ABSTRACT
Radiation-induced liver disease (RILD), also known as radiation hepatitis, is a serious side effect of radiotherapy (RT) for hepatocellular carcinoma. The therapeutic dose of RT can damage normal liver tissue, and the toxicity that accumulates around the irradiated liver tissue is related to numerous physiological and pathological processes. RILD may restrict treatment use or eventually deteriorate into liver fibrosis. However, the research on the mechanism of radiation-induced liver injury has seen little progress compared with that on radiation injury in other tissues, and no targeted clinical pharmacological treatment for RILD exists. The DNA damage response caused by ionizing radiation plays an important role in the pathogenesis and development of RILD. Therefore, in this review, we systematically summarize the molecular and cellular mechanisms involved in RILD. Such an analysis is essential for preventing the occurrence and development of RILD and further exploring the potential treatment of this disease.
METHODS:
1. We used PubMed and University of SouthChina Library to retrieve and download literature.
2. We identified studies on radiation-inducedliver damage, ionizing radiation, radiation and liver cancer, liver DNA damage, radiation and liver immune damage, microRNA and radiation-induced liver damage, radiation and inflammation, radiation and aging, radiation and mitochondrial damage, radiation and Kupffer cells, hepatic stellate cells, and sinusoidal endothelial cells.
3. Inclusion criteria included experimentalarticles and comments on postradiation liver tissue and cells (human and mouse)or experimental articles and comments on radiation-induced DNA damage in liver tissue and hepatocytes (human and mouse).
4. Exclusion criteria included experimental articles on other organs and cancers and reviews of radiation damage; articles that are too old; non-English research and conference abstracts; and withdrawn articles.
1. Introduction
The statistics of the International Agency for Research on Cancer show that since 2020, hepatocellular carcinoma (HCC) has become the sixth cancer with the third highest morbidity and mortality worldwide, accounting for 8.3% of the total cancer deaths [Citation1,Citation2]. HCC has a complex pathogenesis, a high degree of malignancy, and easy metastasis and recurrence. Most patients with liver cancer are diagnosed in the late stage of the disease. This situation greatly limits the treatment of liver cancer. Ionizing radiation (IR) is widely involved in medical diagnosis or treatment and is particularly important in nuclear medicine and RT. RTX, which includes internal RT and radioisotopes, can be used in all stages of liver cancer and has thus become a common method for the treatment of this malignancy [Citation3,Citation4]. However, because the liver is sensitive to radiation, radiotoxicity usually accumulates in the normal tissues around the tumor, resulting in 24.7% of patients developing varying degrees of RILD within 22 months after RT [Citation5]. RILD is a form of subacute liver injury caused by RT. In 1965, Ingold et al. proposed that RT causes RILD [Citation6], which is the most common and fatal complication after RT. Radiation doses of 50–70 Gy are considered to be effective for controlling most solid malignant tumors; however, approximately 5%–10% of patients develop classic RILD when their whole liver is exposed to the cumulative dose of 30–35 Gy [Citation7,Citation8]. In addition to HCC, some upper abdominal tumors with poor chemotherapeutic drug selectivity may cause RILD due to RT [Citation9]. Given that the liver is large and adjacent to the gastrointestinal tract, it is also exposed to IR during RT for gastrointestinal cancer [Citation10]. After receiving neoadjuvant chemoradiotherapy, 10% of patients with esophageal cancer develop liver metastasis and RILD [Citation11]. Hematopoietic stem cells (HSCs) and bone marrow transplantation may also cause RILD [Citation9]. The accumulation of toxicity in normal liver tissue after IR may restrict the use of this treatment or produce serious chronic side effects, such as radiation-induced bystander effects (RIBE) in paracancerous cells, radiation adaptive response and genomic instability, parenchyma cell loss and excessive fibrous tissue formation, and even radiation-induced liver fibrosis (RILF) and cirrhosis in the later stage. Therefore, normal liver tissue injury is an important factor limiting the RT of liver tumors and is closely related to the high mortality of liver cancer [Citation8].
In addition, some studies have found that the interaction between hepatocytes and hepatic nonparenchymal cells (NPCs) can lead to hepatocyte damage. For example, Kupffer cells (KCs), hepatic stellate cells (HSCs), and hepatic sinusoidal endothelial cells (SEC) are radiosensitive. The radiation damage of these cells in the body can cause inflammation and apoptosis and promote the production of related cytokines. This situation leads to the abnormal structure and function of the liver during radiation treatment, and eventually RILD transforms into liver fibrosis. Moreover, NPCs are induced to produce a large number of growth factors and chemokines, thus prompting the accumulation of macrophages, lymphocytes, and other inflammatory cells to form a tumor-promoting microenvironment for tumor cell proliferation and invasion [Citation8,Citation12–14]. RILD is usually accompanied by changes in the tumor microenvironment (TME) and intestinal microflora; DNA damage; and immune system responses, such as the release of microbe-associated molecular patterns after tissue injury. Microorganisms may control the direct nuclear effect or bystander effect of IR, thus triggering a series of cellular responses [Citation10,Citation14]. In addition to the immune system activation caused by DNA damage, RT can induce immunosuppression and recruit immunosuppressive T regulatory cells and tumor-associated macrophages to the TME [Citation9,Citation11].
In this review, we summarize and discuss the effects of radiation on the molecular and cellular mechanism of RILD to summarize the potential molecular targets in RILD, develop new therapeutic strategies, prevent the occurrence of RILD, inhibit RILD, and improve the effect of RT.
2. Molecular mechanism of RILD
Radiation-induced DNA damage (RIDD) mainly includes direct damage to DNA molecules caused by the interaction between charged particles (electrons or ions) and DNA chains in the nucleus and by oxidative stress induced by reactive oxygen species (ROS) and reactive nitrogen species (RNS) and their binding into peroxynitrite anions (ONOO−), thus inflicting indirect damage through various processes, such as base oxidation induction, purine site removal, and helix unwinding [Citation15–17]. IR-induced extracellular water decomposition results in a significant increase in exogenous ROS [Citation18]. ROS-induced DNA damage accounts for 65% of RIDD [Citation19]. Therefore, intracellular antioxidants can reduce the ability of IR to cause DNA damage. Organisms have evolved complete endogenous enzymes and antioxidant systems for the repair of DNA damage caused by the production of ROS to adapt to the environment. These adaptations include antioxidant enzymes, such as glutathione peroxidase, catalase (CAT), superoxide dismutase (SOD), and detoxifying enzyme cytochrome P450 [Citation20], as well as the blockage of the oxidation chain by increasing the level of glutathione (GSH), scavenging free radical molecules, and inhibiting lipid peroxidation (LPO) [Citation21] to regulate the imbalance of the reduction/oxidation (redox) system [Citation22]. In addition, inflammatory cells trigger inflammatory responses by releasing high mobility group protein 1, adenosine triphosphate, heat-shock protein, inflammatory bodies, and other molecules after IR-induced programmed cell death, such as apoptosis, scorch death, and autophagy. The transfer of cytokines and chemokines to neighboring cells or organs leads to the activation of inflammatory response, thus inducing oxidative damage and DNA damage in nonirradiated cells and RIBE [Citation23,Citation24]. Compared with that on other tissues, research on the mechanism of radiation injury in liver tissue is scant.
2.1. RIDD ()
IR activates the complex and highly regulated DDR in cells. DDR plays an important role in the occurrence and development of RILD. DDR is mainly executed by three protein kinases related to the phosphatidylinositol 3 kinase family: ataxia telangiectasia mutation (ATM), ataxia – telangiectasia and Rad3-related (ATR), and DNA-dependent protein kinase catalytic subunit (DNA-PKcs). RIDD includes single-stranded (ssDNA) and double-stranded DNA (dsDNA). DNA double-stranded breaks (DSBs) are not only an important factor leading to RIDD, they are also particularly fatal lesions. When they cannot be repaired in time, they lead to cell senescence, death, and even canceration [Citation25]. ATM and DNA-PKcs are activated in DSB, and ATR interacts with ssDNA in response to various types of DNA damage [Citation26]. As a key molecule of HR repair, RAD51 has recombinant enzyme activity and can rapidly repair DSBs. Aobama et al. found that radiation enhances the expression of the RAD51-associated protein-1 gene and promotes the growth of intrahepatic cholangiocarcinoma [Citation27]. Li et al. discovered that low-dose irradiation could induce mild oxidative stress and promote histone deacetylation to inhibit the expression of miR-193b-3p then increase the expression of Rad51 by activating the DNA repair response instead of causing DDR [Citation28]. Furthermore, Cai et al. discovered that IR promotes the binding of histone deacetylase 4 to RAD51 nuclear translocation and ubiquitin binding enzyme 9 (Ubc9), resulting in the acetylation of Ubc9 and enhancing the radiation sensitivity of cells [Citation29]. IR can also produce oxidative damage through the formation of cluster/complex DNA damage (CDD) [Citation30]. CDDs need to activate the repair system for a long time. This requirement is related to the incomplete repair of damage sites and the increase in mutation rate, thus prompting genomic instability.
Figure 1. After IR exposure, DNA damage induces the formation of DNA strands, including ssDNA, dsDNA, and CDD (multiple damage sites) in the nucleus and direct damage to DNA. Water molecule ionizing inflicts induces the production of ROS.ATM: ataxia telangiectasia mutation; ATR: ataxia telangiectasia is related to rad3; DNA-PKcs: DNA-dependent protein kinase catalytic subunit; ATRIP: ATR interacting protein; ETAA1: Ewing tumor-associated antigen; TopBP1: topoisomerase II binding protein; 53bp1: p53 binding protein 1; γH2AX: phosphorylated histone H2AX; JNK: c-Jun amino terminal kinase; Rad51ap1:rad51 associated protein-1; HDAC4: histone deacetylase 4; Ubc9: ubiquitin-binding enzyme 9; TGF-α: transforming growth factor α; TNF-α: tumor necrosis factor α.
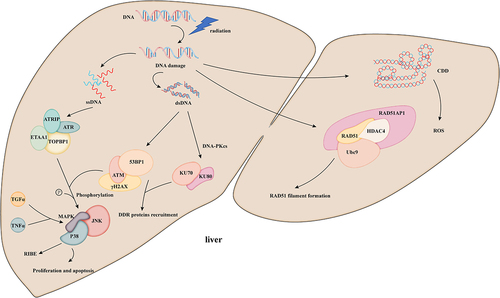
IR induces the phosphorylation of the MAPK superfamily by ATM/ATR, including the classic MAPK pathway extracellular signal-regulated kinase (ERK), c-jun N-terminal kinase (JNK), and p38MAPK (P38) [Citation31]. The activation of ERK, ATM, and AKT by IR in a DSB-dose-dependent manner increases cell proliferation and apoptosis [Citation32]. Similarly, JNK is activated by IR in an ATM-dependent manner and induces apoptosis. However, the binding site of its substrate and the recruitment of other kinases have not been further explored [Citation33]. In addition, p38 enzyme activity is induced after DNA damage, which affects cell proliferation through oxidative stress [Citation34]. After IR, autocrine growth factor ligands, such as transforming growth factor α (TGF-α) and tumor necrosis factor α (TNF-α), enhance the response of the intracellular MAPK pathway, thereby promoting the bystander effect in cells [Citation31].
This series of studies on DDR shows that DDR plays an important role in IR-induced RILD. However, the specific mechanism of this role is unclear and therefore needs further exploration.
2.2. RNAs mediate RILD
2.2.1. MicroRnas ()
MicroRNAs (miRNAs) participate in a variety of signaling pathways, such as hematopoiesis, organogenesis, cell proliferation, and apoptosis [Citation44]. They can control the radiosensitivity of hepatocytes by regulating the expression of various DDR/DNA repair genes, cell cycle checkpoints, apoptosis, and the TME. The expression level of miRNAs changes significantly after irradiation. As a key component of DDR, the expression of numerous miRNAs stimulate DSB production in an ATM-dependent manner [Citation45].
Table 1. Latest research on RNAs in RILD.
Through the high-throughput deep sequencing of RILD model mice, 48 miRNAs were found to be differentially expressed. These differentially expressed miRNA are involved in regulatory pathways in vivo, such as transforming growth factor-β (TGF-β) signaling, mitogen-activated protein kinase (MAPK) signaling, apoptosis, and the Wnt signaling pathway [Citation46]. Martin et al. demonstrated that IR stimulates DSB production by inhibiting the expression of miR-335 in an ATM-dependent manner, which results in DSBs mainly through the activation of ATM, the phosphorylation of the cAMP response element binding protein, and the down-regulation of the CtIP protein (a key factor in HRR regulating DNA end resection and repair) [Citation47]. In addition, the phosphorylation of p53 by ATM leads to its dissociation from the p53 inhibitor MDM-2 to activate p53, which induces the expression of numerous miRNAs (miR-34s [Citation35], miR-34a, and miR-192 [Citation45,Citation48]. After IR, the specific expression of miR-34a in the liver is increased independently of radiation dose mainly because miR-34a aggravates tissue damage by promoting DNA damage, producing cytokines and cell senescence, or inhibiting cell cycle progression and antioxidant molecule production [Citation49]. miR-34a-5p can also negatively regulate the expression of Krüppel-like factor 4 (KLF4) and induce hepatocyte apoptosis after IR [Citation37]. At the same time, the specific expression of miR-34a in the liver is increased independently of radiation dose, while miR-34a regulates cell cycle arrest and senescence in the G1 phase by inducing p53, inflammation, and apoptosis to promote DNA damage and aggravate tissue damage [Citation49,Citation50]. miR-34a has been found to activate p53 by inhibiting the activation of mitochondrial deacetylase sirtuin 1 (SIRT1) and protecting p53 from acetylation on its K382 residue [Citation51]. Similarly, Li et al. found that the expression of miR-34a and miR-93 is inversely proportional to that of oxidative stress regulation and oxidative stress defense proteins (SIRT1 and Mgst1) and controls the upstream transcription factors (TFs) transcription factor 1 (Sp1) and Nrf2, thus executing a double inhibitory regulatory function at the post-transcriptional level [Citation52]. miR-34a specifically inhibits the activation of p53 by regulating the Myc/Arf/Hdm2 axis [Citation53]. miR-34 can inhibit Mdm4 to enhance the transcriptional activity of p53, reduce the production of the p53 protein, and establish a positive feedback loop while activating p53 [Citation54].
After IR, HSCs can inhibit the signal transduction of lipopolysaccharide (LPS)/Toll-like receptor 4 (TLR4), which inhibits the release of proinflammatory factors (TLR4, IL-1 receptor-related kinase 1 [IRAK1], and tumor necrosis factor receptor-related factor 6 [TRAF6]) and cell proliferation and differentiation and alleviates hepatocyte apoptosis and fibrosis through the NF-κB pathway [Citation38]. RIBE increases the possibility of DDR and secondary cancer after IR. Fu et al. found that overexpressed miR-495 inhibits nitric oxide (NO), a key signal transduction factor of RIBE, and its downstream product, transforming growth factor β1 (TGF-β1), by the regulating the Sp1/endothelial NO synthase (eNOS) pathway, thus attenuating RIBE during RT [Citation39]. miR-21 and miR-34c secreted by the extracellular vesicles (EVs) of irradiated cells can induce the bystander effect in nonirradiated cells [Citation40,Citation41].
In short, the expression level of miRNAs in cells changes significantly after IR. IR induces DDR to regulate the expression of miRNAs by promoting the transcription of miRNA genes or post-transcriptional interaction with miRNAs, thus affecting the occurrence and development of RILD. Even miRNAs can be transferred from irradiated cells to bystander cells by EVs to promote RILD. Although recent progress has been made in the research on the expression and biogenesis of miRNAs in DDR after IR, which is helpful for regulating RILD in the clinic, many unknown problems remain to be solved, and further studies should be done to explore the potential function of miRNAs.
2.2.2. Other RNAs
Cyclic RNAs (circRNAs), as a sponge of miRNAs, competitively absorb miRNAs in the cytoplasm and participate in regulating gene expression [Citation42]. The IR-induced circRNA/miR-146a-5p/RAC1 axis promotes the secretion of proinflammatory cytokines and hepatocyte fibrosis by HSC [Citation42]. CircRNAZNF292 (cZNF292) induces hypoxia and inhibits the SOX9/Wnt/β-catenin axis in a time-dependent manner, thus inhibiting cell proliferation, angiogenesis, and radiation resistance [Citation43]. In one study, Wei et al. found a new class of small RNAs (diRNAs) induced by DSB, which is produced at DNA damage sites and activates HRR by interacting with Dicer or Ago2, leading to chromatin modification or protein complex recruitment to DSB sites to promote repair [Citation55]. The focal formation of the DDR protein requires site-specific DICER and DROSHA-dependent small RNAs, called DNA damage response RNAs (DDRNAs), which act dependently of the MRE11/RAD50/NBS1 (MRN) complex [Citation56]. diRNAs and DDRNAs have the sequences of post-IR damage sites, and as DICER and DROSHA-dependent RNA products, they regulate the response of miRNAs to IR in cells by regulating DDR activation and affecting ATM phosphorylation [Citation45]. Other factors, such as pre-mRNA treatment factor 19 (PRP19), also up-regulate the expression of cyclin D1 by regulating the expression of eIF4E to promote DNA damage repair, thus enhancing the radiation resistance of HCC [Citation57].
2.3. Radiation-induced senescence
Cell senescence is a multistage, multistep dynamic process. When the DNA repair response after IR cannot repair the damage caused by radiation, hepatocytes that have lost their regeneration ability mitigate damage by promoting cell necrosis, senescence, or apoptosis. After senescence, cells secrete a large number of proinflammatory factors, chemokines, growth factors, and proteases, thus forming a proinflammatory microenvironment that affects nearby cells in an autocrine or paracrine manner. These phenotypes are called senescence-associated secretory phenotypes (SASPs) [Citation58]. Senescence-inducing signals (such as DNA damage) induce senescence or cell cycle arrest (blocking cell cycle checkpoints) after sensing DDR and activate DNA repair mechanisms [Citation59] at the G1 phase checkpoints (p53 mMDM2 and CHK2), S phase checkpoints (NBS1, BRCA1, and SMC1), and G2/M phase checkpoints (Brca1, hRad17) involved in IR-induced cell cycle arrest [Citation60]. The accumulation of a large number of senescent cells after IR leads to the loss of liver regeneration and homeostasis, which not only leads to abnormal liver structure and function but also to irreversible liver failure, hepatitis B recurrence, and even liver cancer [Citation61].
Previous studies have shown that p53 induces apoptosis after IR [Citation62]. Le et al. found that in the livers of mice, the loss of p53 after treatment with a sublethal dose of IR has no effect on the long-term expression of senescence markers. Meanwhile, the expression of p53 binding protein 1 and cyclin-dependent kinase inhibitor p16 increases at first then decreased at the focus of DNA injury. Senescence has been proven to be independent of p53 and the intact immune system [Citation63]. However, after IR on the right hepatic lobe of rats, hepatocytes enlarge and the expression levels of senescence-associated β-galactosidase, p16, and p21 increase, proving that radiation could induce hepatocyte senescence [Citation64]. Low-dose radiation can mainly causes cell senescence, whereas high-dose radiation can cause apoptosis accompanied by the reduction in SIRT1 expression [Citation65]. Radiation-induced ROS production and p38 kinase activation promote SIRT1 degradation. The inhibition of ROS and p38/MAPK pathways and the overexpression of SIRT1 can reduce radiation-induced SASPs, while the inhibition of SIRT1 activity can induce senescence [Citation66].
Studies have found that specific antiaging small-molecule inhibitors can selectively kill senescent cells and can be used to improve the organ damage caused by IR. However, this effect has not been studied in RILD but in other tissues; for example, ABT-737 specifically inhibits the apoptosis of lung senescent cells induced by IR through BCL-W and BCL-XL [Citation67]. In mouse bone marrow HSCs and muscle stem cells, ABT-263 can not only inhibit apoptosis and the IR-induced secretion of SASPs through BCL-2 and BCL-xL but also selectively eliminates IR-induced senescent cells [Citation68]. Moreover, in the kidney, ABT-263 can selectively eliminate IR-induced senescent cells, increase renal tubule proliferation, improve function, and reduce fibrosis [Citation69]. Therefore, antiaging small-molecule inhibitors can effectively eliminate senescent cells. Given that senescent cells are the key determinants of tissue regeneration, new strategies and therapeutic targets for alleviating RILD by eliminating senescent cells need further study.
2.4. Radiation-induced mitochondrial DNA damage ()
Mitochondria are the main target of postradiation cytotoxicity. IR inhibits the transfer of the electron transient chain (ETC) in oxidative phosphorylation (OXPHOS) by changing membrane permeability, resulting in excessive superoxide that cannot be removed by SOD and CAT in time. Excessive superoxide induces the generation of a large amount of endogenous ROS [Citation70,Citation71]. IR causes the hydrolyzation of organisms to produce hydroxyl radicals (•OH), leading to a significant increase in the level of exogenous ROS, which induces mitochondrial dysfunction, stimulates mitochondrial ROS (MtROS) production, and induces cell senescence and apoptosis [Citation71]. Studies have shown that IR can destroy the body’s normal redox system. In addition, MtROS interact with oxidative stress through other mitochondria-related pathways and are the main source of endogenous ROS [Citation72,Citation73]. Mitochondrial DNA (mtDNA) is more sensitive to radiation than nuclear DNA and lacks histones and an effective DNA repair system, leading to slow damage repair (the repair rate can be increased by the copy number of DNA; however, this effect may lead to mitochondrial dysfunction) [Citation71]. Damage can accumulate and can be passed on to offspring cells to cause long-term damage and even secondary cancer [Citation64].
Figure 2. Mitochondria are the main targets of radiation-mediated cytotoxic effects. IR inhibits the transmission of the etc during OXPHOS by changing membrane permeability, resulting in the production of excess superoxides. The long-term production of excess superoxides promotes the generation of a large number of endogenous ROS by other pro-oxidases. In living organisms, radiation induces hydrolysis to generate •OH, resulting in a significant increase in the level of exogenous ROS and thereby prompting mitochondrial dysfunction, stimulating MtROS production, and inducing cellular senescence and apoptosis. OXPHOS: oxidative phosphorylation; ETC: electronic transient chain; • OH: hydroxyl radicals; ROS: reactive oxygen species; MtROS: mitochondrial ROS; RONS: reactive oxygen species and nitrogen species; SIRT3: sirtuin 3; SIRT1: sirtuin 1; SOD2: manganese superoxide dismutase; SHP-1: SH2-containing protein tyrosine phosphatase; BCL-2: B-cell lymphoma-2; ATR: recombinant ataxia telangiectasia and Rad3-related protein; ATM: ataxia–telangiectasia mutated proteins; TDG: thymine DNA glycosylase; NBS1: Nijmegen breakage syndrome protein 1; XPA: Xeroderma pigmentosum gene A; c-MYC: cell-MYC.
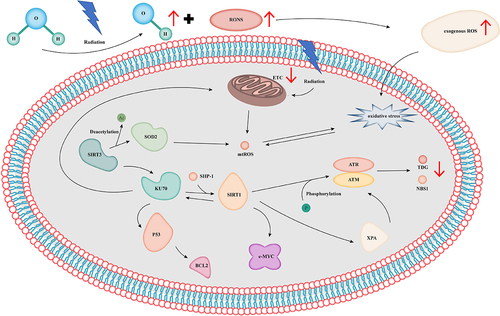
Mitochondrial deacetylase sirtuin 3 (SIRT3), the main protein deacetylase of the mitochondria, is the main regulator of the mitochondrial response to IR and other oxidative stresses. The deacetylation of Ku70 protects cells from stress and regulates mitochondrial oxidative metabolism and antioxidant defense. The knockout of SIRT3 in mice can promote the occurrence and development of IR-induced RILD by affecting the cascade of hydrogen peroxide and hydrogen peroxide signals [Citation74,Citation75]. The superoxide anion (O2 −) and FOXO3a affect RILD through SIRT3 [Citation75–77]. Manganese SOD (Mn-SOD) is an oxidase in eukaryotic mitochondria that realizes antioxidation by dismutating highly active superoxides into water and hydrogen peroxide. Mn-SOD enhances radiosensitivity by inhibiting apoptosis, MtROS production, and LPO [Citation78,Citation79]. SIRT3 changes the enzymatic function of Mn-SOD by regulating its deacetylation [Citation80]. In mouse liver mitochondria, IR induces the deacetylation and increases the enzyme activity of Mn-SOD, indicating that the IR-induced SIRT3/Mn-SOD axis can mitigate the oxidative stress and injury induced by IR [Citation81]. However, the specific mechanism through which SIRT3 acts on RILD by regulating Mn-SOD is unclear.
After IR, PIC regulates the activities of antioxidant enzymes and inhibits oxidative stress, inflammation, and apoptosis to improve mitochondrial function through the AMPK/SIRT1/PGC-1α axis, thereby ameliorating RILD [Citation82]. Resveratrol inhibits the expression of the c-Myc protein by promoting SIRT1 expression and deacetylase activity, thus enhancing the resistance of HepG2 cells to IR-induced micronucleus DNA damage. Xie et al. found that the knockout of SIRT1 in hepatoma cells under hypoxia decreases the expression and acetylation level of the c-Myc protein and enhances the radiosensitivity of hepatoma cells [Citation83,Citation84]. Interestingly, Kang et al. found that epigallocatechin gallate (EGCG) has the opposite effect on mouse AML12 and H22 cells after IR. In mouse hepatocytes, EGCG promotes the expression of Bcl2; inhibits the expression of Bax and Caspase-3 by inhibiting the miR-34a/SIRT1/P53 axis; and attenuates RILD through antioxidation. In mouse hepatoma cells, the activation of the miR-34a/SIRT1/P53 axis can inhibit the expression of Bcl2 and promote the expression of Bax and Caspase-3 to enhance the radiosensitivity of H22 [Citation85]. These two results may be due to differences in the TME.
Regulating the acetylation of SIRT3 and SIRT1 can regulate mitochondrial metabolism and antioxidation, thus alleviating the damage caused by IR-induced oxidative stress. In addition to SIRT3 and SIRT1, other sirtuins regulate the function of mitochondria. For example, SIRT4 and SIRT5 are also involved in the regulation of mitochondrial function and play an indispensable role in metabolic regulation, energy balance, and disease [Citation86].
3. Cellular mechanism of RILD
Liver NPCs, such as KCs, SECs, and HSCs, are well known to respond to IR and release many cytokines after IR [Citation87]. The interaction between hepatocytes and NPCs is a double-edged sword. The inhibition of KCs can reduce radiation-induced SEC apoptosis and protect the liver from radiation-induced damage [Citation88]. The IR-induced apoptosis of SECS and inflammation caused by oxidative stress are considered to be the initiating factors of RILD. Phenotypic changes in liver sinusoidal endothelial cells (LSECs), including the disappearance of LSECs in the window of Disse gap; the formation of capillaries and the basement membrane, which has been proved to be a precursor of fibrosis; and the secretion of fibronectin EIIIA promotes HSC activation [Citation89]. Radiation prompts NPCs to secrete inflammatory cytokines and causes RILD [Citation90]. RILD can be promoted by activating the JNK/c-Jun pathway to increase the phosphorylation of ERK, Akt, and p38 and the secretion of Fas ligands [Citation91].
3.1. Role of SECs in RILD ()
Cheng et al. found that apoptosis occurs mainly in SECs rather than in hepatocytes after IR. In vivo, the systemic infusion of MSC-conditioned medium specifically inhibits SEC apoptosis through Akt and ERK1/2, decreases the secretion and expression of inflammatory factors, and increases the expression of anti-inflammatory factors, which all improve the VOD of rats with IR-induced liver injury [Citation92]. The intravenous injection of bone marrow mesenchymal stem cells (BM-MSCs) into mice can significantly reduce serum transaminase and alleviate radiation-induced injury [Citation93]. BM-MSC injection into rats can improve RILF by inhibiting the transformation of the growth factor-β/Smad signaling pathway [Citation94]. The overexpression of hepatocyte growth factors induces adipose-derived mesenchymal stem cells to down-regulate α-smooth muscle actin and fibronectin to ameliorate RILF [Citation95]. The TIF is a special microenvironment surrounding NPCs that plays an important role in the immune response in IR-induced liver injury [Citation96]. FTY720 alleviates RILD by activating the AKT pathway but has no protective effect on hepatoma cells likely in relation to its gene mutation and the tumor-promoting microenvironment [Citation97].
Figure 3. Morphological changes in postradiation LSECs. Hepatocytes and hepatic sinus-like endothelial cells are spatially separated by the Disse space, a narrow gap between hepatocytes and blood sinus endothelial cells that also contains ECM. LSECs disappear in the window of the Disse gap and capillaries are formed, while secreting fibronectin EIIIA promotes the activation of HSC and forms a basement membrane. KCs are activated and release inflammatory factors.
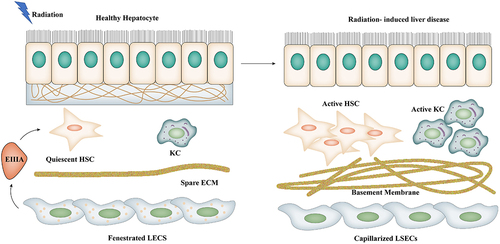
3.2. KCs release TNF-α
KCs are efficient resident macrophages that recognize, ingest, and degrade cell fragments, foreign bodies, or pathogens, thus ensuring the dynamic balance of the liver and eliminating antibodies, fragments, or dead cells [Citation98]. However, KCs are also a major source of proinflammatory cytokines in toxic reactions.
When the body is damaged by external stimuli, such as IR, cells and tissues produce a large amount of ROS and KCs to phagocytize and kill bacteria and other substances and attract numerous inflammatory monocyte-derived macrophages into the liver by producing a variety of inflammatory factors and chemokines [Citation99,Citation100]. Newly infiltrated macrophages adjust their phenotypes in accordance with the signals of the liver microenvironment (such as danger signals, fatty acids, and cell fragment phagocytosis), which can not only promote tissue repair but also aggravate injury; the damaging effect of this phenomenon is mainly reflected by the expression of inflammatory factors, including TNF-α [Citation101,Citation102]. The continuous release of TNF-α and malignant cells may hijack the TNF-dependent survival pathway to gain a selective growth advantage, resulting in the occurrence and development of a variety of diseases [Citation101,Citation103]. IR promotes the secretion of growth factor ligands, for example, TGF-α and TNF-α, by enhancing the response of the intracellular MAPK pathway, thus promoting the bystander effect in cells [Citation31]. The interaction between KCs and hepatocytes has also been confirmed to be involved in the pathogenesis of several toxic liver injuries, including alcoholic liver disease, ischemia/reperfusion injury, and primary graft dysfunction after experimental liver transplantation [Citation104–106]. In animal experiments, the production of ROS after IR is positively correlated with liver injury in mice [Citation107].
Radiation toxicity is related to the excessive production of early inflammatory cytokines. The expression of TNF-α, IL-1, and IL-6 in RT-induced monocytes/macrophages is considered to be the intermediary of the pathogenesis of radiation pneumonia and radiation pulmonary fibrosis [Citation108,Citation109]. Radiation can also induce the expression of IL-1 β, IL-6, and TNF-α in the liver [Citation110]. Christin et al. reported that irradiated hepatocytes cultured in conditioned medium containing increased levels of TNF-α produced by irradiated KC have higher apoptosis rates than normal irradiated hepatocytes [Citation111]. However, later studies found that radiation induces apoptosis in SECs, not hepatocytes. SEC damage may lead to microcirculatory blood flow disturbance and adhesion molecule-dependent leukocyte marginalization and activation and eventually inflict secondary hepatocyte injury on hepatocytes [Citation88,Citation90]. However, regardless of the type of apoptosis induced, the GdCl3-selective inactivation of KCs can reduce the production of radiation-induced cytokines and protect the liver from acute radiation injury [Citation88,Citation90]. Antisense oligodeoxynucleotides can protect the liver from radiation-induced apoptosis by inhibiting the secretion of TNF-α by KCs after IR, resulting in a decrement in the level of tumor necrosis factor receptor 1 [Citation112]. Embryo-derived KCs subsets enhance the IR resistance of hepatocytes by up-regulating the CDKN1A gene encoding the p21-cip1/WAF1 protein [Citation113].
In summary, the activation and release of TNF-α by KCs play a key role in initiating IR-induced hepatocyte injury. However, the specific mechanism of this effect needs further exploration.
3.3. Release of TGF-β by HSCs ()
IR-induced hepatic fibrosis is a prominent feature of RILD, and HSCs are the main effector cells of RILF. IR induces the activation of HSCs, and HSCs are activated and transformed into myofibroblast-like cells. All kinds of fibrosis factors take HSCs as the final target cell. Through the production of collagen and the secretion of a variety of fibrosis-related inflammatory factors, cytokines, and receptors, the synthesis of the extracellular matrix (ECM) is increased, and the synthesis and secretion of tissue inhibitor of metalloproteinase and plasminogen activator inhibitor-1 (also known as SERPINE1) are increased. This effect reduces the degradation of ECM and promotes the accumulation of ECM. At the same time, excessive ECM induces LSECs to form the basement membrane, resulting in liver dysfunction. Cell proliferation and contraction increase intrahepatic sinusoidal pressure, and many factors interact with each other to lead to liver fibrosis and cirrhosis [Citation89,Citation114,Citation115].
Figure 4. After radiation, hepatocytes interact with NPCs, activate the inflammatory response, and participate in the development of RILD. Radiation directly induces various damages in hepatocytes through oxidative stress and inflammatory response and activates LSECs, KCs, and HSCs by secreting inflammatory factors and growth factors, prompting these cells to secrete TNF-α and TGF-β, which play an important role in RILF. TNF α: tumor necrosis factor α; TGF-α: transformational growth factor α; RIBE: radiation-induced bystander effects; ROS: reactive oxygen species; NOS: reactive nitrogen species; DDR: DNA damage response; IL-1β:interleukin 1β; IL-18: Interleukin 18; IL-6: Interleukin 6; EIIA: fibronectin secretion; TLR4: Toll-like receptor 4; HGF: hepatocyte growth factor; TNFR1: tumor necrosis factor receptor 1; RAC1: Ras-associated C3 Botulinum toxin substrate 1; LPO: lipid peroxidation; Hh: Hedgehog.
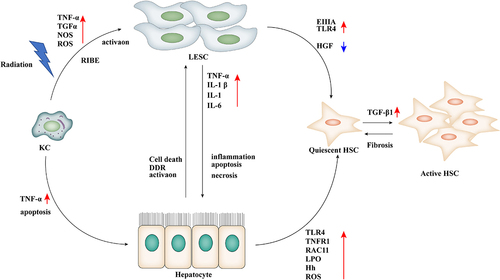
TGF-β is a transforming growth factor that plays an important role in regulating cell proliferation, differentiation, migration, and fibrosis after injury. It is also one of the most important fibrogenic cytokines in hepatic fibrosis given that it regulates ECM proteins in RILD. In SD rats in the early stage of IR, TGF-β1 and TGF-β3 mRNAs are differentially expressed, in which TGF-β1 promotes fibrosis [Citation116]. In RILF model rats with TGF-β1 overexpression, oxidative stress damage is reduced and liver fibrosis is significantly improved, indicating that TGF-β plays an important role in RILD [Citation117–119].
The proteomic analysis of L×2 after irradiation and TGF-β1 treatment revealed that in HSCs, exposure to IR or TGF-β1 increases the expression of α-SMA, collagen 1, and fibronectin [Citation115]. Liver cells cultured in conditioned medium (added to the supernatant of irradiated HSC cells) after IR have higher damage and death rates than normal irradiated hepatocytes. IR induces the activation of HSCs, and the TLR4 signal cascade reaction occurs in activated HSCs, which promotes the secretion of inflammatory cytokines, chemokines, and adhesion molecules into the cell culture medium. The infiltration of inflammatory cells into the damaged microenvironment promotes the occurrence and development of hepatic inflammation and injury and leads to liver fibrosis and even the formation of the tumor-promoting microenvironment to promote the proliferation and migration of HCC cells [Citation38,Citation120,Citation121]. KD, an extract of traditional Chinese herbal medicine, has a protective effect on the liver. In the rat model of RILF, KD alleviates RILF by inhibiting the expression of fibrosis-related proteins by regulating the TGF-β1/Smad/CTGF axis [Citation122]. In a similar study, Sun et al. found that pirfenidone inhibits intestinal fibrosis in rats by inhibiting the TGF-β1/Smad/CTGF axis [Citation123]. SMAD3 can induce the transcription of fibrogenic miRNA and long noncoding RNA and inhibit the transcription of antifibrotic miRNA. Paeoniflorin (C23H28O11) inhibits the progression of RILF by regulating the TGF-β1/Smad signaling pathway in RILF rats [Citation124]. This series of studies demonstrates that the TGF-β 1/Smad signaling pathway plays a key role in the process of IR-induced fibrosis [Citation125]. In terms of drugs, in rats given pioglitazone after exposure to IR, the levels of serum fibrosis markers, such as hyaluronic acid, laminin, and type III procollagen, have decreased. In addition, the expression levels of hepatic fibrosis markers, TGF-β1, and hydroxyproline have reduced. Pioglitazone is suggested to improve RILD in irradiated rats by regulating antifibrosis [Citation126].
Other related protein studies, such as Ras-related C3 botulinum toxin substrate 1 (RAC1), are specifically increased in HSC cells after IR. Cheng et al. demonstrated in vitro that the circRSF1/miR-146a-5p/RAC1 axis can increase cell viability and promote the inflammation and fibrosis of irradiated cells, thus inducing RILD [Citation42]. Wang et al found that the Hedgehog pathway promotes EMT and regulates the repair response in RIDD by inducing the proliferation of progenitor cells and myofibroblast-HSCs with the accumulation of radiation dose [Citation127]. In addition, ROS produced by IR can regulate the fibrosis induced by TGF-β 1 through an atypical SMAD-independent mechanism and can be prompted by the TGF-β-mediated PI3K/Akt signaling pathway [Citation128]. TGF-β1 can aggravate the occurrence and development of RILD by positive feedback [Citation119].
3.4. Treatment of RILD ()
Although the diagnostic criteria for RILD are very perfect, no specific treatment for RILD exists. Clinical data show that the mortality rate of RILD caused by RT is as high as 76.5% [Citation153]. Taking drugs, such as aspartate and ornithine, can improve liver function and reduce radiation-induced damage to hepatocytes [Citation154]. As a commonly used radiation protective drug, amifostine can treat platinum toxicity and IR-induced hematopoietic injury and inflammation, as well as protect normal liver tissue from IR damage [Citation155,Citation156]. Moreover, some studies have found that in the tumor cells of the rat liver, amifostine can protect HCs from radiation damage without affecting the effect of RT [Citation157] but induces corresponding side effects, such as dizziness, nausea, vomiting, and transient hypotension. Melatonin and vitamin D can be used as antioxidants to reduce the incidence of neurotoxicity, thrombocytopenia, and hypoxia during chemotherapy by removing active free radicals [Citation158], inhibiting inflammation, and repairing DSBs [Citation159]. They are expected to become a new adjuvant to inhibit the side effects of RT and improve its therapeutic effect. However, no clinical trials have been carried out to prove their safety and effectiveness. NU5455 is a highly selective oral DNA-PKcs inhibitor that has stronger antitumor activity than the small-molecule inhibitors of DNA repair proteins (ATM, PARP, and ATR) [Citation160]. At the same time, it does not significantly affect acute DNA damage in normal tissues and does not aggravate radiation-induced toxicity. It is mainly used as an adjuvant for RT by improving the radiosensitivity of cells [Citation161].
Table 2. Latest research on antioxidants that relieve RILD.
Symptomatic support therapy include restricting water and sodium intake; using oral diuretics or puncture to treat ascites and improve water – electrolyte balance; installing a transjugular intrahepatic portosystemic shunt to reduce the risk of varicose vein bleeding; taking appropriate amounts of anticoagulants, such as steroids, warfarin, and heparin, to reduce the formation of hepatic vein thrombosis; and taking analgesics to relieve pain [Citation162]. With the continuous development of RT technology in addition to traditional X-ray therapy, the accuracy of RT has increased. At present, specific treatment methods can be selected in accordance with the target site and disease stage. The latest RT technology can enable high-dose radiation to act on the focus while limiting its damage to the surrounding healthy tissue; this effect greatly increases the chance of the long-term survival of patients [Citation163,Citation164]. A growing number of clinical studies are exploring the combination of immunotherapy and RT, which is expected to become a new combined treatment strategy for HCC, and have achieved preliminary results [Citation164].
However, these methods mainly aim to prevent and alleviate symptoms rather than fundamentally cure RILD. Therefore, the molecular pathogenic mechanism of RILD should be further studied to find additional available drugs for the accurate prevention and treatment of patients with RILD and improve their health.
Traditional Chinese medicine has complex chemical structures and biological activities. Studies have found that traditional Chinese medicine can not only protect normal cells and radiosensitize cancer cells, it can also improve drug efficacy, neutralize adverse reactions, reduce oxidative stress, down-regulate inflammatory reactions and apoptosis, promote DNA damage repair, and play an important role in RT [Citation85,Citation165]. Curcumin, a natural diketone compound, exhibits antioxidant, anti-inflammatory, and radioprotective properties by inhibiting the activation of the Wnt/β-catenin and NF-κB pathways and the activation of the transcription of oxidative stress-related genes, such as nucleotide-bound oligomeric domain-like receptor 3 inflammatory bodies, and plays an important role in liver injury caused by various factors [Citation166,Citation167]. Srinivasan et al. found that 6.91 μM curcumin can specifically increase the levels of serum enzymes (such as ALT, AST, and LDH) and nonenzymatic antioxidants (such as SOD, CAD, and GSH) to protect liver function, reduce oxidative stress, and protect the liver from IR damage [Citation129]. In vivo experiments showed that curcumin attenuates oxidative stress through the Nrf2/keap1 pathway [Citation168]. Compounds, for example, curcumin and copper curcumin (1:1) and hemoglobin – curcumin nanoparticles, also have protective effects on radiation-induced liver injury [Citation149,Citation150]. Curcumin can also mitigate RILD in rats by inhibiting the secretion of TNF-α, IL-1, and IL-6 through the NF-κB pathway [Citation130]. Berberine (Ber) has low toxicity and anti-RT characteristics. It is a new type of RT and chemotherapy drug for liver cancer. Ber has been innovatively loaded into folic acid-targeted Janus gold mesoporous silica nanocarriers to enhance the effect of chemotherapy and RT by affecting cell metabolism and changing TIM [Citation131]. Crocin is a water-soluble carotenoid that is mainly composed of Crocin I. It can induce the repair of liver injury by inhibiting the expression of MDA and increasing the level of GSH [Citation132]. The total triterpenes of Ganoderma lucidum can increase the activities of antioxidant enzymes and the level of GSH in the livers and brains of mice after RT and significantly reduce DNA strand breaks [Citation143].
A growing number of Chinese herbal medicine extracts have been found to play an important role in RT. The ethanolic extract of Antrodia cinnamomea can protect hepatocytes by up-regulating the expression of Nrf2 and its downstream oxidoreductase to eliminate ROS [Citation133]. PIC can alleviate RILD by regulating the activity of oxidases, such as SOD, CAT, and GSH-PX [Citation82]. Spatholobus suberectus (JXT) can not only increase the expression of pJAK2/JAK2, pSTAT5a/STAT5a, and Bcl-2 in bone marrow tissue, it can also increase the level of antioxidant enzymes and inhibit radiation-induced ROS [Citation142]. Mangosteen extract inhibits inflammatory signal transduction by down-regulating TNF-α/NF-κ B, and TGF-β 1, thus reducing IR-induced oxidative damage, inflammation, and apoptosis [Citation144]. Coix seedling extract [Citation146], Phoenix dactylifera pit extract [Citation147], and Cicer arietinum extract [Citation145] improve RILD by regulating the oxidation/antioxidation pathway.
Synthetic drugs, for example, diosmin, ameliorate RILD by promoting the expression of PPAR-γ and inhibiting miR-17-5p to activate the Wnt/β-catenin signaling pathway [Citation148]. In the radiation-resistant HCC cell line HepG2-R, Aurora-a reduces RT-induced apoptosis and enhances radiation resistance in HCC by activating the NF-κB signal pathway [Citation169]. Pioglitazone improves RILD by regulating anti-inflammatory and antifibrosis signal pathways [Citation126]. Yang et al. found that minichromosome maintenance protein 3 increases the nuclear transfer of p65 and enhances radiation resistance in HCC by activating the NF-κB signal pathway [Citation170]. The NF-κB signaling pathway and radioresistance are very important in RT-induced liver injury. Cotton flavin alleviates RILD by improving RT-induced oxidative stress [Citation152].
Others compounds and materials, such as lycopene [Citation134], folic acid [Citation136], diallyl disulfide [Citation137], EGCG [Citation22,Citation85], Panax pseudo-ginseng [Citation135], grape seed oil [Citation141], thymoquinone [Citation17], Nigella sativa oil [Citation138], and caffeic acid phenethyl ester [Citation139,Citation140], all inhibit oxidative stress and inflammation and reduce radiation damage.
4. Discussion
In this review, we analyze and summarize the various molecular and cellular mechanisms and clinical treatment of RILD. RILD is the main complication of RT for liver cancer and abdominal malignant tumors [Citation171]. The toxicity of RT to normal tissue is also the main reason limiting its clinical application. The accumulation of toxicity leads to the abnormal structure and function of the liver and even RILF and liver cirrhosis, thus seriously affecting the follow-up treatment and prognosis [Citation8]. Given that RILD is a multistage, multistep dynamic process, it connects a series of responses through a complex cascade response network, in which various RNAs, oxidative stress, inflammation, senescence, fibrosis, and immune responses interact under the regulation of multiple signal pathways. Relieving tissue damage, restoring cell homeostasis, eliminating inflammation, and reducing cytotoxicity are essential for the treatment of RILD. Although current studies have found that many potential therapeutic targets, whether RNAs, antioxidants, or immunosuppressants, play a role by preventing or reducing radiation toxicity, inhibiting inflammation and oxidative stress, and improving radiation sensitivity, most studies lack sufficient clinical data to verify the toxicity and carcinogenicity of these targets to normal tissues. This situation does not provide substantial help to patients. No targeted drugs for the clinical treatment of RILD exist. In the future, a deepened understanding of the molecular and cellular mechanisms of RILD is needed, and additional potential biomarkers of RILD must be found to promote the development of targeted RT, improve the quality of RT, and ultimately provide economical targeted drugs and RT with reduced toxicity and increased effectiveness to patients.
Acknowledgments
Thank you all for helping and guiding me.
Disclosure statement
No potential conflict of interest was reported by the author(s).
Data availability statement
Data sharing does not apply to this article as no new data were created or analyzed in this study.
Additional information
Funding
References
- Feng RM, Zong YN, Cao SM, et al. Current cancer situation in China: good or bad news from the 2018 global cancer statistics? Cancer Commun (Lond). 2019;39:22.
- Sung H, Ferlay J, Siegel RL, et al. Global cancer statistics 2020: gLOBOCAN estimates of incidence and mortality worldwide for 36 cancers in 185 Countries. CA Cancer J Clin. 2021;71(3):209–249.
- Fan X, Shan S, Wu P, et al. Irradiated and CCl 4 -treated bone marrow-derived liver macrophages exhibit different gene expression patterns and phenotypes. Scand J Immunol. 2020;92(5):e12916.
- Xiao Z, Shen J, Zhang L, et al. Therapeutic targeting of noncoding RNAs in hepatocellular carcinoma: recent progress and future prospects. Oncol Lett. 2018;15:3395–3402.
- Jun BG, Kim YD, Cheon GJ, et al. Clinical significance of radiation-induced liver disease after stereotactic body radiation therapy for hepatocellular carcinoma. Korean J Intern Med. 2018;33:1093–1102.
- Ingold JA, Reed GB, Kaplan HS, et al. Radiation hepatitis. Am J Roentgenol Radium Ther Nucl Med. 1965;93:200–208.
- Treiber T, Treiber N, Meister G. Regulation of microRNA biogenesis and its crosstalk with other cellular pathways. Nat Rev Mol Cell Biol. 2019;20(1):5–20.
- Kim J, Jung Y. Radiation-induced liver disease: current understanding and future perspectives. Exp Mol Med. 2017;49(7):e359.
- Munoz-Schuffenegger P, Ng S, Dawson LA. Radiation-induced liver toxicity. Semin Radiat Oncol. 2017;27(4):350–357.
- Benson R, Madan R, Kilambi R, et al. Radiation induced liver disease: a clinical update. J Egypt Natl Canc Inst. 2016;28(1):7–11.
- Voncken FEM, Aleman BMP, van Dieren JM, et al. Strahlungsinduzierte Leberschäden ähneln Lebermetastasen im FDG-PET-CT nach Radiochemotherapie beim Ösophaguskarzinom. Strahlentherapie Und Onkologie : Organ der Deutschen Rontgengesellschaft [Et Al]. 2018;194(2):156–163.
- Tsuchida T, Friedman SL. Mechanisms of hepatic stellate cell activation. Nat Rev Gastroenterol Hepatol. 2017;14(7):397–411.
- Du S, Chen G, Yuan B, et al. DNA sensing and associated type 1 interferon signaling contributes to progression of radiation-induced liver injury. Cell Mol Immunol. 2021;18(7):1718–1728.
- Cao Y, Yin Y, Wang X, et al. Sublethal irradiation promotes the metastatic potential of hepatocellular carcinoma cells. Cancer Sci. 2021;112(1):265–274.
- Chatzipapas KP, Papadimitroulas P, Emfietzoglou D, et al. Ionizing Radiation and Complex DNA Damage: Quantifying the Radiobiological Damage Using Monte Carlo Simulations. Cancers (Basel). 2020;12(4):799.
- Taysi S, Tascan AS, Ugur MG, et al. Radicals, Oxidative/Nitrosative Stress and Preeclampsia. Mini Rev Med Chem. 2019;19(3):178–193.
- Taysi S, Algburi FS, Mohammed Z, et al. Thymoquinone: a review on its pharmacological importance, and its association with oxidative stress, COVID-19, and radiotherapy. Mini Rev Med Chem. 2022;22(14). DOI:10.2174/1389557522666220104151225
- Qin H, Zhang H, Zhang S, et al. Protective effect of sirt1 against radiation-induced damage. Radiat Res. 2021;196:647–657.
- Park HS, You GE, Yang KH, et al. Role of AKT and ERK pathways in controlling sensitivity to ionizing radiation and adaptive response induced by low-dose radiation in human immune cells. Eur J Cell Biol. 2015;94(12):653–660.
- Shedid SM, Abdel-Magied N, Saada HN. Role of betaine in liver injury induced by the exposure to ionizing radiation. Environ Toxicol. 2019;34:123–130.
- Ali OSM, Amin NE, Abdel Fattah SM, et al. Ameliorative effect of kefir against γ-irradiation induced liver injury in male rats: impact on oxidative stress and inflammation. Environ Sci Pollut Res Int. 2020;27(28):35161–35173.
- Yi J, Chen C, Liu X, et al. Radioprotection of EGCG based on immunoregulatory effect and antioxidant activity against (60)Coγ radiation-induced injury in mice. Food Chem Toxicol. 2020;135:111051.
- Szatmári T, Kis D, Bogdándi EN, et al. Extracellular vesicles mediate radiation-induced systemic bystander signals in the bone marrow and spleen. Front Immunol. 2017;8:347.
- Farhood B, Khodamoradi E, Hoseini-Ghahfarokhi M, et al. TGF-β in radiotherapy: mechanisms of tumor resistance and normal tissues injury. Pharmacol Res. 2020;155:104745.
- Her J, Bunting SF. How cells ensure correct repair of DNA double-strand breaks. J Biol Chem. 2018;293(27):10502–10511.
- Saldivar JC, Cortez D, Cimprich KA. The essential kinase ATR: ensuring faithful duplication of a challenging genome. Nat Rev Mol Cell Biol. 2017;18(10):622–636.
- Obama K, Satoh S, Hamamoto R, et al. Enhanced expression of RAD51 associating protein-1 is involved in the growth of intrahepatic cholangiocarcinoma cells. Clin Cancer Res. 2008;14:1333–1339.
- Lee ES, Won YJ, Kim BC, et al. Low-dose irradiation promotes Rad51 expression by down-regulating miR-193b-3p in hepatocytes. Sci Rep. 2016;6:25723.
- Tsai CL, Liu WL, Hsu FM, et al. Targeting histone deacetylase 4/ubiquitin-conjugating enzyme 9 impairs DNA repair for radiosensitization of hepatocellular carcinoma cells in mice. Hepatology. 2018;67:586–599.
- Sage E, Shikazono N. Radiation-induced clustered DNA lesions: repair and mutagenesis. Free Radic Biol Med. 2017;107:125–135.
- Dent P, Yacoub A, Fisher PB, et al. MAPK pathways in radiation responses. Oncogene. 2003;22:5885–5896.
- Khalil A, Morgan RN, Adams BR, et al. ATM-dependent ERK signaling via AKT in response to DNA double-strand breaks. Cell Cycle (Georgetown, Tex). 2011;10:481–491.
- Nagane M, Kuppusamy ML, An J, et al. Ataxia-Telangiectasia Mutated (ATM) kinase regulates eNOS expression and modulates radiosensitivity in endothelial cells exposed to ionizing radiation. Radiat Res. 2018;189:519–528.
- Kim J, Wong PK. Loss of ATM impairs proliferation of neural stem cells through oxidative stress-mediated p38 MAPK signaling. Stem cells.2009; Vol. 27, pp. 1987–1998.
- He L, He X, Lim LP, et al. A microRNA component of the p53 tumour suppressor network. Nature. 2007;447:1130–1134.
- Wu ZF, Zhang JY, Shen XY, et al. A mouse radiation-induced liver disease model for stereotactic body radiation therapy validated in patients with hepatocellular carcinoma. Med Phys. 2016;43:4349.
- Chen Q, Li L, Tu Y, et al. MiR-34a regulates apoptosis in liver cells by targeting the KLF4 gene. Cell Mol Biol Lett. 2014;19(1):52–64. DOI:10.2478/s11658-013-0115-y
- Chen Y, Wu Z, Yuan B, et al. MicroRNA-146a-5p attenuates irradiation-induced and LPS-induced hepatic stellate cell activation and hepatocyte apoptosis through inhibition of TLR4 pathway. Cell Death Amp Dis. 2018;9:22.
- Fu J, Jiang M, Zhang M, et al. MiR-495 functions as an adjuvant to radiation therapy by reducing the radiation-induced bystander effect. Acta Biochim Biophys Sin (Shanghai). 2016;48:1026–1033.
- Xu S, Wang J, Ding N, et al. Exosome-mediated microRNA transfer plays a role in radiation-induced bystander effect. RNA Biol. 2015;12:1355–1363.
- Rastogi S, Hwang A, Chan J, et al. Extracellular vesicles transfer nuclear Abl-dependent and radiation-induced miR-34c into unirradiated cells to cause bystander effects. Mol Biol Cell. 2018;29:2228–2242.
- Chen Y, Yuan B, Chen G, et al. Circular RNA RSF1 promotes inflammatory and fibrotic phenotypes of irradiated hepatic stellate cell by modulating miR-146a-5p. J Cell Physiol. 2020;235:8270–8282.
- Yang W, Liu Y, Gao R, et al. Knockdown of cZNF292 suppressed hypoxic human hepatoma SMMC7721 cell proliferation, vasculogenic mimicry, and radioresistance. Cell Signal. 2019;60:122–135.
- Han Y, Zhang J, Huang S, et al. MicroRNA-223-3p inhibits vascular calcification and the osteogenic switch of vascular smooth muscle cells. J Biol Chem. 2021;296:100483.
- Mao A, Liu Y, Zhang H, et al. microRNA expression and biogenesis in cellular response to ionizing radiation. DNA Cell Biol. 2014;33:667–679.
- Lu J, Chen C, Hao L, et al. MiRNA expression profile of ionizing radiation-induced liver injury in mouse using deep sequencing. Cell Biol Int. 2016;40:873–886.
- Martin NT, Nakamura K, Davies R, et al. ATM-dependent MiR-335 targets CtIP and modulates the DNA damage response. PLoS Genet. 2013;9:e1003505.
- Georges SA, Biery MC, Kim SY, et al. Coordinated regulation of cell cycle transcripts by p53-Inducible microRnas, miR-192 and miR-215. Cancer Res. 2008;68(24):10105–10112. DOI:10.1158/0008-5472.CAN-08-1846
- Lacombe J, Zenhausern F. Emergence of miR-34a in radiation therapy. Crit Rev Oncol Hematol. 2017;109:69–78.
- Tarasov V, Jung P, Verdoodt B, et al. Differential regulation of microRnas by p53 revealed by massively parallel sequencing: miR-34a is a p53 target that induces apoptosis and G1-arrest. Cell Cycle (Georgetown, Tex). 2007;6:1586–1593.
- Chen F, Hu SJ. Effect of microRNA-34a in cell cycle, differentiation, and apoptosis: a review. J Biochem Mol Toxicol. 2012;26:79–86.
- Li N, Muthusamy S, Liang R, et al. Increased expression of miR-34a and miR-93 in rat liver during aging, and their impact on the expression of Mgst1 and Sirt1. Mech Ageing Dev. 2011;132:75–85.
- Sotillo E, Laver T, Mellert H, et al. Myc overexpression brings out unexpected antiapoptotic effects of miR-34a. Oncogene. 2011;30:2587–2594.
- Okada N, Lin CP, Ribeiro MC, et al. A positive feedback between p53 and miR-34 miRnas mediates tumor suppression. Genes Dev. 2014;28(5):438–450. DOI:10.1101/gad.233585.113
- Wei W, Ba Z, Gao M, et al. A role for small RNAs in DNA double-strand break repair. Cell. 2012;149:101–112.
- Francia S, Michelini F, Saxena A, et al. Site-specific DICER and DROSHA RNA products control the DNA-damage response. Nature. 2012;488:231–235.
- Yu XN, Zhang GC, Liu HN, et al. Pre-mRNA processing factor 19 functions in DNA damage repair and radioresistance by modulating cyclin D1 in hepatocellular carcinoma. Mol Ther Nucl Acids. 2022;27:390–403.
- Zhu W, Zhang X, Yu M, et al. Radiation-induced liver injury and hepatocyte senescence. Cell Death Discov. 2021;7:244.
- Fletcher-Sananikone E, Kanji S, Tomimatsu N, et al. Elimination of radiation-induced senescence in the brain tumor microenvironment attenuates glioblastoma recurrence. Cancer Res. 2021;81(23):5935–5947. DOI:10.1158/0008-5472.CAN-21-0752
- Bakkenist CJ, Kastan MB. DNA damage activates ATM through intermolecular autophosphorylation and dimer dissociation. Nature. 2003;421:499–506.
- Toesca DAS, Ibragimov B, Koong AJ, et al. Strategies for prediction and mitigation of radiation-induced liver toxicity. J Radiat Res. 2018;59:i40–9.
- Du SS, Zeng ZC, Tang ZY, et al. Regenerative capacity of normal and irradiated liver following partial hepatectomy in rats. Int J Radiat Biol. 2009;85(12):1114–1125.
- Le ON, Rodier F, Fontaine F, et al. Ionizing radiation-induced long-term expression of senescence markers in mice is independent of p53 and immune status. Aging Cell. 2010;9(3):398–409.
- Serra MP, Marongiu F, Sini M, et al. Hepatocyte senescence induced by radiation and partial hepatectomy in rat liver. Int J Radiat Biol. 2014;90:876–883.
- Panganiban RA, Mungunsukh O, Day RM. X-irradiation induces ER stress, apoptosis, and senescence in pulmonary artery endothelial cells. Int J Radiat Biol. 2013;89:656–667.
- Marampon F, Gravina GL, Festuccia C, et al. Vitamin D protects endothelial cells from irradiation-induced senescence and apoptosis by modulating MAPK/SirT1 axis. J Endocrinol Invest. 2016;39:411–422.
- Yosef R, Pilpel N, Tokarsky-Amiel R, et al. Directed elimination of senescent cells by inhibition of BCL-W and BCL-XL. Nat Commun. 2016;7:11190.
- Chang J, Wang Y, Shao L, et al. Clearance of senescent cells by ABT263 rejuvenates aged hematopoietic stem cells in mice. Nat Med. 2016;22:78–83.
- Mylonas KJ, O’Sullivan ED, Humphries D, et al. Cellular senescence inhibits renal regeneration after injury in mice, with senolytic treatment promoting repair. Sci Transl Med. 2021;13(594). DOI:10.1126/scitranslmed.abb0203.
- Smeitink JA, Zeviani M, Turnbull DM, et al. Mitochondrial medicine: a metabolic perspective on the pathology of oxidative phosphorylation disorders. Cell Metab. 2006;3:9–13.
- Kam WW, Banati RB. Effects of ionizing radiation on mitochondria. Free Radic Biol Med. 2013;65:607–619.
- Kawamura K, Qi F, Kobayashi J. Potential relationship between the biological effects of low-dose irradiation and mitochondrial ROS production. J Radiat Res. 2018;59:ii91–7.
- Schulz E, Wenzel P, Münzel T, et al. Mitochondrial redox signaling: interaction of mitochondrial reactive oxygen species with other sources of oxidative stress. Antioxid Redox Signaling. 2014;20:308–324.
- ] LoBianco FV, Krager KJ, Carter GS, Alam S, Yuan Y, Lavoie EG, Dranoff JA, Aykin-Burns N. The Role of Sirtuin 3 in Radiation-Induced Long-Term Persistent Liver Injury. Antioxidants. 9. Basel, Switzerland; 2020.
- Coleman MC, Olivier AK, Jacobus JA, et al. Superoxide mediates acute liver injury in irradiated mice lacking sirtuin 3. Antioxid Redox Signaling. 2014;20:1423–1435.
- Sundaresan NR, Samant SA, Pillai VB, et al. SIRT3 is a stress-responsive deacetylase in cardiomyocytes that protects cells from stress-mediated cell death by deacetylation of Ku70. Mol Cell Biol. 2008;28(20):6384–6401.
- Jacobs KM, Pennington JD, Bisht KS, et al. SIRT3 interacts with the daf-16 homolog FOXO3a in the mitochondria, as well as increases FOXO3a dependent gene expression. Int J Biol Sci. 2008;4:291–299.
- Motoori S, Majima HJ, Ebara M, et al. Overexpression of mitochondrial manganese superoxide dismutase protects against radiation-induced cell death in the human hepatocellular carcinoma cell line HLE. Cancer Res. 2001;61:5382–5388.
- Indo HP, Inanami O, Koumura T, et al. Roles of mitochondria-generated reactive oxygen species on X-ray-induced apoptosis in a human hepatocellular carcinoma cell line, HLE. Free Radic Res. 2012;46(8):1029–1043. DOI:10.3109/10715762.2012.698012
- Tao R, Vassilopoulos A, Parisiadou L, et al. Regulation of MnSOD enzymatic activity by Sirt3 connects the mitochondrial acetylome signaling networks to aging and carcinogenesis. Antioxid Redox Signaling. 2014;20:1646–1654.
- Tao R, Coleman MC, Pennington JD, et al. Sirt3-mediated deacetylation of evolutionarily conserved lysine 122 regulates MnSOD activity in response to stress. Mol Cell. 2010;40:893–904.
- Mahmoud Moustafa E, Rashed ER, Rashed RR, et al. Piceatannol promotes hepatic and renal AMPK/SIRT1/PGC-1α mitochondrial pathway in rats exposed to reserpine or gamma-radiation. Int J Immunopathol Pharmacol. 2021;35:20587384211016194.
- Xie Y, Zhang J, Xu Y, et al. SirT1 confers hypoxia-induced radioresistance via the modulation of c-Myc stabilization on hepatoma cells. J Radiat Res. 2012;53:44–50.
- Xie Y, Zhang J, Ye S, et al. SirT1 regulates radiosensitivity of hepatoma cells differently under normoxic and hypoxic conditions. Cancer Sci. 2012;103:1238–1244.
- Kang Q, Zhang X, Cao N, et al. EGCG enhances cancer cells sensitivity under (60)Coγ radiation based on miR-34a/Sirt1/p53. Food Chem Toxicol. 2019;133:110807.
- Parihar P, Solanki I, Mansuri ML, et al. Mitochondrial sirtuins: emerging roles in metabolic regulations, energy homeostasis and diseases. Exp Gerontol. 2015;61:130–141.
- Zhou LY, Wang ZM, Gao YB, et al. Stimulation of hepatoma cell invasiveness and metastatic potential by proteins secreted from irradiated nonparenchymal cells. Int J Radiat Oncol Biol Phys. 2012;84:822–828.
- Chen YX, Zeng ZC, Sun J, et al. Radioprotective effect of kupffer cell depletion on hepatic sinusoidal endothelial cells. Radiat Res. 2015;183:563–570.
- Natarajan V, Harris EN, Kidambi S. Secs (Sinusoidal Endothelial Cells), Liver Microenvironment, and Fibrosis. Biomed Res Int. 2017;2017:4097205.
- Du SS, Qiang M, Zeng ZC, et al. Inactivation of kupffer cells by gadolinium chloride protects murine liver from radiation-induced apoptosis. Int J Radiat Oncol Biol Phys. 2010;76:1225–1234.
- Dong Y, Shen X, He M, et al. Activation of the JNK-c-Jun pathway in response to irradiation facilitates Fas ligand secretion in hepatoma cells and increases hepatocyte injury. J Exp Clin Cancer Res. 2016;35:114.
- Chen YX, Zeng ZC, Sun J, et al. Mesenchymal stem cell-conditioned medium prevents radiation-induced liver injury by inhibiting inflammation and protecting sinusoidal endothelial cells. J Radiat Res. 2015;56:700–708.
- Mouiseddine M, François S, Souidi M, et al. Intravenous human mesenchymal stem cells transplantation in NOD/SCID mice preserve liver integrity of irradiation damage. Methods Mol Biol. 2012;826:179–188.
- Shao CH, Chen SL, Dong TF, et al. Transplantation of bone marrow-derived mesenchymal stem cells after regional hepatic irradiation ameliorates thioacetamide-induced liver fibrosis in rats. J Surg Res. 2014;186:408–416.
- Zhang J, Zhou S, Zhou Y, et al. Hepatocyte growth factor gene-modified adipose-derived mesenchymal stem cells ameliorate radiation induced liver damage in a rat model. PLoS One. 2014;9:e114670.
- Zhi-Feng W, Le-Yuan Z, Xiao-Hui Z, et al. TLR4-dependent immune response promotes radiation-induced liver disease by changing the liver tissue interstitial microenvironment during liver cancer radiotherapy. Radiat Res. 2014;182:674–682.
- Zhang SM, Chen YX, Sun J, et al. FTY720, a sphingosine-1-phosphate (S1P) receptor modulator, protects sinusoid endothelial cells from radiation injury in vitro. Hepatol Int. 2015;9:149–154.
- Tacke F. Targeting hepatic macrophages to treat liver diseases. J Hepatol. 2017;66:1300–1312.
- Mossanen JC, Krenkel O, Ergen C, et al. Chemokine (C-C motif) receptor 2-positive monocytes aggravate the early phase of acetaminophen-induced acute liver injury. Hepatology. 2016;64:1667–1682.
- Zigmond E, Samia-Grinberg S, Pasmanik-Chor M, et al. Infiltrating monocyte-derived macrophages and resident kupffer cells display different ontogeny and functions in acute liver injury. J Immunol. 2014;193:344–353. (Baltimore, Md : 1950).
- Yuan D, Huang S, Berger E, et al. Kupffer Cell-Derived Tnf Triggers Cholangiocellular Tumorigenesis through JNK due to Chronic Mitochondrial Dysfunction and ROS. Cancer Cell. 2017;31:771–89.e6.
- Su L, Li N, Tang H, et al. Kupffer cell-derived TNF-α promotes hepatocytes to produce CXCL1 and mobilize neutrophils in response to necrotic cells. Cell Death Amp Dis. 2018;9:323.
- Nakagawa H, Umemura A, Taniguchi K, et al. ER stress cooperates with hypernutrition to trigger TNF-dependent spontaneous HCC development. Cancer Cell. 2014;26:331–343.
- Slevin E, Baiocchi L, Wu N, et al. Kupffer Cells: inflammation Pathways and Cell-Cell Interactions in Alcohol-Associated Liver Disease. Am J Pathol. 2020;190:2185–2193.
- Li JY, Gu X, Zhang WH, et al. GdCl3 abates hepatic ischemia-reperfusion injury by inhibiting apoptosis in rats. Hepatobiliary Pancreatic Dis Int. 2009;8:518–523.
- Rentsch M, Puellmann K, Sirek S, et al. Benefit of Kupffer cell modulation with glycine versus Kupffer cell depletion after liver transplantation in the rat: effects on postischemic reperfusion injury, apoptotic cell death graft regeneration and survival. Transpl Int. 2005;18:1079–1089.
- An JH, Kim J, Seong J. Redox signaling by ionizing radiation in mouse liver. Ann N Y Acad Sci. 2004;1030:86–94.
- Fedorocko P, Egyed A, Vacek A. Irradiation induces increased production of haemopoietic and proinflammatory cytokines in the mouse lung. Int J Radiat Biol. 2002;78:305–313.
- Linard C, Marquette C, Mathieu J, et al. Acute induction of inflammatory cytokine expression after gamma-irradiation in the rat: effect of an NF-kappaB inhibitor. Int J Radiat Oncol Biol Phys. 2004;58:427–434.
- Christiansen H, Sheikh N, Saile B, et al. X-Irradiation in rat liver: consequent upregulation of hepcidin and downregulation of hemojuvelin and ferroportin-1 gene expression. Radiology. 2007;242:189–197.
- Christiansen H, Saile B, Neubauer-Saile K, et al. Irradiation leads to susceptibility of hepatocytes to TNF-alpha mediated apoptosis. Radiother Oncol. 2004;72:291–296.
- Huang XW, Yang J, Dragovic AF, et al. Antisense oligonucleotide inhibition of tumor necrosis factor receptor 1 protects the liver from radiation-induced apoptosis. Clin Cancer Res. 2006;12:2849–2855.
- Soysa R, Lampert S, Yuen S, et al. Fetal origin confers radioresistance on liver macrophages via p21(cip1/WAF1). J Hepatol. 2019;71(3):553–562.
- Wei J, Feng L, Li Z, et al. MicroRNA-21 activates hepatic stellate cells via PTEN/Akt signaling. Biomedicine & Pharmacotherapy = Biomedecine & Pharmacotherapie. 2013;67:387–392.
- Yuan B, Chen Y, Wu Z, et al. Proteomic Profiling of Human Hepatic Stellate Cell Line LX2 Responses to Irradiation and TGF-β1. J Proteome Res. 2019;18:508–521.
- Seong J, Kim SH, Chung EJ, et al. Early alteration in TGF-beta mRNA expression in irradiated rat liver. Int J Radiat Oncol Biol Phys. 2000;46:639–643. physics.
- Meng XM, Nikolic-Paterson DJ, Lan HY. TGF-β: the master regulator of fibrosis. Nat Rev Nephrol. 2016;12:325–338.
- Wu Y, Wang W, Peng XM, et al. Rapamycin Upregulates Connective Tissue Growth Factor Expression in Hepatic Progenitor Cells Through TGF-β-Smad2 Dependent Signaling. Front Pharmacol. 2018;9:877.
- Du SS, Qiang M, Zeng ZC, et al. Radiation-induced liver fibrosis is mitigated by gene therapy inhibiting transforming growth factor-β signaling in the rat. Int J Radiat Oncol Biol Phys. 2010;78:1513–1523.
- Kim SY, Jeong JM, Kim SJ, et al. Pro-inflammatory hepatic macrophages generate ROS through NADPH oxidase 2 via endocytosis of monomeric TLR4-MD2 complex. Nat Commun. 2017;8:2247.
- Shen X, Zhao J, Wang Q, et al. The Invasive Potential of Hepatoma Cells Induced by Radiotherapy is Related to the Activation of Hepatic Stellate Cells and Could be Inhibited by EGCG Through the TLR4 Signaling Pathway. Radiat Res. 2022;197:365–375.
- Nie X, Yu Q, Li L, et al. Kinsenoside Protects Against Radiation-Induced Liver Fibrosis via Downregulating Connective Tissue Growth Factor Through TGF-β1 Signaling. Front Pharmacol. 2022;13:808576.
- Sun YW, Zhang YY, Ke XJ, et al. Pirfenidone prevents radiation-induced intestinal fibrosis in rats by inhibiting fibroblast proliferation and differentiation and suppressing the TGF-β1/Smad/CTGF signaling pathway. Eur J Pharmacol. 2018;822:199–206.
- Hu Z, Qin F, Gao S, et al. Paeoniflorin exerts protective effect on radiation-induced hepatic fibrosis in rats via TGF-β1/Smads signaling pathway. Am J Transl Res. 2018;10:1012–1021.
- Wang B, Wei J, Meng L, et al. Advances in pathogenic mechanisms and management of radiation-induced fibrosis. Biomedicine & Pharmacotherapy = Biomedecine & Pharmacotherapie. 2020;121:109560.
- Radwan RR, Hasan HF. Pioglitazone ameliorates hepatic damage in irradiated rats via regulating anti-inflammatory and antifibrogenic signalling pathways. Free Radic Res. 2019;53:748–757.
- Wang S, Lee Y, Kim J, et al. Potential role of Hedgehog pathway in liver response to radiation. PLoS One. 2013;8:e74141.
- Xiao L, Zhang H, Yang X, et al. Role of phosphatidylinositol 3-kinase signaling pathway in radiation-induced liver injury. Kaohsiung J Med Sci. 2020;36:990–997.
- Srinivasan M, Sudheer AR, Rajasekaran KN, et al. Effect of curcumin analog on gamma-radiation-induced cellular changes in primary culture of isolated rat hepatocytes in vitro. Chem Biol Interact. 2008;176:1–8.
- Li W, Jiang L, Lu X, et al. Curcumin protects radiation-induced liver damage in rats through the NF-κB signaling pathway. BMC Complement Med Ther. 2021;21:10.
- Li XD, Wang Z, Wang XR, et al. Berberine-loaded Janus gold mesoporous silica nanocarriers for chemo/radio/photothermal therapy of liver cancer and radiation-induced injury inhibition. Int J Nanomedicine. 2019;14:3967–3982.
- Bakshi HA, Zoubi MSA, Hakkim FL, et al. Dietary Crocin is Protective in Pancreatic Cancer while Reducing Radiation-Induced Hepatic Oxidative Damage. Nutrients. 2020;12:1901.
- Kuo TH, Kuo YH, Cho CY, et al. Protective Effect of Antrodia cinnamomea Extract against Irradiation-Induced Acute Hepatitis. Int J Mol Sci. 2019;20(4):846.
- Srinivasan M, Sudheer AR, Pillai KR, et al. Lycopene as a natural protector against gamma-radiation induced DNA damage, lipid peroxidation and antioxidant status in primary culture of isolated rat hepatocytes in vitro. Biochim Biophys Acta. 2007;1770:659–665.
- Kim HG, Jang SS, Lee JS, et al. Panax ginseng Meyer prevents radiation-induced liver injury via modulation of oxidative stress and apoptosis. J Ginseng Res. 2017;41:159–168.
- Srinivasan M, Sudheer AR, Pillai KR, et al. Influence of ferulic acid on gamma-radiation induced DNA damage, lipid peroxidation and antioxidant status in primary culture of isolated rat hepatocytes. Toxicology. 2006;228:249–258.
- Nakajima T, Vares G, Ninomiya Y, et al. Diallyl disulfide mitigates DNA damage and spleen tissue effects after irradiation. Med Sci Monit. 2019;25:8920–8927.
- Cikman O, Ozkan A, Aras AB, et al. Radioprotective effects of Nigella sativa oil against oxidative stress in liver tissue of rats exposed to total head irradiation. J Invest Surg. 2014;27:262–266.
- Cikman O, Taysi S, Gulsen MT, et al. The radio-protective effects of caffeic acid phenethyl ester and thymoquinone in rats exposed to total head irradiation. Wien Klin Wochenschr. 2015;127:103–108.
- Cikman O, Taysi S, Gulsen MT, et al. The radioprotective effects of caffeic acid phenethyl ester and thymoquinone on oxidative and nitrosative stress in liver tissue of rats exposed to total head irradiation. West Indian Med J. 2015;65:1–7.
- Ismail AF, Salem AA, Eassawy MM. Hepatoprotective effect of grape seed oil against carbon tetrachloride induced oxidative stress in liver of γ-irradiated rat. J Photochem Photobiol, B. 2016;160:1–10.
- Dong XZ, Wang YN, Tan X, et al. Protective effect of JXT ethanol extract on radiation-induced hematopoietic alteration and oxidative stress in the liver. Oxid Med Cell Longev. 2018;2018:9017835.
- Smina TP, Joseph J, Janardhanan KK. Ganoderma lucidum total triterpenes prevent γ-radiation induced oxidative stress in Swiss albino mice in vivo. Redox Rep. 2016;21:254–261.
- Hassan AA, Moustafa EM, El-Khashab IH, et al. Mangosteen hinders gamma radiation-mediated oxidative stress and liver injury by down-regulating TNF-α/NF-κB and pro-fibrotic factor TGF-β1 inducing inflammatory signaling. Dose Response. 2021;19:15593258211025190.
- Sayed AA, Abbas OA, Saad MA, et al. Cicer arietinum extract ameliorate γ-irradiation disorders via modulation of oxidative/antioxidative pathway. J Photochem Photobiol, B. 2018;183:46–56.
- Li X, Yi J, Zhu J, et al. Protective effect of coix seed seedling extract on (60) Co-γ radiation-induced oxidative stress in mice. J Food Sci. 2022;87:438–449.
- Abdel-Magied N, Ahmed AG, Abo Zid N. Possible ameliorative effect of aqueous extract of date (Phoenix dactylifera) pits in rats exposed to gamma radiation. Int J Radiat Biol. 2018;94:815–824.
- Hasan HF, Abdel-Rafei MK, Galal SM. Diosmin attenuates radiation-induced hepatic fibrosis by boosting PPAR-γ expression and hampering miR-17-5p-activated canonical Wnt-β-catenin signaling. Biochem Cell Biol. 2017;95:400–414.
- Koiram PR, Veerapur VP, Kunwar A, et al. Effect of curcumin and curcumin copper complex (1:1) on radiation-induced changes of anti-oxidant enzymes levels in the livers of Swiss albino mice. J Radiat Res. 2007;48:241–245.
- Gao R, Gu Y, Yang Y, et al. Robust radiosensitization of hemoglobin-curcumin nanoparticles suppresses hypoxic hepatocellular carcinoma. J Nanobiotechnology. 2022;20:115.
- Vorotnikova E, Rosenthal RA, Tries M, et al. Novel synthetic SOD/catalase mimetics can mitigate capillary endothelial cell apoptosis caused by ionizing radiation. Radiat Res. 2010;173:748–759.
- Khan A, Manna K, Das DK, et al. Gossypetin ameliorates ionizing radiation-induced oxidative stress in mice liver–a molecular approach. Free Radic Res. 2015;49:1173–1186.
- Liang SX, Zhu XD, Xu ZY, et al. Radiation-induced liver disease in three-dimensional conformal radiation therapy for primary liver carcinoma: the risk factors and hepatic radiation tolerance. Int J Radiat Oncol Biol Phys. 2006;65:426–434.
- Mahpour NY, Pioppo-Phelan L, Reja M, et al. Pharmacologic management of hepatic encephalopathy. Clin Liver Dis. 2020;24:231–242.
- Yu X, Li M, Zhu L, et al. Amifostine-loaded armored dissolving microneedles for long-term prevention of ionizing radiation-induced injury. Acta Biomater. 2020;112:87–100.
- Koukourakis MI, Giatromanolaki A, Fylaktakidou K, et al. Amifostine protects mouse liver against radiation-induced autophagy blockage. Anticancer Res. 2018;38:227–238.
- Symon Z, Levi M, Ensminger WD, et al. Selective radioprotection of hepatocytes by systemic and portal vein infusions of amifostine in a rat liver tumor model. Int J Radiat Oncol Biol Phys. 2001;50:473–478.
- Nuszkiewicz J, Woźniak A, Szewczyk-Golec K. Ionizing radiation as a source of oxidative stress-the protective role of melatonin and vitamin D. Int J Mol Sci. 2020;21(16):5804.
- Wang Y, Wang P, Zheng X, et al. Therapeutic strategies of melatonin in cancer patients: a systematic review and meta-analysis. Onco Targets Ther. 2018;11:7895–7908.
- Jackson SP, Bartek J. The DNA-damage response in human biology and disease. Nature. 2009;461:1071–1078.
- Willoughby CE, Jiang Y, Thomas HD, et al. Selective DNA-PKcs inhibition extends the therapeutic index of localized radiotherapy and chemotherapy. J Clin Invest. 2020;130:258–271.
- Ge PS, Runyon BA. Treatment of patients with cirrhosis. N Engl J Med. 2016;375:767–777.
- Sato H, Demaria S, Ohno T. The role of radiotherapy in the age of immunotherapy. Jpn J Clin Oncol. 2021;51:513–522.
- Lee YH, Tai D, Yip C, et al. Combinational immunotherapy for hepatocellular carcinoma: radiotherapy, immune checkpoint blockade and beyond. Front Immunol. 2020;11:568759.
- Zhang X, Chen X, Wang L, et al. Review of the efficacy and mechanisms of traditional Chinese medicines as a therapeutic option for ionizing radiation induced damage. Front Pharmacol. 2021;12:617559.
- Jagetia GC. Radioprotection and radiosensitization by curcumin. Adv Exp Med Biol. 2007;595:301–320.
- Xie YL, Chu JG, Jian XM, et al. Curcumin attenuates lipopolysaccharide/d-galactosamine-induced acute liver injury by activating Nrf2 nuclear translocation and inhibiting NF-kB activation. Biomedicine & Pharmacotherapy = Biomedecine & Pharmacotherapie. 2017;91:70–77.
- He Y, Yue Y, Zheng X, et al. Curcumin, inflammation, and chronic diseases: how are they linked?. Molecules. Basel, Switzerland 2015;20:9183–9213.
- Shen ZT, Chen Y, Huang GC, et al. Aurora-a confers radioresistance in human hepatocellular carcinoma by activating NF-κB signaling pathway. BMC Cancer. 2019;19:1075.
- Yang Q, Xie B, Tang H, et al. Minichromosome maintenance 3 promotes hepatocellular carcinoma radioresistance by activating the NF-κB pathway. J Exp Clin Cancer Res. 2019;38:263.
- Pan CC, Kavanagh BD, Dawson LA, et al. Radiation-associated liver injury. Int J Radiat Oncol Biol Phys. 2010;76:S94–100. physics.