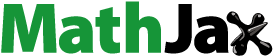
ABSTRACT
Gefitinib (GEB) is one of the drugs used for patients with epidermal growth factor receptor (EGFR)-positive mutations in non-small cell lung cancer (NSCLC). However, application of GEB is limited by its low water solubility, stability, and utilization rate, especially the side effects while GEB is given by oral. In this study, nanoliposome was used as a carrier to prepare nanoliposome compound drug (GL) by embedding GEB in the nanoliposome perfectly combined with green nontoxic solvent and thin-film dispersion method. The nanoliposome structure was expected to improve the water solubility and biocompatibility of GEB, thus improving the effect of cancer treatment. The surface electronegative nanoliposomes can effectively avoid protein adsorption and prolong the circulation time in vivo. Meanwhile, the ratio of lecithin to cholesterol (LE/CH) was explored to maximize the encapsulation efficiency of nanoliposome. Subsequent test results showed that GL exhibited better stability, smaller particle size and higher encapsulation efficiency. In addition, in vitro drug release curve also further confirmed that GL had a promising drug sustained-release effect. In particular, a series of in vitro tests such as cell activity, apoptosis, colony formation, scratch, invasion, and cell cycle assays were performed. The results indicated that GL significantly enhanced the pro-apoptotic effect on A549 cells. Most cell cycles of A549 cells were blocked in the G0/G1 phase influenced by GL, thus inhibiting the proliferation of cancer cells. In vivo anti-tumor studies showed that compared with pure GEB, GL had a significant inhibiting effect on NSCLC. In conclusion, the GL which was synthesized by a simple method in this study significantly improved the treatment effect of cancer cells, which proved that the nanoliposome carrier had an excellent application prospect in the treatment of lung cancer.
KEYWORDS:
1. Introduction
At present, lung cancer is a malignant tumor with the highest morbidity (11.6%) and mortality (18.4%) [Citation1], about 75–80% of which is non-small cell lung cancer (NSCLC) [Citation2]. Smoking, ionizing radiation, and air pollution are the main carcinogenic factors of lung cancer [Citation3–5]. The conventional treatments such as surgical resection, radiotherapy, and chemotherapy have a poor curative effect on lung cancer, and most of the chemotherapy is given mainly by intravenous administration, with dispersed drug distribution and obvious toxic side effects [Citation6]. In addition, the utilization of oral anticancer drugs is affected by primary metabolism. Based on dose-response effects, chemotherapeutic drugs have been found to cause significant toxic side effects to normal cells, resulting in increased weakness and death in patients [Citation7]. Therefore, in order to reduce the toxicity of chemotherapy drugs in vivo, drug sustained release and targeted drug delivery have become the focus of current research [Citation8–11]. Meanwhile, relevant studies have found that many inhibitors with anti-cancer signaling pathways can significantly improve the therapeutic effect of NSCLC [Citation12–15].
In recent years, epidermal growth factor receptor tyrosine kinase inhibitor (EGFR-TKI) has broken through the bottleneck of traditional chemotherapy drugs due to its specific efficacy, mild adverse reactions, and convenient oral administration [Citation16,Citation17], and it has become an indispensable treatment method of advanced NSCLC [Citation18,Citation19]. Clinical results show that first-line EGFR-TKIS therapy for NSCLC with EGFR mutations is superior to standard chemotherapy [Citation20], in which gefitinib (GEB) shows better curative effect. GEB as a selective EGFR-TKI, can reduce the activity of tyrosine kinase and block the information transmission between tumor cells involving EGFR, thus inhibiting the survival, proliferation, and metastasis of tumor cells and playing an anti-tumor role [Citation21]. Currently, the marketed GEB preparations are tablets with problems of slow oral absorption, wide distribution in vivo and increasing degree of gastrointestinal adverse reactions with the dosage increase. It was reported that the details of microtubules’ dynamic and static behavior are critical to drug efficacy [Citation22,Citation23]. Microtubules are the small fibers of tubulin that constitute the living cytoskeleton and play an important role in mitosis. The mechanical loads and natural dynamics generated by biological cells play a prominent role in estimating the mechanics, especially the linear and nonlinear frequency responses of microtubules [Citation24]. In the dynamic stability reaction of the microtubules, the physical proximity of the cells (such as membrane and cell matrix) will be prominent [Citation25]. Since microtubules are likely to be used as biosensors, this property could be used to indicate malignant tumors [Citation26] with clinical guiding significance for the diagnosis and drug therapy of tumor. Therefore, it is necessary to load or coat GEB with some specific nano-carriers to reduce its in vivo toxicity and improve the therapeutic effect.
In recent years, drug carriers with certain targeting or aggregation functions, especially nanoliposomes, have been widely used to solve poor infiltration of drugs in tumors [Citation27]. Nanoliposomes are bilayer-structured circular vesicles formed by phospholipids and cholesterol (CH), which can coat hydrophilic and lipophilic drugs, and can also load biological macromolecules [Citation28,Citation29]. Because of the enhanced permeability and retention (EPR) effect of nanoscale, nanoliposomes can be passively targeted and enriched in tumor tissue [Citation30]. Therefore, they are often used as drug carriers due to the characteristics of passive targeting, slow release, cellular affinity, and biocompatibility [Citation31–33]. Some relevant studies have demonstrated that nanoliposome coating can improve utilization and stability of drugs, and reduce toxic side effects in vivo [Citation34,Citation35]. Nanoliposome is also one of the clinically approved nano-drug carriers for tumor therapy [Citation36]. It has been confirmed that nanoliposomes can be used as drug delivery carriers in the treatment of lung diseases [Citation37].
At present, the preparation of nanoliposomes takes organic reagents with high toxicity like chloroform and ether as synthetic solvents, which has certain potential security problems. The nanoliposomes in this study were innovatively prepared by thin-film dispersion method with anhydrous ethanol as the lipid solvent (a green, safe and harmless solvent) (). The second difference of this study was that the encapsulation rate of the nanoliposome to GEB was maximized by optimizing the composition ratio of lecithin to cholesterol (LE/CH). Subsequently, a series of performance tests such as Zeta potential, drug release behavior, MTT, and anti-tumor effect in vivo were conducted, so as to explore whether the compound of GEB embedded by nanoliposomes (GL) had significant tumor-inhibiting ability.
2. Materials and methods
2.1. Materials
Gefitinib (GEB, C22H24ClFN4O3, 99%), lecithin (LE, C42H80NO8P, 98%), DL-Malic acid (C4H6O5, 99%) and cholesterol (CH) (>98%) were purchased from Shanghai Aladdin Bio-Chem Technology Co., LTD. Anhydrous ethanol (C2H6O, 99.5%), sodium dihydrogen phosphate (NaH2PO4, 99%), Disodium hydrogen phosphate (Na2HPO4, 99%) and Triton X-100, 98% were obtained from Hangzhou Lanbao Biological Co., LTD.
A549 lung cancer cells (ZQ0003) and 16HBE human bronchial epithelial cells (ZQ0001) were purchased from Shanghai Zhong Qiao Xin Zhou Biotechnology Co., Ltd. and cultured in Dulbecco’s Modified Eagle’s medium (DMEM; Gibco, MA, USA). Fetal bovine serum (FBS; <10 EU/ml) and double antibodies (penicillin, streptomycin; 10000 U/ml, 10 mg/ml) were from Gibco. BALB/c nude mice (6 weeks, 15–20 g) were provided by Shanghai SLAC Laboratory Animal Co., Ltd.
2.2. Methods
2.2.1. Preparation of GL
In this study, thin-film dispersion method was used to synthesize GL. A certain amount of LE, CH and GEB (dissolved in DL-Malic acid) were accurately weighed and transferred to a pear-shaped glass bottle. The bottle was added with 30 mL ethanol, heated, and treated by ultrasonic wave for a few minutes. The liquid in the bottle was then evaporated gradually at 40°C to form a dense thin film. On the other hand, 30 mL phosphate buffer solution (PBS) was absorbed and added into a 40 °C water bath for preheating. Afterward, the above solution was poured into the pear-shaped bottle, mixed with the liposome membrane, and hydrated at 40°C for 30 min. After the reaction, the solution was placed on a magnetic stirrer at room temperature for 30 min, treated by ultrasonic wave for 2 min, and extruded by a 220 nm polycarbonate membrane filter to obtain GL.
2.2.2. Transmission electron microscopy (TEM), particle size and potentiometric analyses
TEM analysis: the samples were vortex-diluted 20 times using ultrapure water with ultrasound in bath type ultrasonic instrument for 1 min. Then, the samples were impregnated with uranyl dye at an alcohol concentration of 70%. TEM (JM-2100, Japanese Electronics Co., Ltd., Tokyo, Japan) was used for analysis after samples were dried at room temperature.
Particle size and Zeta potential analyses: The particle size and Zeta potential of liposomes were measured on Malvern Zetasizer Nano ZS90 (Malvern Panalytical Ltd., UK) using dynamic light scattering (DLS). Before detection, the samples were diluted in ultrapure water and PBS (pH 7.4). The test temperature was 25°C, and the average value was taken after three detections.
2.2.3. Determination of encapsulation efficiency and in vitro release characteristics
An ultraviolet-visible spectrophotometer (Shimadzu; UV-3600) was used to detect GL encapsulation efficiency. Then, 0.5 mL GL and blank control of nanoliposomes were taken out and dissolved in 10 mL centrifuge tubes, respectively. The samples were centrifuged for 1 h at 1 × 104 rpm. The supernatant of GL group was removed and its absorbance was measured at the maximum absorption wavelength (λGEB = 330 nm) to obtain the concentration of free GEB (C1). Then, another 0.5 mL GL and blank control of nano liposomes were absorbed from the original samples, respectively, which were added with 4.5 mL intraprep permeabilization reagent triton X-100 solution (10%). The solution was shaken up-and-down and centrifuged. The absorbance of the supernatant was measured to obtain the concentration of total GEB (C2). The encapsulation efficiency was calculated by formula (2–1).
C0 was the initial addition amount of GEB, C1 was the content of free GEB in GL, and C2 was the content of GEB after the membrane of GL was broken.
The prepared GL and the GEB standardized solution in same concentration were placed into the treated dialysis bags, respectively, and the mouth of the bags was sealed with the dialysis tubing closures. The bags were then put into PBS containing 30% methanol and stirred uniformly at 100 r/min. The temperature of PBS was controlled at 37°C ± 0.5°C. The 0.5 ml PBS was taken at several time points, respectively. After sampling, PBS containing 30% methanol should be supplemented until the solution reached the initial scale. The PBS was filtered by a 0.22-μm microfiltration membrane. Ultraviolet (UV) detection was performed and the cumulative release percentage was calculated by using formula (2–2).
Ct was the drug concentration sampled at time t and C0 was the drug-loading concentration of prepared GL.
2.2.4. Evaluation of cell viability
The cells were inoculated into 96-well plates at a concentration of 5 × 104 cells/well and treated with different concentrations of GEB and GL (CGEB = 0, 10, 20, 30, 40, and 50 μg/mL). Meanwhile, cells treated with PBS were used as the control group with three replicates in each group. After continuous culture for 24 h, the culture medium was removed and MTT solution (200 μL, 0.5 mg/mL) was added to each well and cells were incubated at 37°C for 4 h. The medium was discarded and 150 μL dimethyl sulfoxide (DMSO) was added to dissolve blue-purple crystal formazan. The optical density (OD) value of each well at 570 nm was read by a microplate reader, and the cell viability of different groups (the ratio of OD values between the experimental group and the control group) was calculated. Finally, the IC50 value was generated by GraphPad.
2.2.5. Cell apoptosis assay
In order to explore the apoptosis mechanisms of cancer cells at different stages, the flow cytometer (BD, Accuri C6, USA) was used to evaluate the apoptosis. A549 cells were seeded on a six-well plate at a density of 105 cells/well and cultured for 24 h. Next, sample solution was added to each well, respectively (PBS was taken as the control group). After treated for 24 h, cells were collected by centrifugation and treated by using Annexin V-FITC apoptosis kit (Solarbio Life Sciences, Beijing, China). Finally, cells were analyzed by flow cytometry.
2.2.6. Colony formation assay
After treated with PBS (control group), GEB, and GL (CGEB = 50 μg/mL) for 48 h, the cells were inoculated into 6-well plates at a density of 500 cells/well. Cells were then cultured in a fresh medium for 1 week, fixed with paraformaldehyde, and stained with crystal violet (Beyotime Biotechnology). Thereafter, the number of colonies was calculated [Citation38].
2.2.7. Scratch and Transwell invasion assays
Scratch assay: A549 cells were spread on a 24-well plate (500 μL per well) at a density of 5 × 105 cells/mL and cultured in DMEM containing 10% FBS for 24 h to form monolayer cells. Scratches were made on the monolayer cells by a 200 μL pipette. After washing with PBS for 3 times, cells were put into different treatment groups (GEB and GL), and PBS was used as the control group. Two parallel samples were incubated for 24 h and then cultured in DMEM containing 2% FBS for another 24 h. The culture medium was discarded, and cells were washed three times with PBS, observed as well as photographed under an inverted optical microscope (Leica, Weizlar, Germany).
Transwell invasion assay: A549 cells were washed with PBS, and suspended by serum-free medium until the cell density was adjusted to 1 × 105 cells/ml. The 600 μL medium containing 10% FBS was added to the lower chamber of a 24-well plate, and the Transwell chamber was placed in the 24-well plate. The 200 μL cell suspension was added into the upper chamber, and the cells were administrated (PBS was the control group), and cultured in an incubator for 24 h. Then, the chamber and medium were removed, and cells in the Matrigel and the upper chamber were wiped gently with a cotton swab. In the new 24-well plate, 4% paraformaldehyde (600 μL) was added and the chamber was fixed for 30 min. Then, the fixed solution was discarded, and cells were stained with 0.2% crystal violet for 10 min, washed 3 times with PBS to remove the crystal violet. After proper air-drying, 5 fields were randomly selected and observed under a high-power microscope to count the cells.
2.2.8. Cell cycle analysis
A549 cells were prepared into the single-cell suspension and inoculated evenly into a 6-well plate at a density of 1 × 105 cells/well. After incubation at 37°C with 5% CO2 overnight, the culture medium was replaced with 1 ml serum-free culture medium, and cells were starved at 37°C with 5% CO2 for 6 h. Then, the culture medium was removed and cells were cultured with GEB and GL solutions (CGEB = 0 μg/mL, 30 μg/mL, and 50 μg/mL) for 24 h, respectively. After culture, the culture medium was removed again, and cells were digested with 0.25% ethylene diamine tetraacetic acid (EDTA) free trypsin, and carefully blown using 5% cold ethanol to form the single-cell suspension, which was fixed overnight at 4°C. The next day, cells were centrifuged at 1500 r/min for 5 min, and washed with cold PBS for twice after discarding the supernatant. Prodium Iodide (PI) dye was added according to the standard steps of cell cycle, and cells were incubated at 37°C for 30 min. After filtering with a 300-mesh filter, the cells were tested on the flow cytometer (BD Accuri C6).
2.2.9. Anti-tumor effect in vivo
BALB/c nude mice were used to establish animal tumor models. A549 cells (density of 1 × 106) containing 100 μL PBS were subcutaneously injected into the right side of mice aged 4 to 6 weeks. When the tumor volume reached about 50 mm3, mice with A549 tumor cells were randomly divided into 3 groups (n = 5) and treated with PBS, free GEB solution, and GL, respectively. Each mouse was injected with 200 μL PBS, free GEB solution, and GL every 2 days (5 mg/kg GEB, 5 times in total), respectively. The tumor volume was measured by a vernier caliper and calculated by formula (2–3). The corresponding mouse weight and tumor weight were also recorded.
L and W were the length and width of the tumor, respectively, and V was the volume of the tumor.
2.2.10. Immunohistochemistry (IHC) experiment
After euthanasia of mice, tumor blocks in nude mice were removed and tumor tissue in each group was fixed with formalin and embedded in paraffin. The tissue was sliced (4 μm), dewaxed with xylene, dehydrated with graded alcohol, and hydrated. After that, hematoxylin and eosin (H&E) staining and immunohistochemical evaluation were performed on the tissue sections. In IHC experiment, protein antibody (Anti Ki-67, 1:200, Dako, CA, USA), receptor-tyrosine kinase (EGFR, 1:200, Abcam, Cambridge, MA, USA) and estrogen receptor (ER-α, 1:100, Santa Cruz, CA, USA) were used as positive and negative controls, respectively. The processes were as follows: the rinsed tissue sections were firstly incubated with appropriate biotinylated secondary antibody, goat anti-mouse or goat anti-rabbit (Dako, Campbellfield, Australia), and then streptavidin-horseradish peroxidase complex (BD Pharmingen, CA, USA) was added for further incubation. Finally, color was developed with 3, 3ʹ -diaminobenzidine (Dako liquid DAB Plus, K3468) for 5 min, and samples were observed under an optical microscope (LEICA, Wetzlar, Germany).
3. Results
3.1. Materials characterization
GL nanocomposite was successfully prepared by green method and thin film hydration method, and its morphology was characterized by particle size, Zeta potential and TEM (). ) shows that compared with the blank liposome (d = 169.6 nm), the average particle size of GL had a certain increase (d = 190 nm). The polymer dispersion index (PDI) of GL was 0.248, indicating that the particle size distribution of the nanoliposome was relatively uniform ()). The Zeta potentials of the blank liposome and the GL are exhibited in ). The results showed that their surface Zeta potentials were both negative, which were −15.55 mV and −8.91 mV, respectively. Nanoparticles with negative charge on the surface were hard to be adsorbed with proteins in the blood. Therefore, GL could reach the tumor site successfully and reduce toxicity in vivo. In addition, the morphology of GL was also studied by TEM ()). It could be observed that GL nanoparticles had a complete spherical structure with good dispersion and no agglomeration. Meanwhile, its particle size was less than 200 nm, which was consistent with the results of DLS particle size analysis. The above results proved that the prepared nanoliposome achieved the expectation. The particle size, Zeta potential, and structure of the prepared nanoliposome would be beneficial to prolong the circulation time of GEB in vivo, and facilitate the aggregation as well as internalization in tumor tissue to achieve satisfactory anticancer effect.
3.2. Study on encapsulation efficiency and in vitro release performance
Relevant data demonstrate that higher CH content in a certain limit increases the various indexes of liposomes. In other words, the “rigidity” of liposome membrane can be adjusted by CH content, so as to improve its permeability, stability, and encapsulation efficiency. However, when the proportion of CH is too high, the encapsulation efficiency shows a downward trend [Citation39]. Hence, the ratio of lecithin to cholesterol (LE/CH) was adjusted to improve the stability and encapsulation efficiency of nanoliposome in this study. It could be concluded from the results in ) that the ratio of LE/CH had a great impact on GL encapsulation efficiency. When LE/CH value (oval-biliary ratio) was between 2 and 3, GL encapsulation efficiency rose linearly. When LE/CH value was greater than 3, the encapsulation efficiency decreased gradually, which reached its maximum (60.26%) with the LE/CH value of 3. In summary, the stability and encapsulation efficiency of nanoliposome could be significantly improved with appropriate cholesterol proportion.
Figure 2. Study on encapsulation efficiency and release behavior of nanoliposome
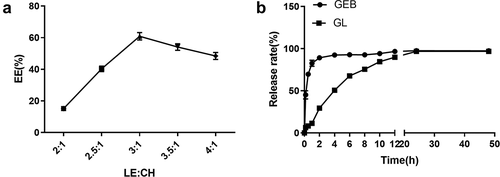
In order to further confirm the stability and sustained-release function of the synthesized nanoliposome, in vitro drug release experiments of GEB solution and GL were carried out. According to the results in ), the overall release rate of GEB standardized solution was fast within 0 to 12 h, and it was released rapidly within 2 h, with the cumulative release rate greater than 80%. In contrast, GL released more slowly, and the cumulative release rate of the system reached 80% at about 12 h. This indicated that GL nanocomposite structure had a certain stability and a better sustained-release effect than GEB solution, thus effectively prolonging the action time of the drugs.
3.3. Study on anti-tumor effect in vitro
First, PBS was used as the control group to evaluate the effect of pure GEB and GL nanocomposite liposome on viability of A549 cells and 16HBE cells by MTT assay.
) shows that with the increase of drug dose, the A549 cell viability in the pure GEB group and the GL group gradually decreased, presenting a dose-dependent pattern. Meanwhile, according to the data in ), the IC50 of A549 cells in the pure GEB group was 41.96 μg/mL, while that in the GL group was only 29.63 μg/mL, which was significantly lower. The above results suggested that GL had a more prominent inhibiting effect on A549 cells compared with the pure GEB group. Interestingly, 16HBE cells treated with GEB and GL showed little difference in their cell viability, which further confirmed the above conclusion.
Figure 3. Effects of GL on viability and apoptosis of A549 cells and 16HBE cells
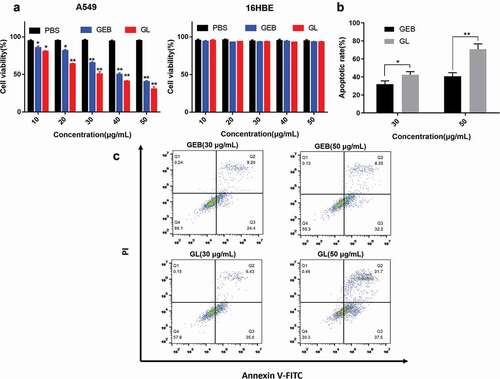
At the same time, the apoptosis mechanism of cancer cells also reflects the anti-cancer ability of anticancer drugs to some extent. Therefore, the apoptosis of each group was also analyzed by flow cytometry (). In this study, A549 cells were treated with 30 μg/mL GEB and 50 μg/mL GL, respectively, to further investigate the correlation between apoptosis rate of cancer cells and drug concentration. It could be concluded that with the increase of drug concentration, the apoptosis rate of A549 cells in GEB and GL groups increased gradually. In addition, under the same drug concentration, GL group showed stronger apoptotic ability than GEB group. Especially under the condition of high drug concentration, the apoptosis rate of the two groups was remarkably different.
Colony formation, scratch, and transwell invasion assays of A549 cells were performed after treatment of pure GEB and GL, respectively. ) exhibits the colony proliferation of A549 cells treated with the same concentration of GEB and GL. The clone number of tumor cells in GEB and GL groups was less than that in PBS control group. In particular, compared with the GEB group, tumor cell proliferation was effectively inhibited after treated with GL, and the clone number of A549 tumor cells was much less than that of the pure GEB group. The results of scratch assay of each treatment group after 0 h and 24 h are presented in ), in which the cell migration ability could be determined by the width change between the scratches. It was observed that compared with 0 h, the scratch widths of GEB and GL groups were narrower after 24 h, but both of them were greater than that of PBS control group. Moreover, the scratch width of GL group was significantly greater than that of GEB group, which proved that the cell migration rate of GL group was significantly lower than that of GEB group, that was GL had a stronger inhibiting ability on A549 cells. On the other hand, in Transwell invasion assay, the invasion ability of A549 tumor cells treated with GL was also dramatically lower than that of the GEB group ()). Based on the above results, it could be concluded that the ability of GET to inhibit the proliferation, migration, and invasion of tumor cells had been significantly enhanced after nanoliposome embedding modification.
Figure 4. Colony formation, scratch and Transwell invasion assays of A549 cells
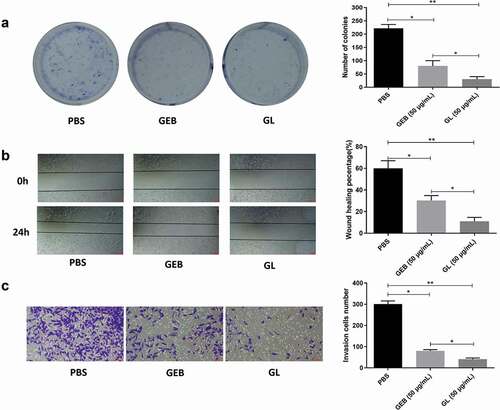
The cycle stagnation of cancer cells is one of the main reasons for of cell growth inhibition, and it is also a sign of the anti-cancer ability of drugs [Citation40]. reflected the cycle distribution of A549 cells treated with different concentrations of GEB and GL, respectively. Most of the A549 cells in PBS, GEB, and GL groups were blocked in the G0/G1 phase after drug administration, especially the cells treated with GL (). Moreover, with the gradual increase of anticancer drug concentration, the number of cancer cells in G0/G1 phase showed a steadily rising trend. The statistical data in ) further proved the above conclusions. In addition, compared with the GEB group, the number of cells in G0/G1 phase was significantly greater in the GL group, indicating that GL could inhibit the normal operation of the cell cycle more effectively, thus inhibiting the proliferation of tumor cells.
Figure 5. Cell cycle comparisons among different treatment groups
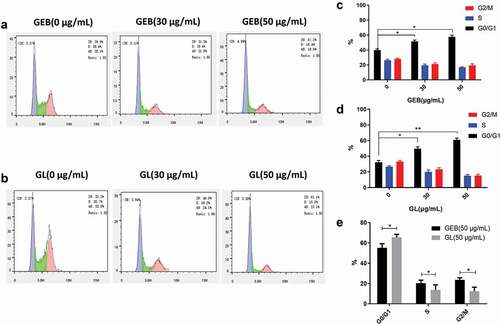
3.4. Anti-cancer effect in vivo
In order to verify whether the anti-cancer effect of GL was improved in vivo, we conducted a series of anti-cancer experiments in mice with PBS and GEB as controls. presents the in vivo anti-cancer evaluation results of BALB/c nude mice lung cancer models treated with PBS, GEB and GL, respectively. The change trend of tumor size in ) implied that with the extension of treatment time, tumor size in the PBS and GEB groups also increased gradually. Especially in the PBS group, tumor size reached 800 mm3 after 14 d of treatment. On the contrary, the tumor size in the GL group remained unchanged, which was still only 100 mm3 after 14 d. The average tumor weight of the GL group after 14 d was also the smallest (0.066 g) compared with the other two groups, whereas that of the PBS group reached 1.167 g ()). Meanwhile, the results in ) also showed that the average tumor volume trend of the three groups was consistent with the trend in ). Based on the above results, GL could effectively inhibit the growth rate of lung cancer. In order to display the tumor inhibition effect of each group more intuitively, the final digital tumor photos of PBS, GEB, and GL groups are presented in ). It could be clearly noted that the tumor-inhibiting effect of GL group after 14 d was particularly significant in comparison with the other two groups. On the other hand, the body weight changes of mice in PBS, GEB, and GL groups were also measured to assess the potential toxicity of GL in mice. The results showed that the weight changes of the mice in these groups were similar ()), which partly proved that GL had low toxicity in vivo.
Figure 6. Evaluation of anti-cancer effect in vivo of different treatment groups
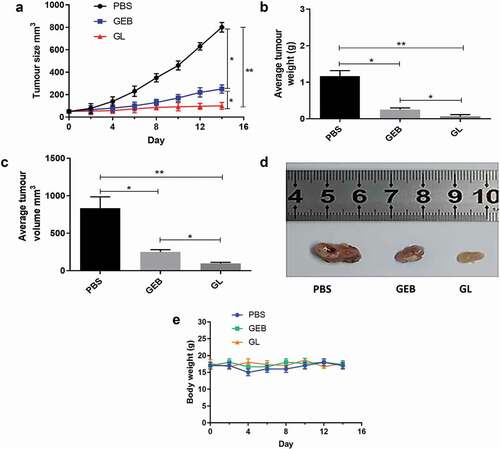
Finally, the mice were euthanized after treatment, and tumors in vivo were removed for H&E staining and immunohistochemical treatment (). The H&E staining results suggested that tumor tissue of the PBS and GEB groups was not prominently different. In contrast, the connective tissue area of GL group was obviously reduced, which suggested that the inhibiting ability of GEB to tumor was greatly improved after embedding and modification of nanoliposome. At the same time, protein antibody (Anti KI-67), receptor-tyrosine kinase (EGFR) and estrogen receptor (ER-α) were used as immunohistochemical indexes in this study. It was also noted that the expression of Ki-67 proliferation marker protein in GL group was significantly down-regulated, indicating that the proliferation of cancer cells in tumor tissue was greatly suppressed. The underlying mechanism may be that EGFR activity was related to the regulation of ER-α expression. When GL was degraded, the GEB released by GL would firstly reduce the expression of ER-α, then inhibit the participation of EGFR, and finally achieve the desired anti-cancer effect.
4. Discussion
GL was prepared by thin-film dispersion method and its stability was evaluated in this study. The particle size and encapsulation efficiency were 189 nm and 60.26%, respectively, indicating that the nanoliposome had ideal particle size and encapsulation efficiency. Besides, small PDI and uniform particle size are conducive to enter into tumor tissue and exert curative effect, thus achieving desired anti-cancer effect. In this study, by adjusting the ratio of LE/CH, the stability, encapsulation efficiency and in vivo cycling time of the nanoliposome composite drug were effectively improved, and GL with smaller particle size was obtained. The effects of GEB and GL on A549 lung cancer cells were investigated, and the anti-cancer activities of GEB and GL in mice tumor models were further verified. The results demonstrated that GL could effectively reduce the proliferation, migration, and invasion of A549 cells and induce apoptosis. It could also enhance GEB ability to block cells in the G0/G1 phase, thereby killing cancer cells. Simultaneously, compared with pure GEB, the nanoliposome carrier could avoid GEB being affected by internal environment, thus effectively enhancing the treatment rate of tumors. Anti-cancer tests in vivo also further confirmed the high efficiency of GL. Therefore, this nanoliposome composite drug is expected to provide a green and efficient solution to the current treatment problem of NSCLC.
Highlights
Gefitinib nano-liposomes with better encapsulation efficiency and smaller particle size are obtained by low toxic and high effective methods;
The drug encapsulation efficiency of nano-liposomes is maximized by exploring the ratio of phosphatidylcholine to cholesterol;
The structure of gefitinib nano-liposomes can prolong the action time of drugs and effectively improve tumor-inhibiting ability.
Authors’ contributions
All authors made substantial contributions to conception and design, acquisition of data, or analysis and interpretation of data; took part in drafting the article or revising it critically for important intellectual content; gave final approval of the version to be published; and agree to be accountable for all aspects of the work.
Consent for publication
All authors consent to submit the manuscript for publication.
Disclosure statement
The authors declare that they have no potential conflicts of interest.
Additional information
Funding
References
- Bray F, Ferlay J, Soerjomataram I, et al. Global cancer statistics 2018: GLOBOCAN estimates of incidence and mortality worldwide for 36 cancers in 185 countries. CA Cancer J Clin. 2018;68:394–424.
- Liu Q, Bai W, Huang F, et al. Downregulation of microRNA-196a inhibits stem cell self-renewal ability and stemness in non-small-cell lung cancer through upregulating GPX3 expression. Int J Biochem Cell Biol. 2019;115:105571.
- Raez LE, Cardona AF, Santos ES, et al. The burden of lung cancer in Latin-America and challenges in the access to genomic profiling, immunotherapy and targeted treatments. Lung Cancer. 2018;119:7–13.
- Deng Q, Fang Q, Xie B, et al. Exosomal long non-coding RNA MSTRG.292666.16 is associated with osimertinib (AZD9291) resistance in non-small cell lung cancer. Aging (Albany NY). 2020;12(9):8001–8015.
- Dijkstra KK, Monkhorst K, Schipper LJ, et al. Challenges in establishing pure lung cancer organoids limit their utility for personalized medicine. Cell Rep. 2020;31(5):107588.
- Liu D, Cao Z, Xu W, et al. Enhancement of chemosensitivity by WEE1 inhibition in EGFR-TKIs resistant non-small cell lung cancer. Biomed Pharmacothe. 2019;117:109185.
- Gala UH, Miller DA, Williams RO 3rd. Harnessing the therapeutic potential of anticancer drugs through amorphous solid dispersions. Biochim Biophys Acta Bioenerg Reviews on Cancer. 2020;1873:188319.
- Park K. Controlled drug delivery systems: past forward and future back. J Control Release. 2014;190:3–8.
- Wang Y, Zhao Q, Han N, et al. Mesoporous silica nanoparticles in drug delivery and biomedical applications. Nanomedicine. 2015;11(2):313–327.
- Karthivashan G, Ganesan P, Park SY, et al. Therapeutic strategies and nano-drug delivery applications in management of ageing Alzheimer’s disease. Drug Deliv. 2018;25:307–320.
- Shen J, Lu GW, Hughes P. Targeted ocular drug delivery with pharmacokinetic/pharmacodynamic considerations. Pharm Res. 2018;35:217.
- Roskoski R Jr. Small molecule inhibitors targeting the EGFR/ErbB family of protein-tyrosine kinases in human cancers. Pharmacol Res. 2019;139:395–411.
- Supuran CT. Carbonic anhydrase inhibitors as emerging agents for the treatment and imaging of hypoxic tumors. Expert Opin Investig Drugs. 2018;27:963–970.
- Cheng Y, Qi Y. Current progresses in metal-based anticancer complexes as mammalian TrxR inhibitors. Anticancer Agents Med Chem. 2017;17:1046–1069.
- Sidera K, Patsavoudi E. HSP90 inhibitors: current development and potential in cancer therapy. Recent Pat Anticancer Drug Discov. 2014;9:1–20.
- Häntschel M, Niebling J, Häring A, et al. Life-threatening pneumonitis after first-line treatment with osimertinib for primary T790M mutated non-small cell lung cancer. Thorac Cancer. 2020;11(7):2044–2047.
- Sari M, Aydiner A. Rare mutations of epidermal growth factor receptor in epidermal growth factor receptor-tyrosine kinase inhibitor-naive non-small cell lung carcinoma and the response to erlotinib therapy. J Cancer Res Ther. 2020;16:132–138.
- Alam MW, Borenäs M, Lind DE, et al. Alectinib, an anaplastic lymphoma kinase inhibitor, abolishes ALK activity and growth in ALK-positive neuroblastoma cells. Front Oncol. 2019;9:579.
- Sun Z, Zeng L, Zhang M, et al. PIM1 inhibitor synergizes the anti-tumor effect of osimertinib via STAT3 dephosphorylation in EGFR-mutant non-small cell lung cancer. Ann Transl Med. 2020;8(6):366.
- Yang JC-H, Cheng Y, Murakami H, et al. A randomized phase 2 study of gefitinib with or without pemetrexed as first-line treatment in nonsquamous NSCLC With EGFR mutation: final overall survival and biomarker analysis. J Thorac Oncol. 2020;15(1):91–100.
- Lai K-C, Chueh F-S, Hsiao Y-T, et al. Gefitinib and curcumin-loaded nanoparticles enhance cell apoptosis in human oral cancer SAS cells in vitro and inhibit SAS cell xenografted tumor in vivo. Toxicol Appl Pharmacol. 2019;382:114734.
- Shariati A, Habibi M, Tounsi A, et al. Application of exact continuum size-dependent theory for stability and frequency analysis of a curved cantilevered microtubule by considering viscoelastic properties. 2020;1–20.
- Zhang X, Shamsodin M, Wang H, et al. Dynamic information of the time-dependent tobullian biomolecular structure using a high-accuracy size-dependent theory. J Biomol Struct Dyn. 2020;1–16. DOI:10.1080/07391102.2020.1760939
- Najaafi N, Jamali M, Habibi M, et al. Dynamic instability responses of the substructure living biological cells in the cytoplasm environment using stress-strain size-dependent theory. J Biomol Struct Dyn. 2020;1–12. DOI:10.1080/07391102.2020.1751297
- Abdelmalek Z, Karbon M, Eyvazian A, et al. On the dynamics of a curved microtubule-associated proteins by considering viscoelastic properties of the living biological cells. J Biomol Struct Dyn. 2020;1–15. DOI:10.1080/07391102.2020.1747549
- Zhang P, Ge Z, Safarpour M. Size-dependent dynamic stability analysis of the cantilevered curved microtubule-associated proteins (MAPs). J Biomol Struct Dyn. 2020;1–13. DOI:10.1080/07391102.2020.1758211
- Tang M, Svirskis D, Leung E, et al. Can intracellular drug delivery using hyaluronic acid functionalised pH-sensitive liposomes overcome gemcitabine resistance in pancreatic cancer? J Control Release. 2019;305:89–100.
- Kahana M, Weizman A, Gabay M, et al. Liposome-based targeting of dopamine to the brain: a novel approach for the treatment of Parkinson’s disease. Mol Psychiatry. 2020. DOI:10.1038/s41380-020-0742-4
- Dharmaraj K, Román Silva JI, Kahlert H, et al. The acid-base and redox properties of menaquinone MK-4, MK-7, and MK-9 (vitamin K(2)) in DMPC monolayers on mercury. Eur Biophys J. 2020;49:279–288.
- Yang B, Chen Y, Tumor-Specific SJ. Chemotherapy by nanomedicine-enabled differential stress sensitization. Angew Chem Int Ed Engl. 2020;59:9693–9701.
- Elnaggar YSR, Omran S, Hazzah HA, et al. Anionic versus cationic bilosomes as oral nanocarriers for enhanced delivery of the hydrophilic drug risedronate. Int J Pharm. 2019;564:410–425.
- Yu L-M, Liu Y-L, Zhu L-B, et al. Boosting the biocatalytic precipitation with enzyme-loaded liposomes: toward a general platform for amplified photoelectrochemical immunoassay. Anal Chim Acta. 2020;1115:1–6.
- Zhi LJ, Sun AL, Tang D. In situ amplified photothermal immunoassay for neuron-specific enolase with enhanced sensitivity using Prussian blue nanoparticle-loaded liposomes. Analyst. 2020;145:4164–4172.
- Zhang X, Liu Y, Luo L, et al. A chemo-photothermal synergetic antitumor drug delivery system: gold nanoshell coated wedelolactone liposome. Mater Sci Eng C Mater Biol Appl. 2019;101:505–512.
- de Freitas CF, Montanha MC, Pellosi DS, et al. Biotin-targeted mixed liposomes: a smart strategy for selective release of a photosensitizer agent in cancer cells. Mater Sci Eng C Mater Biol Appl. 2019;104:109923.
- Zhou C, Xie X, Yang H, et al. Novel class of ultrasound-triggerable drug delivery systems for the improved treatment of tumors. Mol Pharm. 2019;16(7):2956–2965.
- Dianat-Moghadam H, Heidarifard M, Jahanban-Esfahlan R, et al. Cancer stem cells-emanated therapy resistance: implications for liposomal drug delivery systems. J Control Release. 2018;288:62–83.
- Chen L, Cheng X, Tu W, et al. Apatinib inhibits glycolysis by suppressing the VEGFR2/AKT1/SOX5/GLUT4 signaling pathway in ovarian cancer cells. Cell Oncol (Dordr). 2019;42(5):679–690.
- Yu M, Song W, Tian F, et al. Temperature- and rigidity-mediated rapid transport of lipid nanovesicles in hydrogels. Proc Natl Acad Sci U S A. 2019;116(12):5362–5369.
- Tian T, Zhang H-X, He C-P, et al. Surface functionalized exosomes as targeted drug delivery vehicles for cerebral ischemia therapy. Biomaterials. 2018;150:137–149.