP-F, which includes residues 2987–3210. (B) Schematic representation of full-length CENP-F. The predicted coiled coil region is colored red, whereas the predicted disordered C-terminal region is colored blue. Located within the disordered region is a bipartite cNLS (yellow), which was experimentally verifiedCitation10 and 2 overlapping classical NES (orange), which were predicted by the program LocNESCitation38 (Table S1). Note that additional NES are predicted within the coiled coil region, which may or may not be accessible. (C) Schematic representation of the expression construct, a CENP-F fragment of residues 2987–3065, which contains the bipartite cNLS. (D) Schematic representation of predicted Cdk1-dependent phosphorylation sites (black lines) within the bipartite cNLS of CENP-F (yellow). Phosphorylation sites were predicted with iGPS 1.0.Citation48 A black star indicates phosphorylated residues of CENP-F that were identified in extracts from HeLa cells arrested in G2 phase by mass spectrometry.Citation41
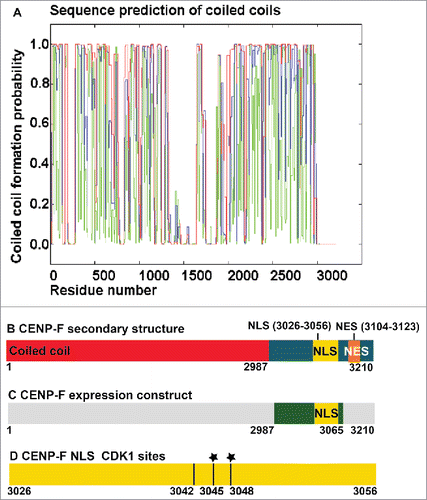
It was previously shown that the C-terminal region is sufficient to target CENP-F to the nucleus. Furthermore, these experiments established that a classical bipartite cNLS in residues 3026–3056 confers nuclear localization.Citation10 Recognition of cNLS by karyopherin α requires these linear sequence motifs to adopt an extended conformation. Consistently, cNLSs are often located in disordered, flexible regions of proteins, which is also true for CENP-F ().
Bipartite cNLS contain 2 clusters of basic residues, which are positively charged. Phosphorylation introduces 2 negative charges and phosphorylation of the −1 position residue adjacent to the major motif (the second basic cluster) by Cdk1 has been described as a mechanism that weakens the cNLS of other proteins with cell cycle-specific localization.Citation32,46,47
We hypothesized that the cNLS of CENP-F is modulated in G2 phase directly by phosphorylation through Cdk1, affecting its cellular localization. Therefore, we used the iGPS softwareCitation48 to predict specific phosphorylation sites for Cdk1 and Cdk2 in the sequence of CENP-F. Both kinases have very similar substrate specificity. Within the bipartite cNLS of CENP-F, 3 Cdk1-specific phosphorylation sites were predicted at residues 3042, 3045 and 3048 (). Of these 3 residues, T3045 and S3048 were experimentally confirmed to be phosphorylated in G2 phase by mass spectrometry analysis of HeLa cell extracts that were arrested in G2 phase.Citation41 The functional role of these Cdk1-specific phosphorylation sites within the cNLS remains to be established. Another Cdk1-specific phosphorylation site is also predicted for residue 3007.
CENP-F shuttles between the cytosol and the nucleus,Citation9,10 and due to its large size of 350 kDa, its translocation from the nucleus to the cytosol needs to be facilitated by an active export pathway, which remains to be identified. Nuclear export pathways generally require recognition of a specific nuclear export signal (NES) by a nuclear export factor. The most widely distributed one is the classical NES,Citation37-39 which is recognized by Crm1.Citation33-36 Classical NESs are present in 20% of all nuclear and cytoplasmic proteins.Citation40 Therefore, we used the program LocNESCitation38 to identify classical NESs in the sequence of CENP-F. Several high-ranking NESs are predicted (Table S1), making it likely that CENP-F is a substrate for nuclear export factor Crm1. It should be noted that prediction of NESs is inherently unreliable, since an NES is a small hydrophobic helix, which will only be recognized by Crm1 if it is accessible and not buried in the core of a protein.Citation37-39 However, the C-terminal region of CENP-F is unstructured, and contains 2 strong predicted NES signals (residues 3104–3123), which are likely accessible for recognition by Crm1 ().
To conclude, we analyzed the protein sequence of CENP-F to develop a hypothesis for the underlying mechanism of its cell-cycle dependent localization. Within the bipartite cNLS of CENP-F, 3 Cdk1-specific phosphorylation sites were identified, which may regulate nuclear localization in a cell cycle-specific manner. Furthermore, several high-scoring classical nuclear export signals were predicted. Classical NES are recognized by the export receptor Crm1. Several of these signals are accessible for recognition, as they are in the unstructured C-terminal region of CENP-F.
The cNLS of CENP-F is a substrate for the G2 phase-specific kinase Cdk1
To test whether the cNLS of CENP-F is indeed a substrate for Cdk1, we performed an in vitro kinase assay. To this end, a C-terminal fragment of CENP-F (residues 2987–3065) that contained the cNLS was purified (, ), and phosphorylated in vitro by the kinase Cdk1/cyclin B. Phosphorylated CENP-F, and a negative control without the kinase, were analyzed on SDS-PAGE (). For phosphorylated CENP-F, a clear upward shift is observed for the band on the gel compared with the negative control, which is typical of phosphorylated proteins and suggests that CENP-F is indeed phosphorylated by Cdk1/cyclin B.
Figure 2. SDS-PAGE analysis of purified CENP-F fragments (residues 2987–3065). Left panel: Purified CENP-F wild-type (wt), CENP-F T3045D/S3048D (2M), and CENP-F T3042D/T3045D/S3048D (3M) fragments are shown in the first 3 lanes. The last lane shows purified karyopherin α (Kα). Molecular weights of standard proteins in kDa are indicated. Right panel: Purified CENP-F wt and S3048D variant (1M). A faint band at 25 kDa represents GST.
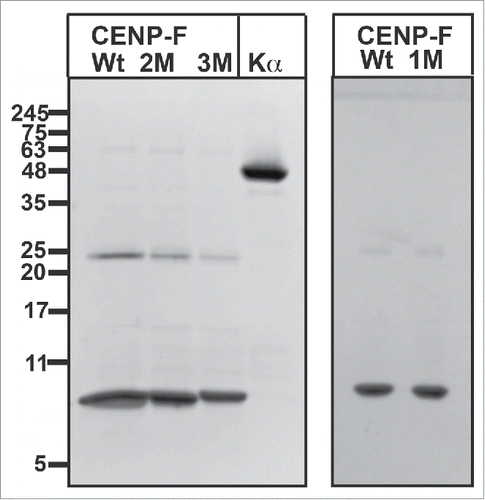
Figure 3. CENP-F is a substrate for Cdk1/cyclin B. (A) Purified CENP-F (residues 2987–3065) was phosphorylated in vitro with the kinase Cdk1/cyclin B. SDS-PAGE analysis of phosphorylated CENP-F (+Cdk1) is shown next to a negative control without Cdk1/cyclin B (-Cdk1). Mass standards are indicated. Note the shift on SDS-PAGE, which suggests that CENP-F is indeed phosphorylated. Cdk1 and cyclin B appear as faint bands at 34 kDa and 60 kDa. Prior to the in vitro kinase assay, the CENP-F fragment () was further purified by gel filtration. (B) Phosphate load of intact phosphorylated CENP-F (from A) as determined by ESI-ion trap mass spectrometry. The mass spectrum shows the +10 to +14 charge states of the intact CENP-F fragment (an 84mer) after phosphorylation. The charge states indicate population of species with 0, 1, 2, 3 and 4 phosphate esters. The intensity is plotted vs. the mass-to-charge ratio (m/z). The peak heights were used to quantify the ratio of each species to total protein amount ().
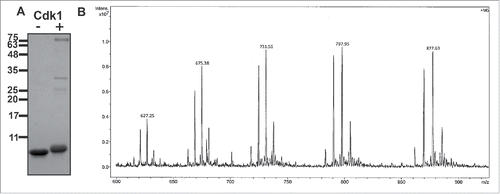
To confirm the number of CENP-F phosphorylation sites, and to determine phosphorylation efficiency, we analyzed the intact in vitro-phosphorylated CENP-F fragment by mass spectrometry (electrospray ionization ion trap mass spectrometry, ESI-ITMS). The observed ESI-ion trap mass spectrum () of the intact protein (an 84mer) indicates 4 phosphorylation sites ().
Table 1. Mass spectrometry analysis of CENP-F 2987–3065 after in vitro phosphorylation with Cdk1/cyclin B.
Phosphoamino acid site location in CENP-F was assessed by examination of tryptic digests by Fourier transform ion cyclotron resonance mass spectrometry (ESI-FTICR MS). Mass spectra (MS1 and MS2) of a CENP-F peptide (residues 3031–3052) were consistent with phosphorylation at T3042, T3045 and S3048, which are the Cdk1-specific phosphorylation sites that are predicted within the cNLS (). In the context of human tissue culture cells arrested in G2 phase, residues 3045 and 3048 of CENP-F are also phosphorylated,Citation41 and our results suggest that these residues are substrates for Cdk1. Mass spectra of a peptide (residues 2995–3016) were consistent with phosphorylation at residue S3007 (), another predicted Cdk1-specific phosphorylation site. Cdk1-specific substrates often contain a proline next to the residue that is phosphorylated,Citation49 as observed for residues T3042, T3045 and S3007. It should be noted that S3048 is a somewhat unusual Cdk1-specific phosphorylation site, as a phenylalanine is located next to the serine.
To conclude, our data show that the cNLS of CENP-F is a substrate for the kinase Cdk1. Our results are consistent with phosphorylation of CENP-F at residues 3007, 3042, 3045 and 3048. Three of these residues are located within the cNLS of CENP-F (, ), opening the possibility that Cdk1 may regulate nuclear import of CENP-F in G2 phase through phosphorylation of the cNLS.
Phosphomimetic mutations of the CENP-F cNLS abolish recognition of the signal by karyopherin α
To test the hypothesis that the cNLS is weakened by phosphorylation through Cdk1, we created phosphomimetic mutations of residues 3042, 3045 and 3048 that are located within the cNLS of CENP-F. Phosphomimetic mutations replace serine or threonine residues with negatively charged aspartate and mimic the effects of phosphorylation, which would introduce 2 negative charges. To probe the effect of phosphomimetic mutations on nuclear import, we purified a C-terminal fragment of CENP-F (residues 2987–3065) (), which contains the cNLS, and corresponding CENP-F fragments with phosphomimetic mutations. To this end, the CENP-F variants S3048D, T3045D/S3048D and T3042D/T3045D/S3048D were created. Notably, the mutant and wild-type protein behaved similar and displayed comparable solubility and yield ().
A cNLS is recognized by nuclear transport factor karyopherin α, which binds with high affinity. To probe the interaction of the cNLS of CENP-F with karyopherin α, we performed binding assays (, Fig. S1). In these experiments, the purified wild-type CENP-F fragment was mixed in equimolar ratio with karyopherin α, and the mixture was separated by analytical size exclusion chromatography (). In addition, the proteins were also analyzed individually (, ). SDS-PAGE analysis of the elution fractions revealed that the wild-type CENP-F fragment with the cNLS interacts strongly with karyopherin α, since both proteins co-elute (, Fig. S1).
Figure 4. Phosphomimetic mutations of the cNLS of CENP-F strongly diminish interaction with nuclear transport factor karyopherin α. (A-I) Purified CENP-F fragments (residues 2987–3065) (0.1 mg) and karyopherin α (0.7 mg) were mixed in 1:1 molar ratio and analyzed by size exclusion chromatography. SDS-PAGE analysis of the peak fractions is shown. Elution volumes are indicated on the bottom, tick marks represent 0.6 mL increments. Positions of molecular weight marker bands and their masses in kDa are indicated on the left. The CENP-F fragments and karyopherin α were also analyzed individually. (A) Karyopherin α. (B) CENP-F wild-type fragment (wt) and karyopherin α. (C) CENP-F S3048D fragment and karyopherin α. (D) CENP-F T3045D/S3048D fragment and karyopherin α. (E) CENP-F T3042D/T3045D/S3048D fragment and karyopherin α. (F) CENP-F wt fragment. (G) CENP-F S3048D fragment. (H) CENP-F T3045D/S3048D fragment. (I) CENP-F T3042D/T3045D/S3048D fragment. An asterisk marks traces of residual GST (glutathione S-transferase).
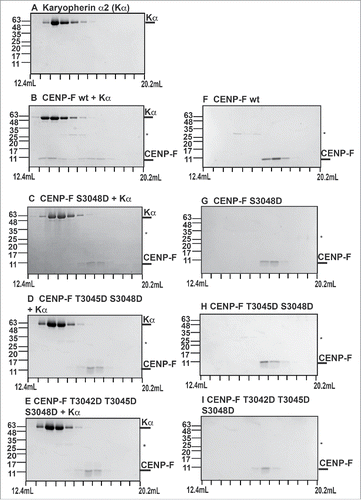
To assess whether Cdk1-specific phosphorylation modulates the interaction between the cNLS of CENP-F and karyopherin α, we also performed these experiments with phosphomimetic variants (, Fig. S1). In these experiments, only a negligible amount of the CENP-F S3048D fragment binds to karyopherin α, and these proteins elute separately (, , , Fig. S1). Similar results are observed for the T3045D/S3048D variant and the T3042D/T3045D/S3048D variant (). These results suggest that phosphorylation of residues 3042, 3045 and 3048 of the cNLS of CENP-F would have a strong effect on the interaction with the nuclear transport factor karyopherin α. It should be noted that nuclear import rates are highly dependent on the affinity of a nuclear localization signal toward a nuclear transport factor,Citation31 and therefore it is expected that these mutations slow down nuclear import.
Affinity is also a function of concentration of the interacting partners. To probe if the lower affinity of karyopherin α to the phosphomimetic variants of the cNLS can be overcome by higher amounts of the substrate, we mixed the wild-type and phosphomimetic variant CENP-F fragments with karyopherin α at molar ratios of 1:1, 1.5:1 and 2:1 CENP-F: karyopherin α. The mixtures were analyzed by size exclusion chromatography (). At all ratios tested, wild-type CENP-F cNLS binds its transport receptor karyopherin α with high affinity, whereas only negligible amounts of the phosphomimetic variants S3048D, T3045D/S3048D and T3042D/T3045D/S3048D interact with karyopherin α.
Figure 5. Comparison of the interaction of karyopherin α with wild-type CENP-F and with phosphomimetic variants of CENP-F. (A-L) Purified CENP-F fragments (residues 2987–3065) and karyopherin α were mixed in 1:1, 1.5:1 and 2:1 ratio respectively and analyzed by size exclusion chromatography. SDS-PAGE analysis of the peak fractions is shown. Elution volumes are indicated on the bottom, tick marks represent 0.6 mL increments. Positions of molecular weight marker bands and their masses in kDa are indicated on the left. (A-C) CENP-F wild-type fragment (wt) and karyopherin α, mixed in 1:1, 1.5:1 and 2:1 molar ratio. (D-F) CENP-F S3048 fragment and karyopherin α. (G-I) CENP-F T3045D/S3048 fragment and karyopherin α. (J-L) CENP-F T3042D/T3045D/S3048D fragment and karyopherin α. An asterisk marks traces of residual GST.
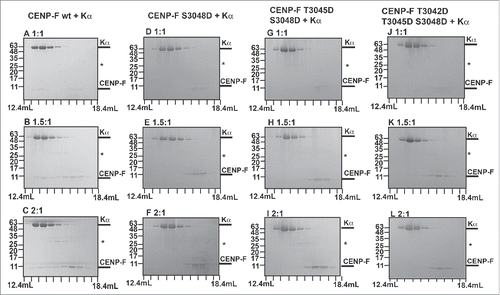
To conclude, while a wild-type CENP-F fragment that includes the cNLS binds to karyopherin α with high affinity, phosphomimetic mutations at positions 3042, 3045 and 3048 strongly diminish the interaction toward karyopherin α. Furthermore, we have confirmed that these sites are substrates for Cdk1 (, ). Combined, these results show that Cdk1 directly phosphorylates the cNLS of CENP-F in G2 phase, thereby weakening the nuclear import signal.
Phosphomimetic mutations of the CENP-F cNLS diminish nuclear localization
Since the phosphomimetic mutations of the cNLS of CENP-F virtually abolish interaction with karyopherin α, the next step was to assess the effect of these mutations on cellular localization of CENP-F. As CENP-F is a very large protein (∼350 kDa) that also undergoes other posttranslational modifications such as farnesylation, these studies were performed with the cNLS of CENP-F rather than the full-length protein, which is a standard approach.Citation47 The CENP-F cNLS (residues 3026–3065) was fused to a fluorescent reporter that was large enough to be excluded from the cell nucleus: an N-terminal GFP-GST-tag (green fluorescent protein-glutathione S-transferase-tag). The resulting GFP-GST-CENP-F-NLS fusion protein was transiently expressed in HeLa cells and imaged by fluorescence microscopy. The location of the cell nucleus was assessed by DAPI stain and cells were classified into 2 categories based on nuclear or cytoplasmic localization of CENP-F. Representative images for cells transfected with the wild-type CENP-F-NLS construct with cytoplasmic () and nuclear () localization are shown. In 4.4% ± 1.2% of total cells, GFP-GST-CENP-F-NLS localized to the cytosol, while 95.6% ± 1.2% of the cells display nuclear localization (, ). The T3045D/S3048D phosphomimetic variant of the CENP-F fragment localizes to the cytosol in twice as many cells (9.6% ± 1.1%). The effect is even more pronounced in the T3042D/T3045D/S3048D variant, where 14.0% ± 3.2% of the cells display cytoplasmic localization of GFP-GST-CENP-F cNLS (, ). To conclude, phosphomimetic mutations of the CENP-F cNLS at the Cdk1-specific phosphorylation sites diminish nuclear localization of the CENP-F cNLS. These results suggest that phosphorylation of the cNLS by Cdk1 would lead to diminished nuclear localization of CENP-F in G2 phase. It should be noted that while the observed effect is significant, the nuclear localization signal is not completely silenced. However, actual phosphorylation (rather than phosphomimetic mutations) may result in a stronger effect on nuclear localization. Furthermore, full-length CENP-F shuttles between the nucleus and the cytosol,Citation9,10 and therefore the steady-state of its localization depends on both nuclear import and export rates. The localization studies were performed with the CENP-F-NLS in the absence of an export pathway. It is expected that in the presence of an active nuclear export pathway, diminished nuclear import rates will result in a more pronounced effect on localization of CENP-F.
Figure 6. Phosphomimetic mutations of the cNLS diminish nuclear localization of CENP-F in cells. GFP-GST-CENP-F-NLS (residues 3026–3065) and its phosphomimetic variants T3045D/S3048D and T3042D/T3045D/S3048D were transiently expressed in HeLa cells. Cells were fixed, stained with DAPI and imaged by fluorescence microscopy. (A, B) Panels show micrographs of GFP-GST-CENP-F-NLS fluorescence (in grayscale), DAPI fluorescence (in grayscale), and an overlay (GFP fluorescence in green, DAPI fluorescence in blue). Scale bar equals 20 μm. (A) Micrographs of a representative wild-type cell with cytoplasmic localization. (B) Micrographs of a representative wild-type cell with nuclear localization. (C) Micrographs were analyzed and cells were classified based on nuclear or cytoplasmic localization of CENP-F. DAPI stain was used to assess the location of the cell nucleus. The table summarizes the percentage of total cells in which GFP-GST-CENP-F-NLS localizes to the cytosol for the wild type and for the phosphomimetic variants. Total cells include cells with nuclear and cytoplasmic localization. Values were averaged from 3 independent data sets. 50–100 cells were analyzed for each data set. Standard deviations were calculated as error estimates. (D) Bar chart of the data from (C).
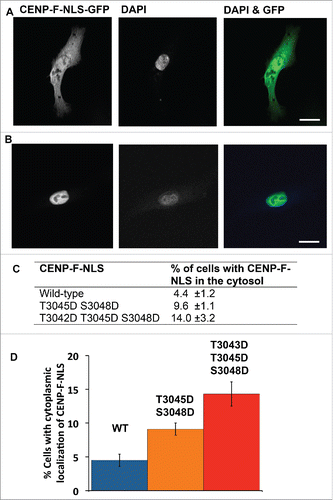
Structural analysis suggests a mechanism for diminished affinity of karyopherin α toward a phosphorylated bipartite cNLS
Several structures of bipartite cNLSs bound to karyopherin α have been determined and thus a structural basis for signal recognition has been established (). To obtain mechanistic insights into how the phosphomimetic mutations of the cNLS of CENP-F abolish binding to karyopherin α, we compared the cNLS of CENP-F with other bipartite cNLSs for which structures in complex with karyopherin α have been established ().Citation28
Table 2. Comparison of the bipartite cNLS of CENP-F with bipartite cNLS/karyopherin α complexes with known structure.
A bipartite cNLS consist of 2 clusters of basic amino acids, the minor and the major motif, separated by a linker region of at least 10 to 12 residues. The consensus sequence of a bipartite cNLS starts with a minor motif KR, followed by a linker of at least 10 residues, and the major motif K(KR)(KRX)(KR), where X can be any residue that is not negatively charged (). The residues of the major motif are denoted P2 – P5. They are recognized by a binding site on karyopherin α that is composed of 4 pockets. The most critical structural determinant is a lysine residue at position P2, which forms a salt bridge with a highly conserved aspartate of karyopherin α.Citation28,31
In the case of the CENP-F bipartite cNLS, the minor motif is KR and the major motif is KKSK (). The linker region between the minor motif and the major motif of the CENP-F cNLS includes 22 residues (). Residues S3048, T3045 and T3042, the Cdk1-dependent phosphorylation sites, are located within the linker region of the cNLS, in the −1, −4 and −7 positions, respectively (). While mutations in the linker often do not result in decreased affinity of the cNLS to karyopherin α, the −1 position in the linker has a special function. For several proteins with cell cycle-specific localization, phosphorylation of the −1 position of the major motif by Cdk1 is a regulatory mechanism that weakens the cNLS and slows down nuclear import.Citation31,32,46,47 In several cases, mutations mimicking the phosphorylation by negatively charged residues, mostly in the −1 position, perturb nuclear localization.Citation46
Our data suggest that a phosphomimetic mutation of the CENP-F cNLS in the −1 position strongly diminishes its affinity for karyopherin α. To obtain mechanistic insights into the regulation of nuclear localization of CENP-F by Cdk1, we compared the cNLS of CENP-F with sequences of cNLS for which structures with karyopherin α bound have been established (). The cNLS of XRCC1 was chosen for comparison with CENP-F, due to the similar major motif, the similarly long linker and the presence of serine in the −1 position.Citation50 In the structure of the karyopherin α/XRCC1 cNLS complex, the minor and major motif are resolved in the electron density, but the linker residues are disordered upstream of the −2 position (). A close-up of the binding pocket of the major motif of the XRCC1 cNLS reveals why serine in the −1 position is important for recognition of the major motif. In the −1, 0 and 1 position, the cNLS makes a rather sharp bend in the binding pocket on the surface of karyopherin α. This sharp bend orients the basic cluster of the P2 - P5 positions so that these residues fit into their respective pockets (). The binding pocket for the residue in the −1 position is rather small, and can only accommodate a small side chain such as serine (, ). The interaction is stabilized by a hydrogen bond between serine 286 from XRCC1 and arginine 238 from karyopherin α (). A larger side chain such as glutamate at this position would likely affect formation of the bend, and compromise binding of the major motif in its site. Furthermore, a negatively charged residue may form salt bridges with positively charged residues such as arginine 238, which may again compromise bending of the cNLS and orientation of the P2 - P5 residues to fit into their pockets. To test if the CENP-F cNLS could potentially bind to karyopherin α in a similar mode as the cNLS of XRCC1, we created a simple structural model by replacing the residues of the XRCC1 cNLS with the residues of the CENP-F cNLS (see methods). Energy minimization or adjustment of rotamers was not performed (). No obvious steric clashes were observed, and therefore it may be possible that the CENP-F cNLS could bind in a similar binding mode, with S3048 in the pocket for the −1 position.
Figure 7. Structural analysis of a bipartite cNLS bound to its nuclear transport receptor karyopherin α. (A) Cartoon representation of the structure of karyopherin α (cyan) with the bipartite cNLS of XRCC1 bound (yellow, shown in stick representation). Note that only the minor and major motif of the bipartite cNLS are ordered in the structure, whereas the linker region is disordered (PDB ID 5E6Q).Citation50 (B-C) Close-up of the binding pocket for the major motif of the cNLS. Karyopherin α is shown in cyan surface presentation and the major motif of the cNLS is shown in yellow, either in stick (B) or in surface (C) representation. The position of the serine residue in −1 position (S268) is indicated (). (D) Close-up of the binding pocket for the major motif of the NLS. Karyopherin α (cyan) and the NLS (yellow) are shown in stick representation. Only residues of karyopherin α that are within 4 Å of the cNLS are shown. S268 at the −1 position is labeled and a hydrogen bond of its carbonyl oxygen with arginine 238 from karyopherin α is indicated. The sequence of the cNLS is shown below and the portion of the sequence that is resolved in the structure is highlighted in yellow. (E) In this simple structural model, the residues from the XRCC1 NLS in D were mutated in COOTCitation57 with the residues from the cNLS of CENP-F. The sequence of the cNLS is shown below and the portion of the sequence that is resolved in the structure is highlighted in yellow. (F) Structure of the major motif of the cNLS of dUTPase bound to karyopherin α, least-squares superimposed with the structure of the phosphomimetic variant of the cNLS with a S11E mutation in the −1 position (PDB IDs 4MZ5 and 4MZ6).Citation46 Karyopherin α (cyan) and the major motif of the wild-type (wt) cNLS (yellow) are shown in stick representation. Only residues of karyopherin α that are within 5 Å of the wt cNLS are shown. The wt cNLS is overlaid with the structure of the phosphomimetic cNLS with an S11E mutation (red, the structure of karyopherin α is not shown for the mutant). Residues 11 and 15 of mutant (red) and wt (black) cNLS are labeled, and hydrogen bonds formed by S11 and R15 of wt cNLS with residues D270 and N228 of karyopherin α are indicated by black dashed lines. The sequence of the major motif of wt (yellow) and phosphomimetic mutant (red) cNLS is shown on the right.
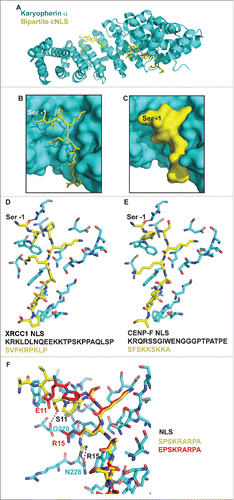
Additional insights into the mechanism of silencing of a cNLS by phosphorylation in the −1 position came from structures of karyopherin α with the major motif of the cNLS of dUTPase which were determined both for the wild-type peptide and the phosphomimetic variant in which serine in the −1 position was replaced by glutamate.Citation46 It should be noted that these structures were determined with a peptide of the major motif of the NLS bound, and therefore the residue in the −1 position represents the N-terminus of the peptide, which may potentially lead to inaccuracy of position compared with a full-length cNLS peptide. shows a structural comparison of the wild-type and phosphomimetic variant of the major cNLS motif bound to karyopherin α. Notably, the phosphomimetic glutamate at the −1 position does not fit well into its binding pocket and forms a new hydrogen bond with D270. The phosphomimetic variant of the major motif is also bent less sharply in its binding pocket compared with the wild-type motif. In addition, the phosphomimetic glutamate forms water-bridged hydrogen bonds with R15 at the 3 position of the major motif of the cNLS. Therefore, R15 is rotated out of its binding pocket and forms a salt bridge with D270 instead of a hydrogen bond with N228. These rearrangements lower the affinity by a factor of 10, as determined by ITC.Citation46 However, a similar rotation of the basic residue at the 3 position is also observed for the native cNLS of XRCC1 (). Nevertheless, the network of salt bridges and hydrogen bonds linking the cNLS with karyopherin α is rearranged in the phosphomimetic variant, compromising proper curvature of the linker and likely affecting the orientation of the basic residues of the major motif. Accordingly, the structure of the phosphomimetic variant of the major motif bound to karyopherin α shows that the cNLS is bent less sharply compared with the wild-type motif ().
To conclude, the cNLS of CENP-F is a substrate for the G2 phase-specific kinase Cdk1 (, and ). Phosphomimetic mutations of the CENP-F cNLS virtually abolish the interaction with karyopherin α, and diminish nuclear localization of the CENP-F cNLS in the context of cells (). Structural comparison with other bipartite cNLS points toward the significance of serine in the −1 position for signal recognition, and notably, one of the Cdk1-specific phosphorylation sites is located in this position (). This position is important as the cNLS makes a sharp bend, which ensures that the large basic residues at the P2 - P5 positions are oriented to fit into their respective pockets. These residues provide most of the binding energy of the cNLS. The pocket for the −1 position only fits a small side chain such as serine. Furthermore, hydrogen bonds and salt bridges are rearranged in phosphorylated or phosphomimetic cNLS to rotate the basic residue at position P3 out of its binding pocket and to prevent tight binding of the −1 position. These structural rearrangements are likely responsible for the low affinity of a cNLS that is phosphorylated in the −1 position toward its transport receptor karyopherin α.
Similar mechanisms for Cdk1-dependent regulation of nuclear transport have been confirmed for several other proteins,Citation32,46,47 making it likely that CENP-F also uses this conserved mechanism to promote nuclear export at G2 phase. Nuclear export of CENP-F at G2 phase is important for initial stages of mitotic spindle assembly and cell cycle progression.Citation4
Discussion
Proposed mechanism for nuclear export of CENP-F in G2 phase
CENP-F is a kinetochore protein that has important functions in cell cycle control, as its knockdown results in misaligned chromosomes, mitotic arrest and mitotic cell death.Citation5,17 Furthermore, it also recruits the dynein machinery via adaptor proteins to a diverse set of cargo, including the cell nucleus, chromosomes and mitochondria.Citation1–6
A regulatory step for many of these processes is likely the export of CENP-F from the nucleus to the cytosol, where the kinetochore and the dynein transport machinery are located. For example, nuclear export of CENP-F is a key regulatory step for recruitment of the dynein machinery to the cell nucleus in G2 phase,Citation11 a pathway that is important to orient the nucleus respective to the centrosome during initial stages of mitotic spindle assembly.Citation4 Here, we provide new insights into the molecular mechanism of cell cycle-specific localization of CENP-F and its regulation by Cdk1.
Nuclear localization of CENP-F during most of interphase is conferred by a classical bipartite cNLS.Citation10 Here, we identified 3 Cdk1-specific phosphorylation sites within the cNLS (,,). Mutations that mimic the effects of phosphorylation of these sites strongly diminish binding of the CENP-F cNLS to its transport receptor karyopherin α (). The phosphomimetic mutations also diminish nuclear localization of the CENP-F cNLS when expressed in cells (). Notably, one of these phosphorylation sites is located in the −1 position of the cNLS adjacent to the major motif, which is an important position for signal recognition of the cNLS (). Structural analysis of bipartite cNLS bound to karyopherin α revealed that at the −1 position, the cNLS makes a sharp bend within its binding pocket on the surface of karyopherin α. This bend orients the basic cluster of the major motif to fit into its binding pocket (). Phosphorylation or phosphomimetic mutation of the −1 position would compromise orientation of the basic cluster toward the pocket, providing a rationale for the observed diminished affinity toward karyopherin α.
Based on our results, we propose a model for the mechanism of G2 phase-specific export of CENP-F. During most of interphase, CENP-F resides in the nucleus,Citation7,9 and nuclear localization is conferred by a bipartite cNLS that is recognized by the transport receptor karyopherin α.Citation10 CENP-F is a protein that shuttles between the nucleus and cytosol,Citation7,9 and due to its large size of 350 kDa, translocation from the nucleus to the cytosol needs to be facilitated by an active nuclear export pathway, however, the responsible nuclear export receptor has not been identified. Based on sequence predictions, the most likely candidate is the nuclear export receptor Crm1, which recognizes classical NES. NES is widespread in the proteome and Crm1 actively exports 20% of all nucleoplasmic and cytoplasmic proteins.Citation40 Notably, 14 NESs with high scores are predicted from the sequence of CENP-F (Table S1).Citation38
The observed nuclear localization of CENP-F during most of interphase would result from faster nuclear import rates compared with the rates of nuclear export (). Nuclear import and export rates are directly correlated with the affinity of a signal toward a transport receptor.Citation31,37 During most of interphase, the cNLS has a strong affinity toward its receptor karyopherin α, which likely correlates with high nuclear import rates, resulting in the observed nuclear localization of CENP-F. Even though an active nuclear export pathway for CENP-F exists, the rate of nuclear export during the cell cycle remains to be established. In G2 phase, Cdk1 would become active and phosphorylate the cNLS of CENP-F in 3 positions. Phosphorylation would weaken the interaction of CENP-F and karyopherin α, thereby slowing down nuclear import. CENP-F would then be exported from the nucleus to the cytosol in G2 phase as the nuclear export pathway would still be active and nuclear export rates would now dominate over nuclear import rates ().
Figure 8. Proposed mechanism for G2 phase-specific nuclear export of CENP-F. (A) During most of interphase, the cNLS has a strong affinity toward its receptor karyopherin α, which likely correlates with high nuclear import rates, resulting in the observed nuclear localization of CENP-F. While it is clear that an active nuclear export pathway for CENP-F exists, the nuclear export receptor and the rate of nuclear export during the cell cycle remains to be established. (B) In G2 phase, Cdk1 would become active and phosphorylate the cNLS of CENP-F. Phosphorylation would weaken the interaction of CENP-F and karyopherin α, thereby slowing down nuclear import. CENP-F would then be exported from the nucleus to the cytosol in G2 phase as the nuclear export pathway would still be active and nuclear export rates would now dominate over nuclear import rates. (C) Nup133/CENP-F pathway for dynein recruitment to the cell nucleus. In G2 phase, CENP-F is recruited to the nucleus by nuclear pore protein Nup133. CENP-F in turn recruits dynein to the nucleus via adaptor proteins NudE/NudEL. This initiates G2 phase-specific transport of the cell nucleus.Citation3-5 This pathway is regulated by Cdk1, which controls G2 phase-specific nuclear export of CENP-F (red arrow).Citation11 (D) Specifically in G2 phase, the cell nucleus is recognized as cargo for dynein-dependent transport toward the minus end of microtubules.
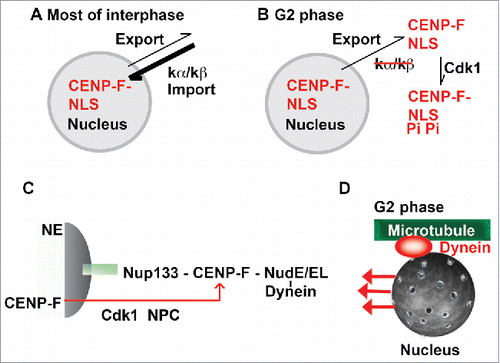
Our data suggest that Cdk1 regulates localization of CENP-F directly by phosphorylating its cNLS rather than by an indirect pathway. The results from our in vitro kinase assay and the subsequent mass spectrometry analysis support that 3 predicted Cdk1 specific phosphorylation sites within the cNLS of CENP-F are substrates for Cdk1. It should be noted that Cdk1-specific substrates often contain a proline next to the residue that is phosphorylated,Citation49 as observed for residues T3042 and T3045. S3048 is a somewhat unusual Cdk1-specific phosphorylation site, as a phenylalanine is located next to the serine (,). Our results are further validated by the fact that T3045 and S3048 are modified by phosphorylation in HeLa cells arrested in G2 phase, although the responsible kinase was not identified in these studies.Citation41 Furthermore, Cdk1 inhibitors block nuclear export of CENP-F in G2 phase and result in its retention in the nucleus, although the underlying mechanism was not established.Citation11 Our results suggest that Cdk1 regulates nuclear import of CENP-F directly through phosphorylation, rather than through an indirect pathway.
Furthermore, we show that phosphomimetic variants of the CENP-F cNLS strongly diminish binding of the cNLS to its transport receptor karyopherin α (). In the context of cells, these phosphomimetic mutations also result in a decreased nuclear localization of the CENP-F cNLS (). While the observed effect is significant, the nuclear localization signal is not completely silenced by phosphomimetic mutations. Notably, actual modification of these residues by phosphorylation adds 2 negative charges and may cause a stronger reduction in affinity compared with the phosphomimetic mutations, which introduce only one negative charge. Furthermore, CENP-F shuttles between the nucleus and the cytosol,Citation9,10 and therefore the steady-state of its localization depends on both nuclear import and nuclear export rates. Our localization studies were performed with a CENP-F fragment that includes the cNLS, however, the construct does not contain a nuclear export signal, unlike full-length CENP-F. It is expected that in the presence of an active nuclear export pathway, diminished nuclear import rates will result in a much more pronounced effect on localization.
It has also been established for several other proteins with cell cycle-specific localization, that phosphorylation by Cdk1 at the −1 position of the major motif of a bipartite cNLS is a regulatory mechanism that decreases the affinity toward karyopherin α by an order of a magnitude and diminishes nuclear import.Citation31,32,46,47 It should be noted that in some cases, change of affinity by 2 orders of magnitude is needed to change localization patterns.Citation31 However, certain proteins in the cell recognize cNLSs and may increase the silencing effect further by recruiting phosphorylated cNLS and masking it from karyopherin α.Citation51 Cell cycle-specific regulation of cellular localization between nucleus and cytosol regulates many important processes in the cell, however, while nuclear import and export signals have been described for many proteins, mechanisms for the regulation of these signals are only established for a few examples, and our results enrich this field, as they provide mechanistic insights into how cell cycle-specific localization of CENP-F is regulated.
We conclude that cell cycle-specific localization of CENP-F is likely regulated by phosphorylation of the cNLS by the G2 phase-specific kinase Cdk1, diminishing nuclear import rates. CENP-F is a shuttling protein and is also actively exported from the nucleus by an unknown pathway, which would lead to nuclear export of CENP-F in G2 phase. Our data provide new insights into a regulatory step that brings CENP-F into the cytosol in G2 phase, where it can engage in pathways that are important for cell cycle control.
Significance of G2 phase-specific nuclear export of CENP-F for cellular transport events
CENP-F can recruit the cytoplasmic dynein machinery to a diverse set of cargo.Citation1 Dynein is the predominant motor complex that mediates transport of cargo toward the minus end of microtubules. Cargo is recognized by adaptor proteins such as CENP-F.Citation12 It remains largely unknown how cargo selectivity is regulated to switch between various types of cargo during the cell cycle and to ensure that the correct cargo is selected at the correct point in time.
CENP-F/dynein dependent transport events are very important for normal physiological function, and therefore it is important to understand how they are regulated. One of the transport pathways that is regulated by nuclear export of CENP-F is the G2 phase-specific recruitment of dynein to the cell nucleusCitation11 which has emerged as an important phenomenon in mitotic progression and brain development.Citation4,5,42 In G2 phase, CENP-F is recruited to the nuclear envelope via nuclear pore protein Nup133 and recruits dynein to the cell nucleus via adaptor proteins NudE and NudEL (Nup133/CENP-F pathway, ).Citation3,4 The nucleus is positioned with respect to the centrosome in a dynein dependent manner along microtubule tracks (). Nuclear positioning is important for initial stages of mitotic spindle assembly in all cell types.Citation4,5,42 In brain progenitor cells, dynein also sustains apical nuclear migration, where the nuclei of these cells migrate, and undergo mitosis once they reach their destination.Citation5 This process ultimately leads to the differentiation of these progenitor cells to neurons and other cell types.
Nuclear export of CENP-F is also a prerequisite for proper timed assembly of CENP-F into the outer plate of the kinetochore, which begins while the nuclear envelope is still intact.Citation9 At kinetochores, CENP-F has important structural rolesCitation16 and also recruits the dynein machinery during chromosome segregation.Citation1,2 Furthermore, CENP-F is important for the formation of microtubule attachments to kinetochores.Citation17,18 These functions of CENP-F contribute to faithful chromosome segregation and regulate cell cycle progression.
Significance of G2 phase-specific nuclear export of CENP-F for therapy of cancers and microcephaly
CENP-F is overexpressed in many cancers, including breast cancer, and is correlated with high risk and poor prognosis.Citation21-25 It is used as a tumor marker for therapeutic purposes and is also a promising cancer target.Citation21-25 Some cancer treatments lower the amount of CENP-F that is assembled into the kinetochore and therefore compensate for the effects of CENP-F overexpression in cancer. Zoledronic acid is such a cancer drug. Zoledronic acid inhibits farnesylation of CENP-F,Citation27 which is required for the assembly of CENP-F into the kinetochore. Consequently, zoledronic acid diminishes the amount of CENP-F that localizes to kinetochores.Citation27 Our results have potential implications for the treatment of cancers that are correlated with overexpression of CENP-F, as they suggest that Cdk1 inhibitor treatment could be beneficial for these cancers. Importantly, CENP-F overexpression can be easily diagnosed by ELISA tests and immunohistology.Citation21,24
Furthermore, recessive CENP-F missense mutants and CENP-F truncations lead to decreased expression of CENP-F and cause a devastating brain development disease in infants, microcephaly, which is correlated with small brain size and cognitive disabilities.Citation19,20 This disease may possibly be caused by defects in nuclear positioning during apical nuclear migration of brain progenitor cells, a fundamental process in brain development.Citation5 The CENP-F/Nup133 pathway for G2 phase-specific dynein recruitment to the cell nucleus is essential for this process, and is regulated by nuclear export of CENP-F in G2 phase.Citation5,11 Therefore, this regulatory mechanism could be targeted by activators of Cdk1 to increase the amount of CENP-F in the cytosol, which may be beneficial for the treatment of microcephaly. These examples show the significance of nuclear import and export pathways of CENP-F for normal physiological functions and for the treatment of devastating human diseases.
Conclusion
Our results provide new insights into the G2 phase-specific export of CENP-F from the nucleus to the cytosol, which is an important regulatory step for nuclear positioning during initial stages of mitosis,Citation11 and important for cell cycle progression and the proper timing of assembly of CENP-F into the kinetochore. CENP-F has a cNLS that promotes nuclear localization during most of interphase. We have identified 3 residues within the cNLS that are substrates for the G2 phase-specific kinase Cdk1. Phosphorylation of the CENP-F cNLS likely diminishes the affinity toward karyopherin α, thus weakening the cNLS and slowing down nuclear import rates in G2 phase. CENP-F is a shuttling protein and is also actively exported from the nucleus. After the cNLS is weakened by phosphorylation, nuclear export rates would dominate over nuclear import, resulting in export of CENP-F into the cytosol. Once CENP-F resides in the cytosol, it can engage in pathways that are important for cell cycle progression, kinetochore assembly and transport processes required for the faithful segregation of chromosomes.
Materials and methods
Cloning, protein expression and purification
Expression constructs of CENP-F (GenBank accession: U19769.1) were generated by amplifying DNA fragments by PCR. Dr. X. Zhu, Institutes for Biological Sciences, Chinese Academy of Sciences, Shanghai, China generously provided a full-length CENP-F construct, which served as a template.Citation10,52 To create N-terminal GST-fusion proteins of CENP-F fragments, these inserts were cloned into the pGEX6p1 vector (GE Healthcare) with the BamHI and XhoI restriction sites. To create N-terminal GFP-GST fusion proteins of CENP-F fragments for localization studies in HeLa cells, the resulting products were cloned into the eGFP-C1 vector (Clontech) with the XhoI and EcoRI restriction sites. GFP-GST-CENP-F (residues 3026–3065) was created by PCR using GFP-GST-CENP-F (residues 2987–3065) as a template and a primer pair that each contained a NotI restriction site. The PCR product was digested with NotI, ligated and transformed using standard methods.
An expression construct of karyopherin α2 (sequence accession NM_002264.3) was created by amplifying a template by PCR. Due to the similarity of the isoforms, this karyopherin α2 construct is referred to in the text as karyopherin α. The insert was cloned into a modified pET28a vector with the NdeI and XhoI restriction sites. In the sequence of the modified pET28a vector, the thrombin cleavage site was replaced by a PreScission protease cleavage site.
Site-directed mutagenesis was performed with the QuikChange Lightning Site-Directed Mutagenesis Kit (Agilent).
Proteins were expressed in E. coli Rosetta 2(DE3)pLysS strain (Novagen). Cells were grown at 37°C to an OD of 0.5 and induced with 0.2 mM IPTG for 3 hours. CENP-F fragments were purified by affinity chromatography with Pierce Glutathione Agarose (Thermo Fisher Scientific) according to the manufacturer's instructions and eluted by overnight proteolytic cleavage on the column with PreScission protease (GE Healthcare). Purified CENP-F fragments (residues 2987–3065) were concentrated to a concentration of 5–6 mg/ml and flash-frozen in liquid nitrogen. Protein concentrations were determined by absorbance of the peptide bond.
Karyopherin α was purified by His6-affinity chromatography, using His-Select nickel affinity gel (Sigma Aldrich) according to the manufacturer's instructions. The His6-tag was removed by overnight proteolytic cleavage with PreScission protease. Subsequently, karyopherin α was further purified by a second step of His6-affinity chromatography. Purified karyopherin α was concentrated to 8 mg/ml and flash-frozen in liquid nitrogen. All steps were performed at 4°C if not otherwise noted. Purified proteins were analyzed by SDS-PAGE on 16% acrylamide gels and stained by Coomassie Blue.
In vitro kinase assay
For in vitro kinase assays, CENP-F (residues 2987–3065) was purified as described above, and further purified by size exclusion chromatography on a Superdex 200 10/300 GL column (GE-Healthcare), using a gel filtration buffer containing 20 mM Tris pH 7.5 at 25°C, 60 mM NaCl and 2 mM DTT. 40 μl CENP-F fragment (at 3.3 mg/ml concentration), 5 μl Cdk1/cyclin B (20 000 units/μl, New England Biolabs), 6 μl 10x PK buffer (0.5 M Tris-HCl, 0.1 M MgCl2, 1 mM EDTA, 20 mM DTT, 0.1% Brij 35, pH 7.5 at 25°C), 3 μl water and 6 μl ATP 10x stock (4 mM in 1x PK buffer) were mixed and incubated for 1 hour at 30°C. 2.5 μl of the reaction was analyzed on SDS-PAGE (16% acrylamide gel) and stained by Coomassie Blue. 6M Guanidinium hydrochloride was added to the remaining sample in a ratio of 1:1 for mass spectrometry analysis. A control reaction without Cdk1/cyclin B was performed. For mass spectrometry analysis, protein was desalted and denatured by microbore RPLC (PLRP300 column). The intact in vitro-phosphorylated CENP-F 84-mer was analyzed by electrospray ionization ion trap mass spectrometry (ESI-ITMS; Bruker Amazon). In addition, CENP-F samples were also treated with trypsin and analyzed. Trypsin digests were desalted with Zip Tips (Millipore). Phosphoamino acid site location in CENP-F was assessed by examination of tryptic digests of the 84mer by electrospray ionization Fourier transform ion cyclotron resonance mass spectrometry (ESI-FTICR MS). Analysis was performed on a Bruker Apex III mass spectrometer (accumulation time 169 μs). The accuracy of masses determined by ESI-FTICR MS is within 0.005 amu. To quantify the ratio of each phosphorylated species respective to the total protein amount, peak heights of the mass spectra were determined.
Analytical size exclusion chromatography
Purified proteins (in a sample volume of 200 μl) were incubated for 30 minutes on ice, filtered (0.2 μM pore size) and cleared by centrifugation for 25 minutes at 16.800 g. The sample was separated by size exclusion chromatography on a Superdex 200 10/300 GL column (GE-Healthcare) at 4 °C. The gel filtration buffer consisted of 20 mM HEPES, pH 7.5, 150 mM NaCl and 2 mM DTT. Analytical gel filtration experiments were performed under identical conditions for all samples. Elution fractions were analyzed by SDS-PAGE and stained by Coomassie Blue.
Localization studies in HeLa cells
HeLa cells were transfected with FuGene (Roche) at a 3.5:1 ratio of μl FuGene to μg DNA, seeded on coverslips after 12 hours and collected after 24–48 hours. Cells were fixed with 2% paraformaldehyde in phosphate buffered saline for 30 min at room temperature, DAPI-stained (double strand DNA was stained using Hoechst 33342, Thermo Fisher Scientific), and coverslips were mounted with Prolong Gold Antifade Mountant (Thermo Fisher Scientific). Images were collected on a Zeiss Axio Imager fluorescence microscope using a 63x oil objective. Micrographs were processed with ImageJCitation53 and Adobe Photoshop.
Sequence predictions, structure analysis and figures
Cdk1- and Cdk2-dependent phosphorylation sites (which have similar specificity) were predicted from the protein sequence of CENP-F with iGPS 1.0.Citation48 The cNLSs were predicted from the protein sequence with cNLS mapper.Citation54 Coiled coils were predicted from the protein sequence with the COILS server.Citation55 Secondary structure prediction was performed with PROFseq, implemented in PredictProtein.Citation56 cNES were predicted with the program LocNES.Citation38
Structure coordinates were analyzed with COOT,Citation57 PyMOL (The PyMOL Molecular Graphics System, Version 1.8 Schrödinger, LLC), and the PISA server.Citation58 Structural models were created by editing existing coordinates in the program COOT.Citation57 Cation-pi interactions were identified with CaPTURE.Citation59 Structure figures were created in PyMOL.
Disclosure of potential conflicts of interest
No potential conflicts of interest were disclosed.
Supplemental_Material.zip
Download Zip (1.7 MB)Acknowledgments
We thank Hyung Bum Kim, Laboratory of Cell Biology, the Rockefeller University, New York for excellent technical support. Furthermore, we thank Dr. John Xue, the Rockefeller University, New York, and Dr. Christof Grewer, Binghamton University, for helpful comments. We thank Dr. Xuelian Zhu, Institutes for Biological Sciences, Chinese Academy of Sciences, Shanghai, China for providing a full-length CENP-F construct. Finally, we thank Dr. Susan Bane, Dr. Brian Callahan, Dr. Christof Grewer and Dr. Susan Flynn at Binghamton University for access to equipment.
Funding
This research was funded by the Research Foundation for the State University of New York and the Department of Chemistry, State University of New York at Binghamton.
References
- Yang ZY, Guo J, Li N, Qian M, Wang SN, Zhu XL. Mitosin/CENP-F is a conserved kinetochore protein subjected to cytoplasmic dynein-mediated poleward transport. Cell Res 2003; 13:275-83; PMID:12974617; https://doi.org/10.1038/sj.cr.7290172
- Liang Y, Yu W, Li Y, Yu L, Zhang Q, Wang F, Yang Z, Du J, Huang Q, Yao X, et al. Nudel modulates kinetochore association and function of cytoplasmic dynein in M phase. Mol Biol Cell 2007; 18:2656-66; PMID:17494871; https://doi.org/10.1091/mbc.E06-04-0345
- Zuccolo M, Alves A, Galy V, Bolhy S, Formstecher E, Racine V, Sibarita JB, Fukagawa T, Shiekhattar R, Yen T, et al. The human Nup107/160 nuclear pore subcomplex contributes to proper kinetochore functions. EMBO J 2007; 26:1853-64; PMID:17363900; https://doi.org/10.1038/sj.emboj.7601642
- Bolhy S, Bouhlel I, Dultz E, Nayak T, Zuccolo M, Gatti X, Vallee R, Ellenberg J, Doye V. A Nup133-dependent NPC-anchored network tethers centrosomes to the nuclear envelope in prophase. J Cell Biol 2011; 192:855-71; PMID:21383080; https://doi.org/10.1083/jcb.201007118
- Hu DJ, Baffet AD, Nayak T, Akhmanova A, Doye V, Vallee RB. Dynein recruitment to nuclear pores activates apical nuclear migration and mitotic entry in brain progenitor cells. Cell 2013; 154:1300-13; PMID:24034252; https://doi.org/10.1016/j.cell.2013.08.024
- Kanfer G, Courtheoux T, Peterka M, Meier S, Soste M, Melnik A, Reis K, Aspenstrom P, Peter M, Picotti P, et al. Mitotic redistribution of the mitochondrial network by Miro and Cenp-F. Nat Comm 2015; 6:8015; https://doi.org/10.1038/ncomms9015
- Zhu X, Mancini MA, Chang KH, Liu CY, Chen CF, Shan B, Jones D, Yang-Feng TL, Lee WH. Characterization of a novel 350-kgdalton nuclear phosphoprotein that is specifically involved in mitotic-phase progression. Mol Cell Biol 1995; 15:5017-29; PMID:7651420; https://doi.org/10.1128/MCB.15.9.5017
- Vergnolle MS, Taylor SS. Cenp-F links kinetochores to Ndel1/Nde1/Lis1/Dynein microtubule motor complexes. Curr Biol 2007; 17:1173-9; PMID:17600710; https://doi.org/10.1016/j.cub.2007.05.077
- Rattner JB, Rao A, Fritzler MJ, Valencia DW, Yen TJ. CENP-F is a ca 400 kDa kinetochore protein that exhibits a cell-cycle dependent localization. Cell Motil Cytoskeleton 1993; 26:214-26; PMID:7904902; https://doi.org/10.1002/cm.970260305
- Zhu X, Chang K-H, He D, Mancini MA, Brinkley WR, Lee WH. The C terminus of mitosin is essential for its nuclear localization, centromere/kinetochore targeting, and dimerization. J Biol Chem 1995; 270:19545-50; PMID:7642639; https://doi.org/10.1074/jbc.270.33.19545
- Baffet A D, Hu D J, Vallee R B. Cdk1 activates pre-mitotic nuclear envelope dynein recruitment and apical nuclear migration in neural stem cells. Dev Cell 2015; 33:703-16; PMID:26051540; https://doi.org/10.1016/j.devcel.2015.04.022
- Cianfrocco MA, DeSantis ME, Leschziner AE, Reck-Peterson SL. Mechanism and regulation of cytoplasmic dynein. Annu Rev Cell Dev Biol 2015; 31:83-108; PMID:26436706; https://doi.org/10.1146/annurev-cellbio-100814-125438
- Splinter D, Razafsky DS, Schlager MA, Serra-Marques A, Grigoriev I, Demmers J, Keijzer N, Jiang K, Poser I, Hyman AA, et al. BICD2, dynactin, and LIS1 cooperate in regulating dynein recruitment to cellular structures. Mol Biol Cell 2012; 23:4226-41; PMID:22956769; https://doi.org/10.1091/mbc.E12-03-0210
- Schlager MA, Serra-Marques A, Grigoriev I, Gumy LF, Esteves da Silva M, Wulf PS, Akhmanova A, Hoogenraad CC. Bicaudal D family adaptor proteins control the velocity of dynein-based movements. Cell Rep 2014; 8:1248-56; PMID:25176647; https://doi.org/10.1016/j.celrep.2014.07.052
- McKenney RJ, Huynh W, Tanenbaum ME, Bhabha G, Vale RD. Activation of cytoplasmic dynein motility by dynactin-cargo adapter complexes. Science 2014; 345:337-41; PMID:25035494; https://doi.org/10.1126/science.1254198
- Chan GKT, Schaar BT, Yen TJ. Characterization of the kinetochore binding domain of CENP-E reveals interactions with the kinetochore proteins CENP-F and hBUBR1. J Cell Biol 1998; 143:49-63; PMID:9763420; https://doi.org/10.1083/jcb.143.1.49
- Yang Z, Guo J, Chen Q, Ding C, Du J, Zhu X. Silencing mitosin induces misaligned chromosomes, premature chromosome decondensation before anaphase onset, and mitotic cell death. Mol Cell Biol 2005; 25:4062-74; PMID:15870278; https://doi.org/10.1128/MCB.25.10.4062-4074.2005
- Musinipally V, Howes S, Alushin GM, Nogales E. The microtubule binding properties of CENP-E' s C-terminus and CENP-F. J Mol Biol 2013; 425:4427-41; PMID:23892111; https://doi.org/10.1016/j.jmb.2013.07.027
- Morris-Rosendahl DJ, Kaindl AM. What next-generation sequencing (NGS) technology has enabled us to learn about primary autosomal recessive microcephaly (MCPH). Mol Cell Probes 2015; 29:271-81; PMID:26050940; https://doi.org/10.1016/j.mcp.2015.05.015
- Waters AM, Asfahani R, Carroll P, Bicknell L, Lescai F, Bright A, Chanudet E, Brooks A, Christou-Savina S, Osman G, et al. The kinetochore protein, CENPF, is mutated in human ciliopathy and microcephaly phenotypes. J Med Genetic 2015; 52:147-56; https://doi.org/10.1136/jmedgenet-2014-102691
- O'Brien SL, Fagan A, Fox EJP, Millikan RC, Culhane AC, Brennan DJ, McCann AH, Hegarty S, Moyna S, Duffy MJ, et al. CENP-F expression is associated with poor prognosis and chromosomal instability in patients with primary breast cancer. Int J Cancer 2007; 120:1434-43; PMID:17205517; https://doi.org/10.1002/ijc.22413
- Cao JY, Liu L, Chen SP, Zhang X, Mi YJ, Liu ZG, Li MZ, Zhang H, Qian CN, Shao JY, et al. Prognostic significance and therapeutic implications of centromere protein F expression in human nasopharyngeal carcinoma. Mol Cancer 2010; 9:237; PMID:20828406; https://doi.org/10.1186/1476-4598-9-237
- Habberstad AH, Gulati S, Torp SH. Evaluation of the proliferation markers Ki-67/MIB-1, mitosin, survivin, pHH3, and DNA topoisomerase II alpha in human anaplastic astrocytomas - an immunohistochemical study. Diag Pathol 2011; 6:43; https://doi.org/10.1186/1746-1596-6-43
- Welner S, Trier NH, Frisch M, Locht H, Hansen PR, Houen G. Correlation between centromere protein-F autoantibodies and cancer analyzed by enzyme-linked immunosorbent assay. Mol Cancer 2013; 12:95; PMID:23978088; https://doi.org/10.1186/1476-4598-12-95
- Ueda S, Kondoh N, Tsuda H, Yamamoto S, Asakawa H, Fukatsu K, Kobayashi T, Yamamoto J, Tamura K, Ishida J, et al. Expression of centromere protein F (CENP-F) associated with higher FDG uptake on PET/CT, detected by cDNA microarray, predicts high-risk patients with primary breast cancer. BMC Cancer 2008; 8:384; PMID:19102762; https://doi.org/10.1186/1471-2407-8-384
- Schafer-Hales K, Iaconelli J, Snyder JP, Prussia A, Nettles JH, El-Naggar A, Khuri FR, Giannakakou P, Marcus AI. Farnesyl transferase inhibitors impair chromosomal maintenance in cell lines and human tumors by compromising CENP-E and CENP-F function. Mol Cancer Ther 2007; 6:1317-28; PMID:17431110; https://doi.org/10.1158/1535-7163.MCT-06-0703
- Brown HK, Ottewell PD, Coleman RE, Holen I. The kinetochore protein Cenp-F is a potential novel target for zoledronic acid in breast cancer cells. J Cell Mol Med 2009; 15:501-13; https://doi.org/10.1111/j.1582-4934.2009.00995.x
- Christie M, Chang C-W, Rona G, Smith KM, Stewart AG, Takeda AAS, Fontes MRM, Stewart M, Vertessy BG, Forwood JK, et al. Structural biology and regulation of protein import into the nucleus. J Mol Biol 2016; 428:2060-90; PMID:26523678; https://doi.org/10.1016/j.jmb.2015.10.023
- Conti E, Uy M, Leighton L, Blobel G, Kuriyan J. Crystallographic analysis of the recognition of a nuclear localization signal by the nuclear import factor karyopherin alpha. Cell 1998; 94:193-204; PMID:9695948; https://doi.org/10.1016/S0092-8674(00)81419-1
- Conti E, Kuriyan J. Crystallographic analysis of the specific yet versatile recognition of distinct nuclear localization signals by karyopherin alpha. Structure 2000; 8:329-38; PMID:10745017; https://doi.org/10.1016/S0969-2126(00)00107-6
- Hodel AE, Harreman MT, Pulliam KF, Harben ME, Holmes JS, Hodel MR, Berland KM, Corbett AH. Nuclear localization signal receptor affinity correlates with in vivo localization in Saccharomyces cerevisiae. J Biol Chem 2006; 281:23545-56; PMID:16785238; https://doi.org/10.1074/jbc.M601718200
- Harreman MT, Kline TM, Milford HG, Harben MB, Hodel AE, Corbett AH. Regulation of nuclear import by phosphorylation adjacent to nuclear localization signals. J Biol Chem 2004; 279:20613-21; PMID:14998990; https://doi.org/10.1074/jbc.M401720200
- Stade K, Ford C, Guthrie C, Weis K. Exportin 1 (Crm1p) is an essential nuclear export factor. Cell 1997; 90:1041-50; PMID:9323132; https://doi.org/10.1016/S0092-8674(00)80370-0
- Fornerod M, Ohno M, Yoshida M, Mattaj I. CRM1 is an export receptor for leucine-rich nuclear export signals. Cell 1997; 90:1051-60; PMID:9323133; https://doi.org/10.1016/S0092-8674(00)80371-2
- Ossareh-Nazari B, Bachelerie F, Dargemont C. Evidence for a role of CRM1 in signal-mediated nuclear protein export. Science 1997; 278:141-4; PMID:9311922; https://doi.org/10.1126/science.278.5335.141
- Fukuda M, Asano S, Nakamura T, Adachi M, Yoshida M, Yanagida M, Nishida E. CRM1 is responsible for intracellular transport mediated by the nuclear export signal. Nature 1997; 390:308-11; PMID:9384386; https://doi.org/10.1038/36894
- Dong X, Biswas A, Suel KE, Jackson LK, Martinez R, Gu H, Chook YM. Structural basis for leucine-rich nuclear export signal recognition by CRM1. Nature 2009; 458:1136-41; PMID:19339969; https://doi.org/10.1038/nature07975
- Xu D, Marquis K, Pei J, Fu S-C, Cagatay T, Grishin NV, Chook YM. LocNES: a computational tool for locating classical NESs in CRM1 cargo proteins. Bioinformatics 2015; 31:1357-65; PMID:25515756; https://doi.org/10.1093/bioinformatics/btu826
- Monecke T, Güttler T, Neumann P, Dickmanns A, Görlich D, Ficner R. Crystal structure of the nuclear export receptor CRM1 in complex with Snurportin1 and RanGTP. Science 2009; 324:1087-91; PMID:19389996; https://doi.org/10.1126/science.1173388
- Kirli K, Karaca S, Dehne HJ, Samwer M, Pan KT, Lenz C, Urlaub H, Gorlich D. A deep proteomics perspective on CRM1-mediated nuclear export and nucleocytoplasmic partitioning. Elife 2015; 4:e11466; PMID:26673895; https://doi.org/10.7554/eLife.11466
- Olsen JV, Vermeulen M, Santamaria A, Kumar C, Miller ML, Jensen LJ, Gnad F, Cox J, Jensen TS, Nigg EA, et al. Quantitative phosphoproteomics reveals widespread full phosphorylation site occupancy during mitosis. Science Signal 2010; 3:ra3; https://doi.org/10.1126/scisignal.2000475
- Splinter D, Tanenbaum ME, Lindqvist A, Jaarsma D, Flotho A, Yu KL, Grigoriev I, Engelsma D, Haasdijk ED, Keijzer N, et al. Bicaudal D2, dynein, and kinesin-1 associate with nuclear pore complexes and regulate centrosome and nuclear positioning during mitotic entry. PLoS Biol 2010; 8:e1000350; PMID:20386726; https://doi.org/10.1371/journal.pbio.1000350
- Lee HO, Norden C. Mechanisms controlling arrangements and movements of nuclei in pseudostratified epithelia. Trends Cell Biol 2013; 23:141-50; PMID:23266143; https://doi.org/10.1016/j.tcb.2012.11.001
- Serio G, Margaria V, Jensen S, Oldani A, Bartek J, Bussolino F, Lanzetti L. Small GTPase Rab5 participates in chromosome congression and regulates localization of the centromere-associated protein CENP-F to kinetochores. Proc Natl Acad Sci U S A 2011; 108:17337-42; PMID:21987812; https://doi.org/10.1073/pnas.1103516108
- Gurden MDJ, Holland AJ, van Zon W, Tighe A, Vergnolle MA, Andres DA, Spielmann HP, Malumbres M, Wolthuis RMF, Cleveland DW, et al. Cdc20 is required for the post-anaphase, KEN-dependent degradation of centromere protein F. J Cell Sci 2010; 123:321-30; PMID:20053638; https://doi.org/10.1242/jcs.062075
- Rona G, Marfori M, Borsos M, Scheer I, Takacs E, Toth J, Babos F, Magyar A, Erdei A, Bozoky Z, et al. Phosphorylation adjacent to the nuclear localization signal of human dUTPase abolishes nuclear import: structural and mechanistic insights. Acta Cryst D 2013; 69:2495-505; https://doi.org/10.1107/S0907444913023354
- Rona G, Borsos M, Ellis JJ, Mehdi AM, Christie M, Kornyei Z, Neubrandt M, Toth J, Bozoky Z, Buday L, et al. Dynamics of re-constitution of the human nuclear proteome after cell division is regulated by NLS-adjacent phosphorylation. Cell Cycle 2014; 13:3551-64; PMID:25483092; https://doi.org/10.4161/15384101.2014.960740
- Song C, Ye M, Liu Z, Cheng H, Jiang X, Han G, Songyang Z, Tan Y, Wang H, Ren J, et al. Systematic analysis of protein phosphorylation networks from phosphoproteomic data. Mol Cell Proteomics 2012; 11:1070-83; PMID:22798277; https://doi.org/10.1074/mcp.M111.012625
- Ubersax JA, Woodbury EL, Quang PN, Paraz M, Blethrow JD, Shah K, Shokat KM, Morgan DO. Targets of the cyclin-dependent kinase Cdk1. Nature 2003; 425:859-64; PMID:14574415; https://doi.org/10.1038/nature02062
- Kirby TW, Gassman NR, Smith CE, Pedersen LC, Gabel SA, Sobhany M, Wilson SH, London RE. Nuclear localization of the DNA repair scaffold XRCC1: uncovering the functional role of a bipartite NLS. Sci Rep 2015; 5:13405; PMID:26304019; https://doi.org/10.1038/srep13405
- Fulcher AJ, Roth DM, Fatima S, Alvisi G, Jans DA. The BRCA-1 binding protein BRAP2 is a novel, negative regulator of nuclear import of viral proteins, dependent on phosphorylation flanking the nuclear localization signal. FASEB J 2010; 24:1454-66; PMID:20040518; https://doi.org/10.1096/fj.09-136564
- Ma L, Zhao X, Zhu X. Mitosin/CENP-F in mitosis, transcriptional control, and differentiation. J Biomed Sci 2006; 13:205-13; PMID:16456711; https://doi.org/10.1007/s11373-005-9057-3
- Schneider CA, Rasband WS, Eliceiri KW. NIH Image to ImageJ: 25 years of image analysis. Nat Meth 2012; 9:671-5; https://doi.org/10.1038/nmeth.2089
- Kosugi S, Hasebe M, Tomita M, Yanagawa H. Systematic identification of cell cycle-dependent yeast nucleocytoplasmic shuttling proteins by prediction of composite motifs. Proc Natl Acad Sci U S A 2009; 106:10171-6; PMID:19520826; https://doi.org/10.1073/pnas.0900604106
- Lupas A, Van Dyke M, and Stock J. Predicting coiled coils from protein sequences. Science 1991; 252:1162-4; PMID:2031185; https://doi.org/10.1126/science.252.5009.1162
- Yachdav G, Kloppmann E, Kajan L, Hecht M, Goldberg T, Hamp T, Hönigschmid P, Schafferhans A, Roos M, Bernhofer M, et al. PredictProtein - an open resource for online prediction of protein structural and functional features. Nucleic Acids Res 2014; 42:W337-43; PMID:24799431; https://doi.org/10.1093/nar/gku366
- Emsley P, Lohkamp B, Scott WG, Cowtan K. Features and development of Coot. Acta Cryst D 2010; D66:486-501; https://doi.org/10.1107/S0907444910007493
- Krissinel E, Henrick K. Inference of macromolecular assemblies from crystalline state. J Mol Biol 2007; 372:774-97; PMID:17681537; https://doi.org/10.1016/j.jmb.2007.05.022
- Gallivan JP, Dougherty DA. Cation-pi interactions in structural biology. Proc Natl Acad of Sci U S A 1999; 96:9459-64; https://doi.org/10.1073/pnas.96.17.9459
- Fontes MRM, Teh T, Jans D, Brinkworth RI, Kobe B. Structural basis for the specificity of bipartite nuclear localization sequence binding by importin-alpha. J Biol Chem 2003; 278:27981-7; PMID:12695505; https://doi.org/10.1074/jbc.M303275200
- Fontes MRM, Teh T, Kobe B. Structural basis of recognition of monopartite and bipartite nuclear localization sequences by mammalian importin-alpha. J Mol Biol 2000; 297:1183-94; PMID:10764582; https://doi.org/10.1006/jmbi.2000.3642
- Roman N, Christie M, Swarbrick CMD, Kobe B, Forwood JK. Structural characterisation of the nuclear import receptor importin alpha in complex with the bipartite NLS of Prp20. PLoS One 2013; 8:e82038; PMID:24339986; https://doi.org/10.1371/journal.pone.0082038