ABSTRACT
The increasing resistance of nasopharyngeal carcinoma to irradiation makes the exploration of effective radiosensitizers necessary. Tetrandrine is known to be an antitumor drug, but little is known regarding its radiosensitization effect on nasopharyngeal carcinoma. We investigated the effect of combined treatment of irradiation and maximum non-cytotoxic doses of tetrandrine on the nasopharyngeal carcinoma cell lines CNE1 and CNE2. The maximum non-cytotoxic doses of tetrandrine in CNE1 and CNE2 cells were assessed using the MTT (3-(4,5-dimethylthiazol-2-yl)-2,5-diphenyltetrazolium bromide) assay. The radiosensitization of cells receiving the maximum non-cytotoxic doses of tetrandrine was assessed by evaluating cell proliferation and DNA damage repair using MTT, clonogenic, comet assays and detection of caspase-3 and phosphorylated histone H2AX (γ-H2AX). The cell cycle was assessed by flow cytometry, and protein expression was detected by western blot analysis. The maximum non-cytotoxic doses of tetrandrine in CNE1 and CNE2 cells were 1.5 μmol/L and 1.8 μmol/L, respectively. When cells were exposed to irradiation and the maximum non-cytotoxic doses of tetrandrine, the survival fraction was decreased. DNA damage and γ-H2AX levels markedly increased. Moreover, tetrandrine abrogated the G2/M phase arrest caused by irradiation. Combined treatment with the maximum non-cytotoxic dose of tetrandrine and irradiation caused suppression of the phosphorylation of CDK1 and CDC25C and increase in the expression of cyclin B1. The study in vivo also showed that the maximum non-cytotoxic dose of tetrandrine could reduce tumor growth in xenograft tumor model. Our results suggest that the maximum non-cytotoxic dose of tetrandrine can enhance the radiosensitivity of CNE1 and CNE2 cells and that the underlying mechanism could be associated with abrogation of radiation-induced G2/M arrest via activation of the CDC25C/CDK1/Cyclin B1 pathway.
Introduction
Nasopharyngeal carcinoma is one of the leading cancers in Southern China (especially in the Guangdong province) with an incidence of up to 25 cases per 100000 individuals [Citation1]. Due to anatomic restrictions and high radiosensitivity, radiotherapy has been the mainstay of treatment of nasopharyngeal carcinoma, achieving a 5-year overall survival of more than 80% for early stage I and IIA disease [Citation2]. However, because the early clinical symptoms of nasopharyngeal carcinoma are untypical, at least 60% of patients are present with locally advanced disease, and about 5%–8% present with distant metastasis at preliminary diagnosis [Citation3], and about 20% of nasopharyngeal carcinoma patients develop local recurrence after radiotherapy [Citation4]. Concurrent chemoradiotherapy has been established as the standard of treatment strategy in locoregionally advanced stage III and IVA disease, which achieves an increase in the 5-year overall survival. However, concurrent chemoradiotherapy is limited in its clinical use due to significant increases in dose-limiting toxicity [Citation5]. These challenges make it necessary to explore effective radiosensitizers that enhance the radiosensitivity of nasopharyngeal carcinoma and improve the therapeutic efficacy of treatments used in combination with radiotherapy.
Tetrandrine, a bisbenzylisoquinoline alkaloid, is isolated from the roof of the Chinese herb Stephania tetrandra [Citation6]. It possesses a broad pharmacological profile, including anti-inflammatory, anti-hypertensive and anti-fibrotic properties, which have led to the use of tetrandrine for the treatment of lung silicosis, arthralgia and rheumatoid arthritis in the clinic [Citation7]. Published data have demonstrated that tetrandrine can enhance sensitivity to radiotherapy and inhibit the growth and proliferation of several types of cancer cells, including glioblastoma, neuroblastoma, and esophageal carcinoma [Citation8–10]. Sun et al. [Citation11] found the effect of tetrandrine of radiotherapy sensibilization on nasopharyngeal carcinoma cells, but this study could not elucidate whether the sensitization is caused by the cytotoxicity or by the sensitization effect of tetrandrine. This point should be clarified because increasing doses of cytotoxic drugs could cause severe side effects. Therefore, it is necessary to study the effect and molecular mechanisms of the radiosensitization of the maximum non-cytotoxic dose of tetrandrine.
In the present study, we evaluated whether the maximum non-cytotoxic dose of tetrandrine could augment the response of the human nasopharyngeal carcinoma cell lines CNE1 and CNE2 to irradiation and investigated the molecular mechanism of the radiosensitization effect.
Results
The maximum non-cytotoxic doses of tetrandrine in CNE1 and CNE2
CNE1 and CNE2 cells were separately treated with a range of doses of tetrandrine from 0.1 μmol/L to 2.0 μmol/L to determine the maximum non-cytotoxic dose of tetrandrine. MTT assays were used to generate a cell growth curve. In the CNE1 cell line, cell proliferation was significantly inhibited when the concentration of tetrandrine was more than 1.5 μmol/L ((A)), while in the CNE2 cell line, cells grew significantly more slowly only when the tetrandrine concentration exceeded 1.8 μmol/L ((B)). These results indicated that 1.5 μmol/L and 1.8 μmol/L were the maximum non-cytotoxic doses in the CNE1 and CNE2 cell lines, respectively. Therefore, we used these doses in the following experiments to verify the effect and mechanism of tetrandrine with irradiation.
Tetrandrine enhanced the radiosensitivity of CNE1 and CNE2 cells
To elucidate the effect of the maximum non-cytotoxic dose of tetrandrine on radiation sensitivity in nasopharyngeal carcinoma cells, we generated cell growth curves and cell proliferation indexes using MTT assays. We observed that the survival rate of both CNE1 and CNE2 cells was reduced after irradiation treatment and the proliferation was more strongly suppressed when combined treatment with irradiation and the maximum non-cytotoxic dose of tetrandrine was used ((A, B)). In contrast, there was no cytotoxicity in cells treated with tetrandrine alone.
Figure 2. Effects of the maximum non-cytotoxic doses of tetrandrine on the radiosensitivity of CNE1 and CNE2 cells. (A) and (B) The cell growth curves of CNE1 and CNE2 cells after different tetrandrine exposures. The data shown are the mean and SE from three independent experiments. *p<0.05 vs control, **p<0.05 vs 4 Gy. (C) and (D) The survival fraction of CNE1 cells after different exposures. (E) and (F) The survival fraction of CNE1 cells after different exposures. The data shown are the mean and SE from three independent experiments.
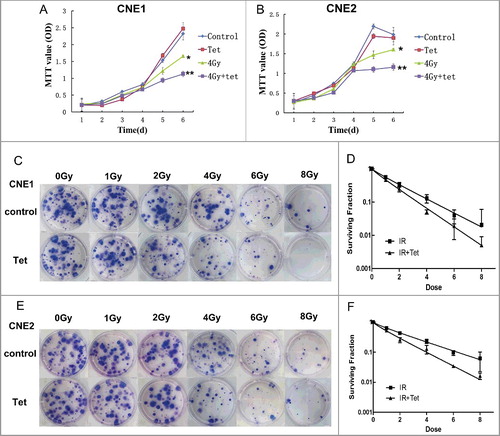
To confirm the sensitization effect of tetrandrine, clone formation was evaluated to examine the radiotherapy sensitivity ((C–F)). The plating efficiency were 0.51 and 0.54 for CNE1 and CNE2 control groups, respectively. The survival fraction in CNE1 was (1 ± 0.089) of control group, (0.97 ± 0.063) of tetrandrine group, (0.129 ± 0.088) of 4Gy irradiation group and (0.050 ± 0.018) of combinate treatment group of 4Gy irradiation and tetrandrine, respectively. And the survival fraction in CNE2 was (1 ± 0.078) of control group, (0.93 ± 0.074) of tetrandrine group, (0.233 ± 0.085) of 4Gy irradiation group and (0.127 ± 0.098) of combinate treatment group of 4Gy irradiation and tetrandrine, respectively. Compared with the control group, there was a decreased survival fraction detected in the irradiation group of CNE1 cells. It is worth noting that following the combined treatment with irradiation and tetrandrine, the survival fraction significantly decreased relative to that of the irradiation group. The same tendency was observed in groups of CNE2 cells. These results demonstrated that tetrandrine could strengthen the inhibition of cell clone formation caused by irradiation, resulting in an enhancement of the sensitivity of CNE1 and CNE2 cells to irradiation.
Combined treatment with tetrandrine and irradiation increased apoptosis of CNE1 and CNE2 cells
Apoptosis is one of the most important end points for irradiation. We investigated whether the radiosensitization by tetrandrine in CNE1 and CNE2 cells related to apoptosis. The expression of cleaved-Caspase3, a specific apoptosis marker, was detected by western blotting. Cells were treated with the maximum non-cytotoxic dose of tetrandrine 1 h after irradiation, and protein was collected 6 h later. (A, C and E) shows increasing cleaved-Caspase3 protein expression following treatment with irradiation alone in CNE1 cells, while the expression was significantly increased after treatment with the combination of the maximum non-cytotoxic dose of tetrandrine and irradiation, which indicated that tetrandrine can upregulate the apoptosis induced by irradiation. The same tendency was observed in CNE2 cells ((B, D, F)).
Figure 3. Caspase3 activity in CNE1 and CNE2 cells was upregulated by tetrandrine during irradiation exposure. (A) The western blot image for cleaved-caspase3 and caspase3 protein expression in CNE1 cells. (B) The western blot image for cleaved-caspase3 and caspase3 protein expression in CNE2 cells. (C) and (E) Quantification of the western blot band intensity for cleaved-caspase3 and caspase3 in CNE1 cells. (D) and (F) Quantification of the western blot band intensity for cleaved-caspase3 and caspase3 in CNE2 cells. Each data point represents the mean and SE from 3 experiments (*p<0.05 vs control, #p<0.05 vs 4Gy).
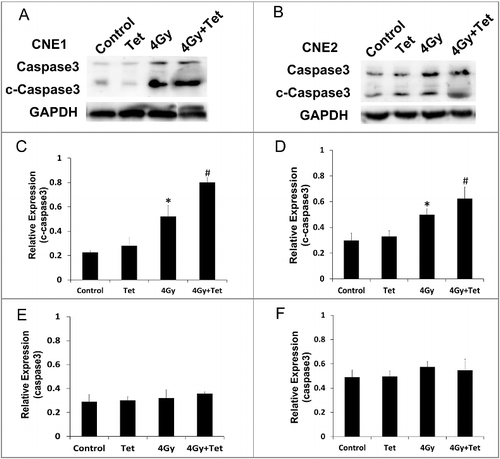
Combined treatment with tetrandrine and irradiation increased DNA double-strand breaks
The main type of damage induced by ionizing rays is DNA double-strand breaks (DSBs), and one of the most important mechanisms for cancer cell resistance to radiotherapy is the high capacity for DNA DSB repair. To examine DNA damage, comet assay was used. As shown in , 4 Gy of irradiation alone induced severer DNA damage than that occurred in control group of CNE1 cells. Exposure to 1.5 μmol/L tetrandrine treatment alone did not induce more DNA damage than the control group, which implied that the maximum non-cytotoxic dose we used in the experiments could not induce DNA damage in cells. Combined treatment with tetrandrine and irradiation significantly increased DNA damage compared to both control group and irradiation group. The same tendency was observed in CNE2 cells. It can be inferred that radiotherapy can cause a significant increase in DNA damage, which was further enhanced by a combination of irradiation and the maximum non-cytotoxic dose of tetrandrine.
Figure 4. Effects of the maximum non-cytotoxic doses of tetrandrine on DNA integrity. (A) Double-strand breaks were analyzed by DNA comet assay. Both CNE1 and CNE2 cells exposed to combined treatment of tetrandrine and irradiation exhibited more significant migration of DNA into the tail of the comet compared with cells exposed to irradiation alone. (B) CNE1 cells were exposed to tetrandrine, irradiation (4 Gy), or combined treatment for 24 h. Western blotting was performed to determine the level of γ-H2AX. (C) Quantification of the western blot band intensity for γ-H2AX in CNE1 cells was performed using ImageJ. Each data point represents the mean and SE from 3 experiments (*p<0.05 vs control, #p<0.05 vs 4 Gy). (D) CNE2 cells were exposed to tetrandrine, irradiation (4 Gy), or combined treatment for 24 h. Western blotting was performed to determine the level of γ-H2AX. (E) Quantification of the western blot band intensity for γ-H2AX in CNE2 cells was performed using ImageJ. Each data point represents the mean and SE from 3 experiments (*p<0.05 vs control, #p<0.05 vs 4 Gy).
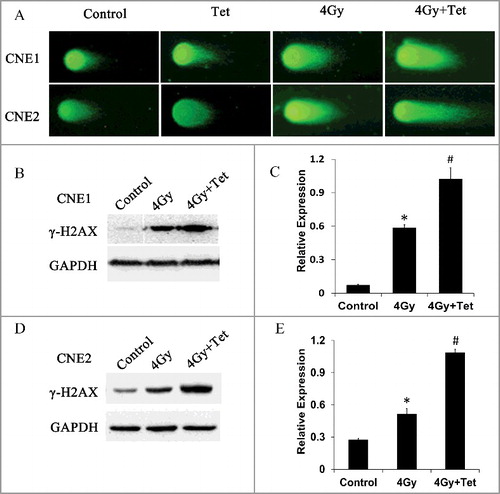
As further proof, we used γ-H2AX to verify DNA damage caused by irradiation and tetrandrine. γ-H2AX on Ser139 is one of the key events of the DNA damage response and is considered to be a marker of DSBs. The expression of γ-H2AX was measured by western blotting (). The expression of γ-H2AX increased after irradiation compared with the control. In addition, the combined treatment of tetrandrine and irradiation led the expression of γ-H2AX increase even more significantly.
These results confirmed that tetrandrine could significantly enhance DNA damage caused by irradiation.
Tetrandrine abrogated the G2/M phase arrest caused by irradiation in CNE1 and CNE2 cells
It is well known that after the induction of DNA damage, including DSBs, cell cycle progression can be interrupted to provide time for damage repair. Flow cytometry was used to observe whether cell cycle transition was influenced by treatment with tetrandrine. As shown in , combined treatment with 4 Gy irradiation and tetrandrine restrained G2/M phase arrest caused by irradiation. In the 4 Gy irradiation group, the percentages of CNE1 and CNE2 cells at G2/M phase were increased to (18.09 ± 1.97)% and (19.58 ± 1.74)%, respectively, compared with (7.81 ± 0.97)% and (8.69 ± 0.82)%, respectively, for the control group. Nevertheless, combined treatment with the maximum non-cytotoxic dose of tetrandrine and irradiation decreased the percentages of CNE1 and CNE2 cells at G2/M phase to (10.02 ± 1.03)% and (9.78 ± 1.22)%, respectively. Therefore, removing the arrest of G2/M phase might be associated with the radiotherapy sensitization effect of tetrandrine.
Figure 5. Effects of tetrandrine with irradiation on cell cycle distribution. (A) CNE1 cells were cultured for 24 h with 1.5 μmol/L tetrandrine, 4 Gy irradiation, or a combination of 1.5 μmol/L tetrandrine and 4 Gy irradiation. The cell cycle distribution of the cells was investigated. (B) CNE cells were cultured for 24 h with 1.8 μmol/L tetrandrine, 4 Gy irradiation, or a combination of 1.8 μmol/L tetrandrine and 4 Gy irradiation. The cell cycle distribution of the cells was investigated. (C) The percentages of cells in the cell cycle phases were determined. The mean and SE were presented for three independent experiments. (*p<0.05 vs control, #p<0.05 vs 4 Gy.)
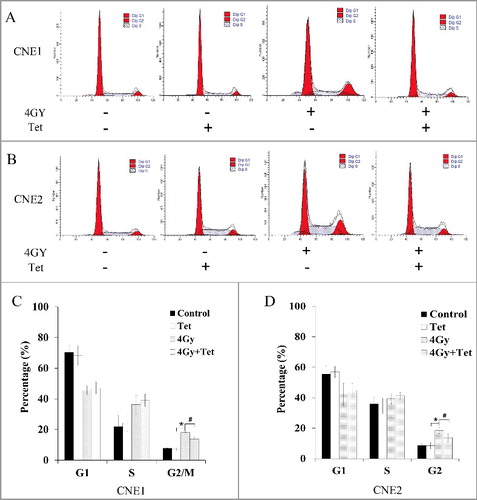
Tetrandrine prevented G2/M arrest by regulating the CDC25C/CDK1/cyclin B1 pathway
To understand the molecular mechanisms by which tetrandrine removes G2/M phase arrest, we studied intracellular signaling by western blotting. shows that combined treatment with the maximum non-cytotoxic doses of tetrandrine and irradiation decreased the phosphorylation of CDK1(Tyr15), the inactive state, but the expression of total CDK1 did not change, which suggests that combined treatment could raise the ratio of activated CDK1. The combined treatment with tetrandrine and irradiation also increased the expression of cyclin B1. It is well known that the activation of the CDK1-cyclin B1 complex requires the activation of CDK1 and expression of cyclin B1, thus our results suggested that the activation of the CDK1-cyclin B1 complex may be a target for the radiosensitivity effect of tetrandrine.
Figure 6. Tetrandrine treatment with irradiation regulated cell cycle-related proteins. (A) The expression of key protein in CNE1 cells. (B) The expression of key protein in CNE2 cells. Cells receiving 4 Gy irradiation pretreatment were exposed to 1.5 μmol/L (CNE1) or 1.8 μmol/L (CNE2) tetrandrine for 1 h, 6 h, and 24 h before collection for western blot analysis.
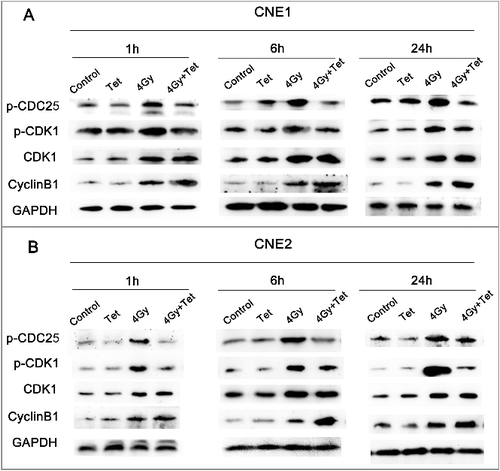
The CDK1-cyclin B1 complex can be activated by CDC25C protein via dephosphorylation of CDK1. CDC25C is inactivated by phosphorylation at Ser216. We found that combined treatment with tetrandrine and irradiation causes a decrease in p-CDC25C-S216 protein expression compared with treatment with irradiation alone. Therefore, tetrandrine may prevent the phosphorylation of CDC25C and increase the activation of CDK1 and cyclin B1, thereby decreasing G2/M arrest.
Tetrandrine enhanced the radiosensitivity of nasopharyngeal carcinoma xenograft tumor growth in vivo.
To evaluate the effect of the maximum non-cytotoxic doses of tetrandrine on radiosensitivity in vivo, we used a subcutaneous xenograft model of CNE1 cells in immunodeficient mice. A range of doses of tetrandrine from 0 mg/kg to 100 mg/kg to determine the maximum non-cytotoxic dose of tetrandrine. As shown in (A and B), tetrandrine of both 75 mg/kg and 100 mg/kg significantly reduced the tumor volume, while tetrandrine of 25 mg/kg and 50 mg/kg had no significant effect on tumor growth. What was more, tetrandrine of both 75 mg/kg and 100 mg/kg significantly reduced the body weight of mice, which indicated more side effect. Therefore, we used tetrandrine of 50 mg/kg in the following experiments in vivo. We found that tetrandrine of 50 mg/kg enhanced the cytotoxicity of irradiation ((B)), without significant weight loss ((C)).
Figure 7. Tetrandrine inhibited nasopharyngeal carcinoma xenograft growth in vivo. (A) The tumor growth curves of mice model after different tetrandrine exposures. (B) The body weight of mice model after different tetrandrine exposures. (C) The tumor growth curves of mice model after treatment with tetrandrine or irradiation. (D) The body weight of mic model after treatment with tetrandrine or irradiation.
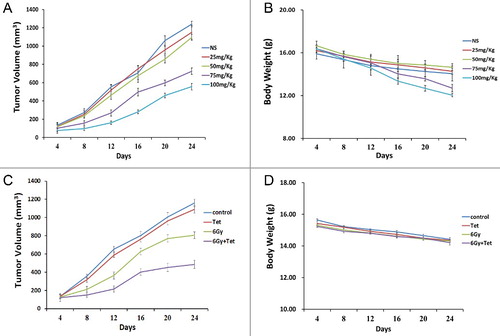
Discussion
Radiotherapy is one of the most common and effective methods for nasopharyngeal carcinoma treatment, for both the radiosensitivity of nasopharyngeal carcinoma and the inaccessible location of the nasopharynx. Advances in radiation have improved the prognosis of nasopharyngeal carcinoma, however, many patients still suffer the treatment failures due to the radioresistance of tumors and residual tumor cells after radiation [Citation12]. Chemoradiotherapy has been used to control advanced nasopharyngeal carcinoma, however, effective agents for combination chemoradiotherapy for nasopharyngeal carcinoma are still to be established [Citation13]. What is more, compared with radiotherapy alone, chemoradiotherapy is invariably associated with higher incidences of complications, such as haematological and non-haematological acute toxic effects [Citation14]. Therefore, studies on radiosensitizer are of great importance which may provide a more effective therapeutic strategy.
Recently, increasing attention has been paid to the radiosensitivity effect of tetrandrine [Citation9,Citation10]. In human neuroblastoma cells, significant concentration-dependent and time-dependent synergistic cytotoxic effects of tetrandrine and radiation have been demonstrated [Citation9]. The radiotherapy-Tet protocol (using irradiation at the beginning of tetrandrine treatment) was more cytotoxic for neuroblastoma cells than the Tet-radiotherapy protocol (using irradiation after tetrandrine treatment) [Citation9]. Yu et al. found that tetrandrine could enhance the radiosensitivity of esophageal carcinoma cells by a mechanism that might involve relief of radiation-induced G2/M arrest [Citation10].
Sun et al. [Citation11] found the effect of tetrandrine of radiotherapy sensibilization on nasopharyngeal carcinoma cells, however, the concentration of tetrandrine used in this study was cytotoxic to cancer cells, which indicated that the side effects of the combined treatment also increased. Consequently, it was unclear whether the sensitization is caused by cytotoxicity or by the sensitization effect of tetrandrine. Our results showed that the maximum non-cytotoxic doses of tetrandrine for CNE1 and CNE2 cells were 1.5 μmol/L and 1.8 μmol/L, respectively. These concentrations were used in subsequent experiments to eliminate the cytotoxicity of tetrandrine and retain only its sensitization effect. We found that the maximum non-cytotoxic dose of tetrandrine treatment combined with irradiation inhibited the proliferation of CNE1 and CNE2 cells and caused a significant decrease in the number of clones relative to irradiation treatment alone. The results inferred that tetrandrine could enhance the radiosensitivity of CNE1 and CNE2. The study in vivo also revealed that the maximum non-cytotoxic dose of tetrandrine could amplify the suppression of tumor growth caused by irradiation. Furthermore, the maximum non-cytotoxic dose of tetrandrine had no obvious side effect, as convinced by showing no significant weight loss of mice.
The most deleterious lesion induced by irradiation is thought to be DSBs. At the occurrence of a DNA lesion, the cell will initiate repair to protect the integrity of the genetic material. If DNA cannot be fully repaired, cells will undergo apoptosis [Citation15]. The high DNA-damage repair capacity of cancer cells leads to irradiation resistance. Our data provided direct evidence that combined treatment with the maximum non-cytotoxic dose of tetrandrine and irradiation could increase DNA damage and reduce DNA repair, leading to an increase in the activation of apoptotic factors.
After DNA damage, cell cycle checkpoints at both the G1/S transition and G2/M transition become active to provide time for cells to repair DNA damage before mitosis to protect genomic stability [Citation16]. If DNA repair fails and cells are released into mitosis in the presence of DSB repair, chromosomal breaks in mitosis will increase, which can cause the activation of apoptosis [Citation17]. Cancer cells may harbor DNA damage by skipping the G1/S checkpoint. Then, the G2/M checkpoint is the last phase where damage can be repaired, thus making it a key defender of cancer cell progression [Citation18]. Therefore, G2/M checkpoint abrogation has been investigated as a means of enhancing the therapeutic index of cytotoxic agents. Recently, several studies have shown that removal of arrest G2/M phase arrest has significant effects on the radiosensitivity of cancer cells [Citation19–21]. Tetrandrine is also recognized as an enhancer of radiosensitivity via abrogation of G2/M arrest in various kinds of cancer [Citation9,Citation22]. It can sensitize human breast cancer cells to irradiation by abolishing G2/M checkpoint controls and inducing the entry of the irradiated cells into mitosis [Citation22]. Chen et al. noted that abrogation of radiation-induced G2/M arrest was related to tetrandrine-induced radiosensitization in neuroblastoma cells [Citation9]. In agreement with these studies, we observed that irradiation arrested CNE1 and CNE2 cells in G2/M phase. Following combined treatment with the maximum non-cytotoxic dose of tetrandrine and irradiation, G2/M arrest was significantly reduced. This finding indicates that the regulation of cell cycle progression caused by tetrandrine was probably related to the sensitization to irradiation. By abrogating the G2/M delay caused by irradiation, tetrandrine treatment may shorten the time for repair of irradiation-induced DNA damage, resulting in a stronger cytotoxic effect and radiosensitivity.
The G2/M phase transition is regulated by the CDK1-cyclin B1 complex directly. Dephosphorylation of CDK1 and expression of cyclin B1 are required for the activation of the CDK1-cyclin B1 complex [Citation23]. During G2/M arrest, the CDK1-cyclin B1 complex is kept in an inactive state by phosphorylation at the conserved tyrosine 15 residue of CDK1 [Citation24]. This inactivation can be abolished by the dephosphorylation of CDK1 by CDC25C, a protein phosphatase whose activity is downregulated via phosphorylation on Ser216 [Citation25]. Thus, the reduced activity of CDC25C and a subsequent increase of CDK1 phosphorylation is the hallmark of cell cycle arrest at G2/M phase. A dose-dependent effect of tetrandrine on the activation of G2/M checkpoint kinases was observed in our study. With an increase in the concentration of tetrandrine, p-CDC25C-Ser216 and p-CDK1-Tyr15 was increased while the expression of total CDK1 did not change. These results showed that tetrandrine itself could halt cell cycle progression at G2/M phase, which appeared to occur in a concentration-dependent manner. This conclusion was also consistent with recent studies [Citation26,Citation27]. We also observed that using the maximum non-cytotoxic dose, 1.5 μmol/L and 1.8 μmol/L in CNE1 and CNE2 cells, respectively, had no significant effect on the activation of G2/M checkpoint kinases.
We contrasted the difference between irradiation treatment and combined treatment with tetrandrine and irradiation. After irradiation, the phosphorylation of CDC25C and CDK1 sharply increased while the expression of cyclin B1 did not change. In contrast, the increased phosphorylation of CDC25C and CDK1 was reversed while the total CDK1 did not change after combined treatment with tetrandrine and irradiation, which indicated that a higher proportion of CDK1 was activated. Combined treatment also promoted the expression of cyclin B1. Therefore, it could be inferred that following combined treatment, the activity of the CDK1-cyclin B1 complex increased, resulting in the G2/M checkpoint transition. This conclusion was consistent with the phenomenon observed in cell cycle analysis.
In conclusion, our study suggests that the maximum non-cytotoxic dose of tetrandrine might efficiently enhance the sensitivity of irradiation in CNE1 and CNE2 cells and improve the cytotoxicity of irradiation. The sensitization mechanism might be involved in downregulation of the phosphorylation of CDK1 and CDC25C, promoting the activation of these proteins and increasing the expression of cyclin B1. Such regulation could prevent DNA repair at the G2/M checkpoint and increase the DNA damage and apoptosis caused by irradiation.
Materials and methods
Cell culture
The human nasopharyngeal carcinoma cell lines CNE1 and CNE2 were obtained from Sun Yat-sen University Cancer Center (Guangzhou, China). Cells were cultured in RPMI-1640 medium (Gibco) supplemented with 10% fetal bovine serum (FBS, Gibco). Cultures were maintained in a standard incubator at 37°C and 5% CO2. The culture medium was changed every 24 h.
Radiation
Cells were exposed to irradiation with a total dose of 4Gy at 250 cGy/min using the Siemens Primus accelerator with 6 MV-photos. All irradiation experiments were carried out at room temperature.
The maximum non-cytotoxic doses of tetrandrine
Tetrandrine was purchased from Zhejiang Haizheng Pharmaceutical Company Limited (Taizhou, Zhejiang, China), with a purity of over 98%. The solution of tetrandrine was diluted in 1mol/L hydrochloric acid to achieve a final concentration of 2500 µM, which was diluted from 0.1 μmol/L to 2.0 μmol/L in RPMI-1640 medium supplemented with 10% FBS for use. MTT assays were performed for 6 consecutive days to generate data for a cell growth curve. The maximum dose of tetrandrine that had no effect on cell proliferation was chosen to be the maximum non-cytotoxic dose.
MTT assay
CNE1 and CNE2 cells were seeded into 96-well plates at a density of 1 × 103 cells/well. Cells were treated with concentrations (0–2.0 μmol/L) of tetrandrine or irradiation and incubated for 1 to 6 days. Tetrandrine was added to combined treated cells 1h after irradiation. After incubation, the medium was removed from each well, and 20 μL of MTT (0.5 mg/mL) (Sigma-Aldirich, USA) was added to each well, and the plates were incubated for another 4 h. Then, 150 μL of dimethylsulfoxide (DMSO, Sigma-Aldirich, USA) was added to dissolve formazan products. The solution was kept in the dark for 10 min at room temperature. The absorbance of the solution was read at 490 nm.
Clonogenic assays
Exponentially growing cells were irradiated at doses of 1–8 Gy at room temperature and then incubated in the presence or absence of tetrandrine1h after irradiation. After this treatment, cells were washed in PBS and trypsinized. Cells were seeded in a 24-well plate in 5 ml medium at a density of 200 cells/well. Colonies were grown for 10−14 d. Plates were washed in PBS, and colonies were fixed with 95% ethanol. Staining was performed using 0.1% crystal violet solution. Colonies of more than 50 cells were counted to calculate the surviving fraction. The proliferation index was the radio of OD value of combined treated group to control group. Six parallel samples were scored for each treatment condition.
Western blot analysis
Cell extracts were obtained with lysis buffer (Beyotime, China). Protein concentration was quantified by BCA protein assay kit (Thermo Scientific, MA). Western blots were performed as previously described [Citation28]. Rabbit monoclonal anti-cyclin B1 (ab32053), phospho-CDC25C-Ser216 (E190), rabbit polyclonal phospho-CDK1-Tyr15 (ab47594), and mouse monoclonal CDK1 (ab18) antibodies were purchased from Abcam. Rabbit polyclonal anti-cleaved-Caspase3 (9661) and anti-Caspase3 (9662) was purchased from Cell Signaling Technology. Polyclonal rabbit anti-GAPDH (10494-1-AP) were pursed from ProteinTech. Polyclonal goat anti-rabbit and anti-mouse secondary antibodies were purchased from CWBIO.
Neutral comet assay
Cells were seeded and irradiated at a dose of 4 Gy in 25-ml culture flasks and incubated in the presence of tetrandrine for 24 h. Cells were irradiated as a monolayer cultured in culture flask. Tetrandrine was added to combined treated cells 1h after irradiation. Cells (1 × 105) were combined with molten LMAgarose at a ratio of 1:10 (v/v) and pipetted onto Comet Slides immediately. After being placed flat at 4°C in the dark for 10 min, slides were immersed in lysis solution at 4°C for 1 h and then washed in neutral electrophoresis buffer for 30 min at 4°C. Then, slides were immersed in DNA precipitation solution and 70% ethanol in turn for 30 min at room temperature. SYBR Green I (100 μL) was placed onto each sample for 30 min in the dark. Slides were viewed by epifluorescence microscopy.
Flow cytometric analysis of the cell cycle
Cells were seeded and irradiated at a dose of 4 Gy in 25-ml culture flasks and incubated in the presence of tetrandrine for 24 h. Tetrandrine was added to combined treated cells 1h after irradiation. Cells (1 × 106) from each group were harvested 24h after irradiation and washed with PBS. Then, 50 μL of 100 μg/mL RNase (Sigma-Aldirich, USA) was added. The cells were incubated for 30 min with RNase solution and then placed on ice. Immediately prior to measurement, the cells were stained by adding 100 μl of 50 μg/mL propidium iodide (PI, Sigma-Aldirich, USA). The histogram of DNA content measured with FACSCalibur (BD Biosciences, USA) was analyzed using FlowJo software.
In vivo
Five-week-old immunodeficient BALB/c nu/nu female mice (18–22 g) were purchased from the Experimental Animal Center of Sun Yat-sen University (Guangzhou, China) and housed in barrier facilities on a 12 h light/dark cycle. CNE1 cells were injured subcutaneously into the right flank (3 × 106 cells in 200 μL of RPMI-1640 medium). When the xenograft volumes reached approximately 100 mm3, the transplanted mice were randomly divided into 4 groups (n = 5 mice each): control group, 50mg/kg tetrandrine group, 6Gy group and combined treatment group. An 6Gy dose of irradiation was delivered to the tumor of 6Gy group and combined treatment group. One day after irradiation, mice were treated by oral administration of 50mg/kg tetrandrine (50mg/kg tetrandrine group and combined treatment group) or normal saline (control group and 6Gy group) every other day. The tumor volumes were determined by measuring length (l) and width (w) and calculating volume (V = 0.5 × l × w2) every 4 days. 24 days after irradiation, the mice were killed by cervical dislocation.
Statistical analysis
Data are expressed as the mean ± SD. Statistical software SPSS 16.0 was used to perform Student's t-tests. The significant difference criterion was set at p<0.05.
Disclosure of potential conflicts of interest
No potential conflicts of interest were disclosed.
Acknowledgments
We thank the radiology department of the Cancer Center of the First Affiliated Hospital of Jinan University (Guangzhou Overseas Chinese Hospital) for their assistance with radiotherapy.
Additional information
Funding
References
- Chan AT. Nasopharyngeal carcinoma. Ann Oncol: Off J Euro Soc Med Oncol. 2010;21(Suppl 7):vii308–312. doi:10.1093/annonc/mdq277 PMID:20943634
- Xiao WW, Han F, Lu TX, et al. Treatment outcomes after radiotherapy alone for patients with early-stage nasopharyngeal carcinoma. Int J Radiat Oncol Biol Phys. 2009;74:1070–1076. doi:10.1016/j.ijrobp.2008.09.008. PMID:19231110
- Liang Z, Zhu X, Li L, et al. Concurrent chemoradiotherapy followed by adjuvant chemotherapy compared with concurrent chemoradiotherapy alone for the treatment of locally advanced nasopharyngeal carcinoma: a retrospective controlled study. Curr Oncol. 2014;21:e408–e417. doi:10.3747/co.21.1777. PMID:24940100
- Suarez C, Rodrigo JP, Rinaldo A, et al. Current treatment options for recurrent nasopharyngeal cancer. Eur Arch Otorhinolaryngol. 2010;267:1811–1824. doi:10.1007/s00405-010-1385-x. PMID:20865269
- Yu H, Wang X, Song A, et al. Concurrent chemoradiotherapy versus radiotherapy alone for locoregionally advanced nasopharyngeal carcinoma. Asian Pac J Cancer Prev. 2012;13:3961–3965. doi:10.7314/APJCP.2012.13.8.3961. PMID:23098500
- Chen YJ. Potential role of tetrandrine in cancer therapy. Acta Pharmacol Sin. 2002;23:1102–1106. PMID:12466047
- Xie QM, Tang HF, Chen JQ, et al. Pharmacological actions of tetrandrine in inflammatory pulmonary diseases. Acta Pharmacol Sin. 2002;23:1107–1113. PMID:12466048
- Chang KH, Chen ML, Chen HC, et al. Enhancement of radiosensitivity in human glioblastoma U138MG cells by tetrandrine. Neoplasma. 1999;46:196–200. PMID:10613597
- Chen Y, Chen JC, Tseng SH. Effects of tetrandrine plus radiation on neuroblastoma cells. Anticancer Res. 2009;29:3163–3171. PMID:19661330
- Yu J, Liu F, Sun M, et al. Enhancement of radiosensitivity and the potential mechanism on human esophageal carcinoma cells by tetrandrine. Cancer Biother Radiopharm. 2011;26:437–442. doi:10.1089/cbr.2011.0964. PMID:21797675
- Sun X, Xu R, Deng Y, et al. Effects of tetrandrine on apoptosis and radiosensitivity of nasopharyngeal carcinoma cell line CNE. Acta Biochimica et Biophysica Sinica. 2007;39:869–878. doi:10.1111/j.1745-7270.2007.00349.x. PMID:17989878
- Rottey S, Madani I, Deron P, et al. Modern treatment for nasopharyngeal carcinoma: current status and prospects. Curr Opin Oncol. 2011;23:254–258. doi:10.1097/CCO.0b013e328344f527. PMID:21330921
- Chow JP, Man WY, Mao M, et al. PARP1 is overexpressed in nasopharyngeal carcinoma and its inhibition enhances radiotherapy. Mol Cancer Ther. 2013;12:2517–2528. doi:10.1158/1535-7163.MCT-13-0010. PMID:23979918
- Chua MLK, Wee JTS, Hui EP, et al. Nasopharyngeal carcinoma. Lancet. 2016;387:1012–1024. doi:10.1016/S0140-6736(15)00055-0. PMID:26321262
- Cavallo F, Feldman DR, Barchi M. Revisiting DNA damage repair, p53-mediated apoptosis and cisplatin sensitivity in germ cell tumors. Int J Dev Biol. 2013;57:273–280. doi:10.1387/ijdb.130135mb. PMID:23784838
- Beishline K, Azizkhan-Clifford J. Interplay between the cell cycle and double-strand break response in mammalian cells. Methods Mol Biol. 2014;1170:41–59. doi:10.1007/978-1-4939-0888-2_3. PMID:24906308
- Deckbar D, Jeggo PA, Lobrich M. Understanding the limitations of radiation-induced cell cycle checkpoints. Crit Rev Biochem Mol Biol. 2011;46:271–283. doi:10.3109/10409238.2011.575764. PMID:21524151
- Bucher N, Britten CD. G2 checkpoint abrogation and checkpoint kinase-1 targeting in the treatment of cancer. Br J Cancer. 2008;98:523–528. doi:10.1038/sj.bjc.6604208. PMID:18231106
- Gogineni VR, Nalla AK, Gupta R, et al. Chk2-mediated G2/M cell cycle arrest maintains radiation resistance in malignant meningioma cells. Cancer Lett. 2011;313:64–75. doi:10.1016/j.canlet.2011.08.022. PMID:21945852
- Yong KJ, Milenic DE, Baidoo KE, et al. Sensitization of tumor to (2)(1)(2)Pb radioimmunotherapy by gemcitabine involves initial abrogation of G2 arrest and blocked DNA damage repair by interference with Rad51. Int J Radiat Oncol Biol Phys. 2013;85:1119–1126. doi:10.1016/j.ijrobp.2012.09.015. PMID:23200172
- Anastasov N, Hofig I, Vasconcellos IG, et al. Radiation resistance due to high expression of miR-21 and G2M checkpoint arrest in breast cancer cells. Radiat Oncol. 2012;7:206. doi:10.1186/1748-717X-7-206. PMID:23216894
- Sun XC, Cheng HY, Deng YX, et al. Tetrandrine: a potent abrogator of G2 checkpoint function in tumor cells and its mechanism. Biomed Environ Sci. 2007;20:495–501. PMID:18348409
- Smits VA, Klompmaker R, Vallenius T, et al. p21 inhibits Thr161 phosphorylation of Cdc2 to enforce the G2 DNA damage checkpoint. J Biol Chem. 2000;275:30638–30643. doi:10.1074/jbc.M005437200. PMID:10913154
- Fisher D, Krasinska L, Coudreuse D, et al. Phosphorylation network dynamics in the control of cell cycle transitions. J Cell Sci. 2012;125:4703–4711. doi:10.1242/jcs.106351. PMID:23223895
- Nigg EA. Mitotic kinases as regulators of cell division and its checkpoints. Nat Rev Mol Cell Biol. 2001;2:21–32. doi:10.1038/35048096. PMID:11413462
- Hou Y, Guo T, Wu C, et al. Effect of tetrandrine combined with epirubicin on the growth of human breast carcinoma multidrug resistance cell line. Yakugaku Zasshi. 2008;128:663–666. doi:10.1248/yakushi.128.663. PMID:18379185
- Yu VW, Ho WS. Tetrandrine inhibits hepatocellular carcinoma cell growth through the caspase pathway and G2/M phase. Oncol Rep. 2013;29:2205–2210. doi:10.3892/or.2013.2352. PMID:23525490
- Qin L, Fan M, Candas D, et al. CDK1 enhances mitochondrial bioenergetics for radiation-induced DNA repair. Cell Rep. 2015;13:2056–2063. doi:10.1016/j.celrep.2015.11.015. PMID:26670043