ABSTRACT
Autophagy is essential for the maintenance of intracellular homeostasis, implicated in various biological processes. Forkhead box protein O1 (FOXO1) is regarded as a key mediator regulating skeletal development. Recent studies indicate that FOXO1 has a multifaceted role in autophagy regulation and dysregulation. Here, we aimed to elucidate the role of FOXO1-autophagy axis in osteogenesis. Osteoblast conditional Foxo1-knockout mice (Foxo1OB−/−, KO) and FOXO1 lentivirus overexpression (Len-FoxO1) model were constructed in vivo. Primary osteoblasts were isolated from KO and their wild-type (WT) littermates. And we also applied overexpression lentivirus to investigate the effects of FOXO1 in vitro. Using Micro-CT, fluorescence labeling detection, real-time qPCR and western blot analyses, we found that bone formation was promoted in Len-FOXO1 mice, which was impaired in KO group. Similarly, FOXO1 overexpression enhanced proliferation, migration and differentiation of osteoblasts, while FOXO1 ablation resulted in poor biological functions of osteoblasts. Through the investigation of autophagic process using mRFP-GFP-LC3 fluorescence labeling and co-immunoprecipitation, we observed that overexpression of FOXO1 initiated autophagy induction, with enhanced FOXO1 interaction with autophagy-related protein 7 (ATG7). On the contrary, FOXO1 knockout in osteoblasts impeded FOXO1-ATG7 conjugation, leading to impaired autophagic activity. Furthermore, inhibition of autophagy by chloroquine (CQ) could reverse favorable influences in bone formation induced by FOXO1 overexpression. Our findings confirmed that FOXO1 was an important regulator of bone formation and autophagy might be part of the underlying mechanisms, offering a significant avenue for the potential strategy in the treatment of bone-related disorders.
Introduction
Bone is a highly mineralized and alive organ that undergoes continuous turnover throughout life [Citation1]. It is well modulated by a metabolic network including bone cells to maintain homeostasis through various biological processes [Citation2]. The perturbation of bone (re)modeling and metabolism could lead to skeletal disorders, posing threats to public health.
Recently, the corresponding link between bone homeostasis and autophagy has drawn increasing interest. Autophagy is an essential catabolic process induced under different stimuli in eukaryotes. The primary role of autophagy is to clear up damaged intracellular components, contributing to the maintenance of cellular function and organismal homeostasis [Citation3]. Defect in autophagy regulation is implicated in multiple diseases, such as cancer, metabolic disorders, neurodegenerative diseases and so forth [Citation4]. Previous study has provided supports the association link autophagy genes to human osteoporosis using a genome-wide association analysis [Citation5]. The autophagic process is found to be vital in bone mineralization, and autophagy deficiency could lead to bone loss in mice [Citation6]. On the other hand, autophagy protein-deficient osteoclasts exhibited a poor capacity of bone resorption [Citation7]. These findings have shed light on the potential role of autophagy in the bone metabolism and skeletal development.
As a member of forkhead transcription factors of the O class (FOXO) family, FOXO1 is abundantly located in adipose tissue, bone and liver with key transcriptional regulatory activity [Citation8–10]. It is concerned in controlling various physiological and pathological processes, such as cell survival, proliferation, oxidative stress and energy metabolism [Citation11,Citation12]. Besides, it is regarded as an attractive candidate to orchestra bone development. In mouse embryos, FOXO1 is found to be at a higher expression level in bone tissues, while FOXO1 silencing affects craniofacial development and leads to dampened skeletogenesis [Citation13]. Moreover, the existence of FOXO1 prevents osteoblast apoptosis, thus alleviating aging-induced loss of bone mass [Citation14]. Our previous experiment has also reported that FOXO1 ablation showed a large amount of bone loss in mice [Citation15]. These findings highlight that FOXO1 is indispensable for bone mass homeostasis. Of note, FOXO1 has the potential to protect against several diseases, including cartilage damage, diabetes, etc, through the modulation of autophagic process [Citation16]. However, whether osteoblastic FOXO1-mediated osteogenesis is related to autophagy has not yet been fully addressed.
Based on these, we hypothesize that FOXO1 may regulate bone formation through autophagy. In this report, we herein use Foxo1 conditional knockout mice to investigate the role of FOXO1-mediated autophagy in osteogenesis, which might provide a therapeutic approach for disorders related to bone metabolism.
Materials and methods
Animal study
All animal studies were performed in accordance with the ARRIVE guidelines and the experimental protocol was approved by the Ethics Committee of West China Hospital of Stomatology, Sichuan University (Chengdu, China). All animals were housed in separate cages under controlled conditions (25°C, 55% humidity) with a 12-hour light and 12-hour dark cycle. They were all provided with standard food and water. Foxo1tm1Rdp(Foxo1fl/fl) mice and Bglap-Cre(Cre±) mice were purchased from Jackson Laboratories (Bar Harbor, USA). Foxo1OB-/- mice (KO) were obtained as previously described [Citation17]. Briefly, Foxo1fl/fl mice were crossed with Bglap-Cre± mice first and Foxo1fl/−Cre± mice were obtained. Then, Foxo1fl/−Cre± mice were crossed with Foxo1fl/fl mice to produce osteoblasts conditional Foxo1-knockout mice (Foxo1OB−/−, KO). KO mice and their WT littermates (as control) were used for the following experiments. After that, we applied FOXO1 overexpression lentivirus (Len-FOXO1, GENECHEM Co., Shanghai, China) in WT mice to establish FOXO1 overexpression model. FOXO1 lentiviral vector (10 μl, 2 × 107 TU/ml) was applied by intravenous injection. The breeding process was all proceeded in the laboratory and the experimentation was under strict management. Aged 8-week-old mice were divided into three groups: Len-FOXO1, WT and KO. Each group contained 15 animals. To further confirm the role of autophagy in bone formation, part of mice in WT and Len-FOXO1 group were treated by chloroquine (CQ), which could inhibit the autophagic process. CQ (Biotrend, Cologne, Germany) was intraperitoneally administered to mice with a dose of 2 mg/kg every 2 d as previously described [Citation18]. All mice were sacrificed by anesthesia overdose. Serum and femoral bone tissues were harvested for following studies.
Micro-CT analysis
After mice were sacrificed, the femurs were fixed in 4% paraformaldehyde. Subsequently, the specimens were transferred to 75% ethanol and dried in air. Bone microarchitectures were measured by Micro-CT (SCANCO 50, Switzerland) at a resolution of 7 μm (70kV, 200 μA, 300 ms integration time). The 3D reconstruction images were obtained first. Then the morphometric analysis was performed by the evaluation script of bone tissue: bone volume per total volume (BV/TV), the mean trabecular number (Tb.N) and the mean trabecular separation (Tb.Sp).
Fluorescence double labeling
Fluorescence double labeling was performed to estimate the level of newly formed bones. Mice were injected intraperitoneally with calcein (10 mg/kg) 14 d before sacrifice and alizarin red (20 mg/kg) 4 d before harvesting. Subsequently, the diaphyseal cortical femoral bones were collected to determine the mineral apposition rate (MAR), mineralized surface/bone surface (MS/BS) and bone formation rate per bone surface (BFR/BS).
Enzyme-linked immunosorbent assay (ELISA)
Blood serum from mice was harvested and centrifuged. Procollagen type 1 N-terminal propeptide (P1NP) and c-telopeptide of type 1 collagen (CTX) were examined by ELISA kit from IDS (Fountain Hills, AZ) following the manufacturer’s instructions.
Immunohistochemistry
Distal femora from different groups were collected and fixed by 4% neutral Paraformaldehyde. After that, they were decalcified in 10% ethylene diamine tetraacetic acid (EDTA) and dehydrated following a graded series of ethanol solutions. Then specimens were embedded in paraffin, sawed into 5 μm longitudinal sections and immunostained for runt-related transcription factor 2 (RUNX2, Abcam).
Cell culture and treatment
Osteoblasts were isolated from calvaria of newborn WT and KO mice. Cells were cultured in α-MEM (Gibco, USA) supplemented with 10% fetal bovine serum (FBS, Gibco, USA). To establish the FOXO1 overexpression model in vitro, osteoblasts isolated from WT mice were transfected with lentivirus-FOXO1 (Len-FOXO1, GENECHEM Co., Shanghai, China) at a multiplicity of infection (MOI) of 40. After incubation for 10 h, the transfected cells were cultured in normal α‐MEM with 10% FBS. Therefore, cells were divided into following groups: (1) Len-FOXO1 group; (2) WT group; (3) KO group.
Transwell assay
Transwell assay was conducted to estimate cell migration. First, α‐MEM containing 10% FBS was added to the lower chambers and osteoblasts were plated into the upper chambers at a density of 5 × 104 cells per well with serum‐free α‐MEM. After 12 h and 24 h culture, the chambers were washed by PBS and then fixed. Unmigrated cells were removed with cotton swabs and migrated cells were measured by cell counting.
Cell proliferation assay
Cells were seeded at a density of 5 × 103 per well in 96-well plates. At 1 d, 3 d and 5 d, the proliferative activity of osteoblasts was detected according to the Cell Counting Kit‐8 (CCK‐8, Jiancheng, Nanjing, China) manual. The optical density (OD) value was tested at 450 nm by a microplate reader (NanoDrop, USA).
Real-time qPCR
For in vivo test, distal femurs were harvested and total RNA from these specimens was extracted using FastPure® Cell/Tissue Total RNA Isolation Kit V2 (Vazyme, Nanjing, China). Then, reverse transcriptions were carried out and cDNA was synthesized by Prime Script Reverse Transcriptase (Takara, Japan). Using SYBR Premix Ex Taq (Takara, Japan), real-time qPCR was performed by ABI 7300 real-time PCR system (Applied Biosystems, USA). The mRNA expressions of osteogenic biomarkers, including Runx2, Osterix/Sp7 (encoded by the Sp7 gene) and alkaline phosphatase (Alpl), were examined. Glyceraldehyde-3-phosphate dehydrogenase (Gapdh) was used as loading control. Primer sequences were listed in .
Table 1. Primer sequences for real time-qPCR.
Western blot analysis
Cells were subjected to lysis buffer (Keygen total protein extraction kit, Keygen Biotech, China) and then centrifuged at 14,000 g for 15 min. After that, the supernatant concentrations from different samples were analyzed by a bicinchoninic acid (BCA) assay (Beyotime, China). Then, protein extracts were applied to sodium dodecyl sulfate polyacrylamide gel electrophoresis (SDS‐PAGE) and transferred onto polyvinylidene difluoride membranes (Millipore Corp., USA). After blocking at room temperature for 1 h, the membranes were probed with primary antibodies overnight. The next day, they were incubated with HRP-conjugated secondary antibodies (1:2000) and washed with Tris‐buffered saline with Tween 20. The immunoreactive bands were computer analyzed by Quantity One (Bio-Rad, USA) and immunoblot images were photographed. The primary antibodies used in this study were as follows: RUNX2 (1:500, Abcam), SP7 (1:500, Abcam), ALPL (1:500, Abcam), LC3 (1:1000, Cell Signaling Technology), BECN1 (1:500, Abcam), ACTB (1:1000, SAB) was used as internal control.
ALPL activity and alizarin red staining
Cells were cultured in osteogenic induction medium (α-MEM, 10% FBS, 10mML-ascorbic acids, antibiotics, 10 mM β-glycerophosphate, and 100 nM dexamethasone (Gibco, USA)). After 7-d osteogenic induction, cells were collected, washed with PBS and fixed by 4% paraformaldehyde for 30 minutes. After that, samples were stained by ALPL staining kit (Beyotime, China) following the manual. Additionally, ALPL activity was determined at 4 d and 8 d according to the protocol provided by ALPL Assay Kit (Beyotime, China). Total protein concentration of each sample was analyzed by BCA Assay Kit (Beyotime, China). Then the ALPL activity results were normalized to the total protein content.
For alizarin red staining, osteoblasts were cultured in osteogenic induction medium for 21 d. Then cells were fixed in 4% paraformaldehyde and stained by alizarin red (OriCell, USA). After that, cetylpyridinium chloride (10%) were added into plates for 15 min. Then the OD values were detected at 562 nm to quantitatively assess calcium deposits.
Fluorescence labeling of mRFP-GFP-LC3
Cells were seeded at a density of 5 × 104 per well in 24-well plates. When cells reached 70–80% confluence, fluorescence double labeling was performed using mRFP-GFP-LC3 adenovirus (Hanbio Bio-technology Co., Ltd., Shanghai, China) to quantify autophagic flux according to the manufacturer’s protocol. After 6-h transfection, the culture medium was replaced by normal α-MEM with 10% FBS. Subsequently, cells in all groups were collected after 36 h and observed by confocal laser scanning microscope (Olympus, Tokyo, Japan).
Co-immunoprecipitation (co-IP)
Seeded at a density of 1 × 105 cells per well in 6-well plates, cells in all groups were harvested at 7 d. The methods of co-IP were carried out as previously reported [Citation19]. In brief, samples were lysed in RIPA buffer (Thermo Fisher Scientific, USA), and then centrifuged at 13,000 g at 4°C for 10 min. The cell lysates were incubated with protein A/G-agarose for 2 h at 4°C for preclearing. Specific antibodies including autophagy-related protein 7 (ATG7,1:500, Abcam), FOXO1 (1:500, Abcam) and ACTB (as internal control, 1:1000, SAB) were incubated overnight at 4°C followed by protein-A/G agarose beads incubation at 4°C for 4 h. After that, the beads were washed several times, mixed with loading buffer and boiled at 95°C for 5 minutes for later Western blot analysis as described above.
Statistical analysis
Statistical analysis was performed by SPSS 20.0 software (SPSS, Inc., Chicago, IL). The results are expressed as mean ± SD with a minimum of three independent samples. Data were evaluated using one-way ANOVA followed by Student-Newman-Keuls (SNK) tests to compare group differences. Values of p < 0.05 were considered to be statistically significant (*p < 0.05, **p < 0.01, ***p < 0.001, ****p < 0.001).
Results
FOXO1 is essential for bone formation in mice
First, we detected the mRNA expression of Foxo1 and protein FOXO1 expression to examine the efficiency of the lentiviral transduction or conditional knockout, respectively (Fig S1A, C). Additionally, FOXO1 overexpression or knockout had neglectable effects on the levels of Foxo3 and Foxo4 mRNA, other two members of FOXO family (Fig S2A). In order to investigate the role of FOXO1 in bone formation, micro-CT analysis was performed to detect the details of bone mass and microstructure. Through the micro-CT 3D reconstruction, Len-FOXO1 group showed an increased amount of bone mass in distal femur compared with WT group. While bone volume in trabecular bone compartment in KO group significantly decreased (). Meanwhile, bone morphological parameters including BV/TV, Tb.N and Tb.Sp were analyzed. FOXO1 overexpression in bone tissues enhanced BV/TV and Tb.N to a large extent, and led to a lower level of Tb.Sp in femur. However, deletion of FOXO1 exhibited opposite effects, showing a sharp decline in BV/TV and Tb.N and a remarkable rise in Tb.Sp (). Besides, fluorescent bone labels were analyzed in distal femur. Quantitative measurements of MAR, MS/BS and BFR/BS were enhanced in mice transfected with FOXO1 lentivirus. But the interlabel width and these parameters (MAR, MS/BS and BFR/BS) dramatically decreased caused by FOXO1 ablation (), which manifested that FOXO1 deficiency impaired bone formation.
Figure 1. The role of FOXO1 in bone formation in mice. (a) three-dimensional reconstruction of distal femurs by Micro-CT. Scale bar = 200 μm. (b) Micro-CT analysis of bone volume per total volume (BV/TV), the mean trabecular number (Tb.N) and the mean trabecular separation (Tb.Sp). (c) double fluorescence labeling of calcein and alizarin red. Scale bar = 25 μm (d) quantitative analysis of mineral apposition rate (MAR), mineralized surface/bone surface (MS/BS) and bone formation rate per bone surface (BFR/BS). Data are expressed as means ± SD, n = 3–6 specimens/group, *p < 0.05, **p < 0.01, ***p < 0.001, ****p < 0.0001.
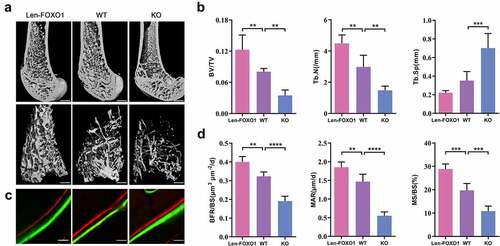
Additionally, two typical serum markers, P1NP and CTX, were evaluated respectively. P1NP is a biomarker for bone formation, and CTX for bone resorption. Results showed that the serum level of P1NP increased in Len-FOXO1 mice when compared to WT mice, while the P1NP content was significantly downregulated in KO mice. On the other hand, we found that Len-FOXO1 group led to a lower level of serum CTX, yet a higher level of CTX shown in KO group (). As observed through real-time qPCR shown in , the mRNA expressions of Runx2, Sp7 and Alpl were promoted in FOXO1 overexpressed mice. But these osteogenic markers were inhibited in conditional FOXO1-knockout mice. Consistently, the protein expression levels of RUNX2, SP7 and ALPL showed similar variations through the western blots assay (). These data suggested that FOXO1 plays a vital role in bone formation and skeletal integrity in mice.
Figure 2. FOXO1 influence on osteogenesis in vivo. (a) the serum level of procollagen type 1 N-terminal propeptide (P1NP) and c-telopeptide of type 1 collagen (CTX). (b) real-time qPCR analysis of runt-related transcription factor 2 (Runx2), Sp7 and alkaline phosphatase (Alpl). (c-d) representative western blot images and quantitative analysis of the protein expressions of RUNX2, SP7 and ALPL. Values are presented as mean ± SD, n = 3–6 specimens/group, ns represents p>0.05, *p<0.05, **p<0.01, ***p<0.001, ****p<0.0001.
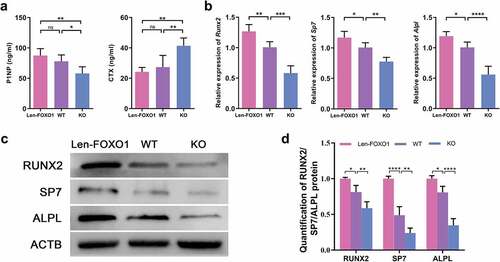
FOXO1 maintains osteoblasts viability and osteogenic differentiation potential
Osteoblasts were isolated from WT and KO mice to further explore the role of FOXO1 in osteogenesis in vitro. Len-FOXO1 group exhibited significant upregulation of the mRNA expression of Foxo1 and protein FOXO1 expression in osteoblasts. Meanwhile, real-time qPCR and western blot results showed successful silencing of FOXO1 in KO group (Fig S1B, D). Besides, we found that FOXO1 overexpression or deletion had neglectable influences on Foxo3 and Foxo4 expression (Fig S2B). Len-FOXO1 group displayed a moderate increase in the migratory response of osteoblasts (P>0.05). In contrast, the cell migration remarkably reduced at 12 h and 24 h in KO group compared to WT group, with statistical significance (). For cell proliferation, results from CCK-8 test showed that overexpression of FOXO1 had neglect effect on cell viability. While FOXO1 deletion in osteoblasts suppressed cell proliferative rate, especially at 3 d and 5 d (). As shown in , the enhanced ALPL activity was detected in Len-FOXO1 osteoblasts, while FOXO1 deficiency exerted inhibitory effect. In addition, FOXO1 overexpression resulted in more calcium nodules after 21-d osteogenic induction shown in alizarin red staining and the quantitative analysis. Nevertheless, fewer alizarin red-positive nodules were calculated in KO group, indicating the impaired capacity of osteogenic differentiation for osteoblasts. In accordance with the observations described above, the mRNA expression levels of Runx2, Sp7 and Alpl displayed similar variations (). Taken together, these results implied that FOXO1 is an important factor in regulating biological functions of osteoblasts and FOXO1 ablation impaired differentiation and mineralization properties of osteoblasts.
Figure 3. Effects of FOXO1 on biological functions of osteoblasts. (a) quantitation of the migratory response of osteoblasts. (b) cell proliferation detected by CCK-8 assay. (c) ALPL staining and alizarin red staining of osteogenic differentiation induction. (d) ALPL activity at 4d and 8d. (e) quantitative analysis of alizarin red staining at 562 nm absorbance. (f-h) Real-time qPCR analysis of runt-related transcription factor 2 (Runx2), Sp7 and alkaline phosphatase (Alpl). All data are presented as mean ±SD of 3 independent experiments, *p<0.05, **p<0.01, ***p<0.001, ****p<0.0001.
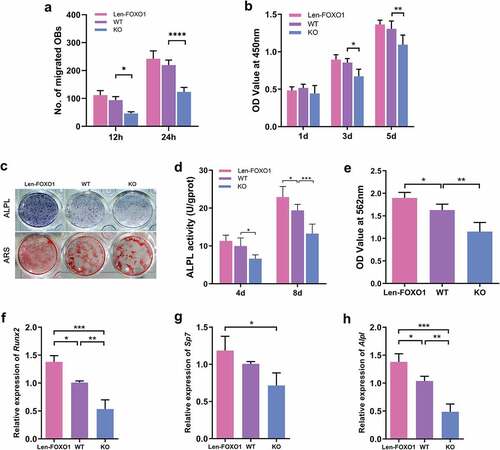
FOXO1-mediated autophagy is involved in the regulation of osteogenesis
To explore the role of autophagy in FOXO1-mediated osteogenesis, western blot analysis was performed to evaluate the LC3II/LC3I ratio and the expression of BECN1, known as key markers for monitoring autophagic activity [Citation20,Citation21]. It was observed that the BECN1 expression and LC3II/LC3I ratio in osteoblasts were significantly upregulated in the Len-FOXO1 group as compared to WT group, while expressions of these markers were downregulated sharply in KO group (). The results of mRFP-GFP-LC3 fluorescence double labeling further confirmed a higher level of autophagic flux in Len-FOXO1 group (). RFP (Red fluorescent protein) signal corresponds to autolysosomes. After lysosomes and autophagosomes fuse to autolysosomes, GFP (green fluorescent protein) would weaken even quench, since it is sensitive to acidic conditions of the lysosome lumen. And yellow puncta (colocalization of green and red fluorescence) referred to autophagosomes. Thus, increased number of yellow and red puncta indicated the augmentation of autophagic flux [Citation22]. As displayed in , representative images and quantitative analysis in KO group indicated lower autophagic flux, reflected in a smaller number of autolysosomes and autophagosomes. Next, co-IP was performed to further investigate the underlying mechanisms involved. Enhanced interaction between FOXO1 and ATG7 was observed in the Len-FOXO1 group, while FOXO1 binding to ATG7 reduced significantly in the KO group ().
Figure 4. The impacts of FOXO1 on autophagy in osteoblasts. (a-b) protein expression levels of LC3 and BECN1 tested by western blot assay. (c) representative images of mRFP-GFP-LC3 fluorescence double labeling. Red puncta refer to the autolysosomes and yellow indicate the autophagosomes in cells. (d) quantification of mRFP-GFP-LC3 fluorescence double labeling analysis. (e-f) analysis of co-immunoprecipitation (co-IP). Values are expressed as mean ± standard deviation (SD) of 3 independent experiments, *p<0.05, **p<0.01, ***p<0.001.
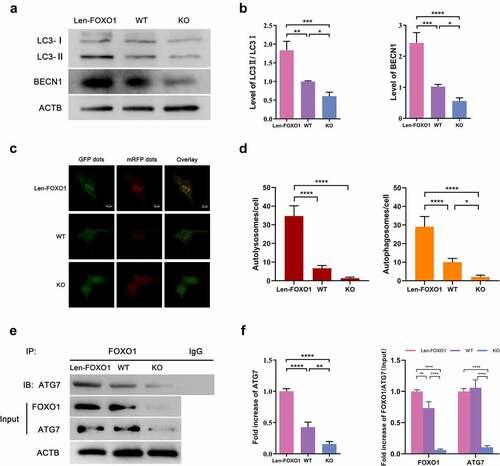
To further confirm the potential of autophagy in bone formation, CQ was used to inhibit autophagy pharmacologically. Compared with WT controls, WT mice treated with CQ exhibited significantly lower BV/TV and Tb.N, yet a higher level of Tb.Sp in femur. Then CQ reversed favorable osteogenic effects of Len-FOXO1, resulting in impaired femur bone density with lower level of BV/TV and Tb.N and higher level of Tb.Sp (). Although the expression level of Runx2 was higher in FOXO1 overexpressed mice compared to WT mice, it was found that the treatment of CQ led to significantly reduced RUNX2 expression in both WT and Len-FOXO1 group according to immunohistochemistry (). Consistent with these findings, the real-time qPCR results of bone tissues indicated a remarkable upregulation of Runx2, Sp7 and Alpl expressions in Len-FOXO1 group. In contrast, mRNA expressions of these osteogenic markers were downregulated sharply in Len-FOXO1 mice by treatment with CQ (). These observations proved that FOXO1-mediated autophagy was implicated in the regulation of osteogenesis.
Figure 5. The role of FOXO1-mediated autophagy in bone formation. (a) three-dimensional reconstruction of distal femur by Micro-CT. Scale bar = 200 μm. (b) micro-CT measurement ofbone volume per total volume (BV/TV), the mean trabecular number (Tb.N) and the mean trabecular separation (Tb.Sp). (C) Immunohistochemical staining of RUNX2 in distal femur. Scale bar = 25 μm. (d) quantitative analysis for mRNA expressions of runt-related transcription factor 2 (Runx2), Sp7 and alkaline phosphatase (Alpl) by real-time qPCR. Data are presented as means ± SD, n = 3–6 specimens/group, *p < 0.05, **p < 0.01, ***p < 0.001, ****p < 0.0001.
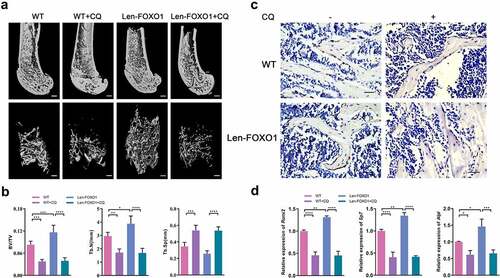
Discussion
Accumulating evidence has indicated the regulatory role of FOXO1 in the bone metabolism. In this study, we chose Foxo1 conditional deletion in osteoblasts to explore its specific effects in skeleton homeostasis. In vivo, FOXO1 deficiency contributed to impaired bone formation rate in mice, which was in accordance with the previous experiment revealing that the silence of FOXO1 dampened bone growth [Citation13]. This previous study exhibited harmful effects of FOXO1 deletion on craniofacial bone development, and our experiment proved that it also could affect the skeletogenesis of long bones [Citation15]. These findings suggested that the existence of FOXO1 was indispensable for skeletal development. In vitro, we found that FOXO1 was responsible for the promoted proliferation, differentiation, and migration of osteoblasts. The effects of FOXO1 on osteoblast physiology were similar in pre-osteoblastic cells [Citation23,Citation24]. It should be noted that FOXO1 might exert inverse effects under different conditions. In hyperglycemia situation, FOXO1 intactness was associated with decreased osteoblast number, thus causing a reduction in bone mass and strength [Citation25]. Therefore, FOXO1 might be an important candidate to positively regulate bone formation in physiological condition, while its influences in the bone metabolism might exhibit in a more complicated mechanism in those pathological situations. Apart from the FOXO1 influences on osteogenesis, it is worth noting that the other two members of FOXO family, FOXO3 and FOXO4, are also expressed in bone tissue and their actions in bone-related cells might be critical for skeletal development [Citation26]. In this study, we found that the expression of FOXO3/4 was not affected in FOXO1 overexpression or knockout group both in vivo and in vitro, which further confirmed the crucial role of FOXO1 in regulating bone formation.
In recent years, there is a growing number of research demonstrating that autophagy plays a pivotal role in the maintenance of bone homeostasis. Dysregulation of autophagic process has been found to be associated with bone metabolic disorders including osteolysis, osteoporosis and osteoarthritis [Citation27]. Induced autophagy participates in cellular apoptosis, differentiation and mineralization, thus strengthening the osteogenic ability of several types of bone-related cells [Citation6,Citation28,Citation29]. In addition, it is reported that autophagy has a favorable influence on bone remodeling and metabolism through controlling the balance of oxidative stress or modulating osteoimmunology [Citation30,Citation31]. Our in vitro results confirmed the promotive role of autophagy in the modulation of osteoblast bioactivity. Moreover, we were intrigued to find that the inactivation of autophagy caused by CQ impeded bone formation in vivo. These findings supported that autophagy was closely related with osteogenesis and inhibition of autophagy could contribute to bone loss, proving the significant role of autophagy in bone formation.
Apart from osteoblasts and bone formation, the involvement of autophagy in osteoclasts, another important cell type participating in the bone mineral homeostasis, has been reported. As mentioned above, increasing autophagy contributed to enhanced osteoblastic functions, but it could also lead to the promotion of osteoclast differentiation and bone resorption. Interestingly, mice with defect in autophagy showed an increased cortical bone thickness caused by impaired biological properties of osteoclasts. And the inactivation of autophagy could block the production of osteoclasts, thereby alleviating bone loss caused by glucocorticoid or ovariectomy [Citation18]. These conflicting facts implied that autophagy might serve different skeletal functions in different types of bone cells. Thus, the appropriate controlling of autophagic process might become an interesting approach for the regulation of bone metabolism, and it is necessary to consider the impacts of autophagy on overall remodeling of the skeletal system.
Increasing evidence has linked FOXO1 to autophagy regulation in various physiological and pathological processes. Our work shed light on that the FOXO1-autophagy axis was involved in the osteogenesis. It should be noted that the role of FOXO1 in the modulation of autophagic process is complex. Several factors such as SIRT1, high mobility group box 1 (HMGB1), etc, have been shown to affect FOXO1 transcription, thereby modulating autophagy in response to different cellular environments [Citation32,Citation33]. Subsequently, how FOXO1 affects autophagic process has been the subject of hotspot in this field. On one hand, FOXO1 can induce autophagy through binding to the genes encoding autophagy proteins and upregulates gene expressions in the nucleus, which requires the translocation of FOXO1 from the cytoplasm into the nucleus [Citation34]. On the other hand, FOXO1 can be excluded from nucleus to the cytosolic compartment, interacting with autophagy-related proteins [Citation35]. ATG7, an E1-like enzyme, is implicated in autophagosome formation and essential for autophagy induction [Citation36]. Shen et al. has reported that ATG7 might act as a FOXO1 binding partner in the regulation of FOXO1 acetylation-dependent autophagy in response to oxidative stimulation [Citation37]. Considering that, we speculated that FOXO1/ATG7-mediated autophagy could also play a crucial role in the process of osteogenesis. Here, we proved that ATG7 could be coprecipitated by the FOXO1 in osteoblasts. And our findings suggested that FOXO1 could induce autophagic process by interacting with ATG7 in the cytoplasm, providing a novel view of FOXO1 regulation of autophagy in the osteogenesis. Of note, recent investigations also pose a question about how FOXO1 coordinates its nuclear versus cytosolic roles in autophagy, since both cytosolic and nuclear regulation of FOXO1 appear to be important for autophagy activation. Further studies on this matter need to be investigated in the future. Collectively, our study still makes a significant, if not sufficient, contribution to the overview about the functional importance of FOXO1/ATG7-mediated autophagy in the bone formation.
Conclusions
In summary, the results of the study revealed that FOXO1 exerts positive effects on osteogenesis in vivo and in vitro, thereby playing a key role in bone formation and skeletal development. In this process, ATG7-mediated autophagy was involved in the favorable impacts of FOXO1 on osteogenesis. Our findings provided broader perspectives for the development of therapeutics in bone-related disorders.
Supplemental Material
Download MS Word (406 KB)Acknowledgements
This work was supported by National Natural Science Foundation of China (82001084), Sichuan Science and Technology Program (2020JDRC0055), West China Hospital Basic and Applied Basic Research Foundation (RD-02-201913) and China Postdoctoral Science Foundation (2019M653442).
Disclosure statement
No potential conflict of interest was reported by the author(s).
Data availability statement
The data that support the findings of this study are available from the corresponding author, [YX], upon reasonable request.
Supplementary material
Supplemental data for this article can be accessed online at https://doi.org/10.1080/15384101.2022.2155443
Additional information
Funding
References
- Suzuki A, Minamide M, Iwaya C, et al. Role of metabolism in bone development and homeostasis. Int J Mol Sci. 2020 Nov 26;21(23):8992. DOI:10.3390/ijms21238992
- Datta HK, Ng WF, Walker JA, et al. The cell biology of bone metabolism. J Clin Pathol. 2008 May;61(5):577–587. DOI:10.1136/jcp.2007.048868
- Yu L, Chen Y, Tooze SA. Autophagy pathway: cellular and molecular mechanisms. Autophagy. 2018;14(2):207–215.
- Ravanan P, Srikumar IF, Talwar P. Autophagy: the spotlight for cellular stress responses. Life Sci. 2017 Nov 1;188:53–67. DOI:10.1016/j.lfs.2017.08.029
- Zhang L, Guo YF, Liu YZ, et al. Pathway-based genome-wide association analysis identified the importance of regulation-of-autophagy pathway for ultradistal radius BMD. J Bone Miner Res. 2010 Jul;25(7):1572–1580. DOI:10.1002/jbmr.36
- Nollet M, Santucci-Darmanin S, Breuil V, et al. Autophagy in osteoblasts is involved in mineralization and bone homeostasis. Autophagy. 2014;10(11):1965–1977. DOI:10.4161/auto.36182
- DeSelm CJ, Miller BC, Zou W, et al. Autophagy proteins regulate the secretory component of osteoclastic bone resorption. Dev cell. 2011 Nov 15;21(5):966–974.
- Chen D, Gong Y, Xu L, et al. Bidirectional regulation of osteogenic differentiation by the FOXO subfamily of forkhead transcription factors in mammalian MSCs. Cell Proliferation. 2019 Mar;52(2):e12540.
- Nakae J, Kitamura T, Kitamura Y, et al. The forkhead transcription factor Foxo1 regulates adipocyte differentiation. Dev cell. 2003 Jan;4(1):119–129.
- Puigserver P, Rhee J, Donovan J, et al. Insulin-regulated hepatic gluconeogenesis through FOXO1-PGC-1alpha interaction. Nature. 2003 May 29;423(6939):550–555. DOI:10.1038/nature01667
- Peng S, Li W, Hou N, et al. A review of FoxO1-regulated metabolic diseases and related drug discoveries. Cells. 2020 Jan 10;9(1):184. DOI:10.3390/cells9010184
- Xing YQ, Li A, Yang Y, et al. The regulation of FOXO1 and its role in disease progression. Life Sci. 2018 Jan 15;193:124–131. DOI:10.1016/j.lfs.2017.11.030
- Teixeira CC, Liu Y, Thant LM, et al. Foxo1, a novel regulator of osteoblast differentiation and skeletogenesis. J Biol Chem. 2010 Oct 1;285(40):31055–31065. DOI:10.1074/jbc.M109.079962
- Ambrogini E, Almeida M, Martin-Millan M, et al. FoxO-mediated defense against oxidative stress in osteoblasts is indispensable for skeletal homeostasis in mice. Cell Metab. 2010 Feb 3;11(2):136–146.
- Zhang Y, Xiong Y, Zhou J, et al. FoxO1 expression in osteoblasts modulates bone formation through resistance to oxidative stress in mice. Biochem Biophys Res Commun. 2018 Sep 10;503(3):1401–1408. DOI:10.1016/j.bbrc.2018.07.055
- Matsuzaki T, Alvarez-Garcia O, Mokuda S, et al. FoxO transcription factors modulate autophagy and proteoglycan 4 in cartilage homeostasis and osteoarthritis. Sci, trans med. 2018 Feb 14;10(428). DOI:10.1126/scitranslmed.aan0746
- Xiong Y, Zhang Y, Xin N, et al. 1alpha,25-Dihydroxyvitamin D3 promotes bone formation by promoting nuclear exclusion of the FoxO1 transcription factor in diabetic mice. J Biol Chem. 2017 Dec 8;292(49):20270–20280. DOI:10.1074/jbc.M117.796367
- Lin NY, Chen CW, Kagwiria R, et al. Inactivation of autophagy ameliorates glucocorticoid-induced and ovariectomy-induced bone loss. Ann Rheumatic Dis. 2016 Jun;75(6):1203–1210. DOI:10.1136/annrheumdis-2015-207240
- Liu K, Guo C, Lao Y, et al. A fine-tuning mechanism underlying self-control for autophagy: deSumoylation of BECN1 by SENP3. Autophagy. 2020 Jun;16(6):975–990.
- Levine B, Liu R, Dong X, et al. Beclin orthologs: integrative hubs of cell signaling, membrane trafficking, and physiology. Trends Cell Biol. 2015 Sep;25(9):533–544.
- Schaaf MB, Keulers TG, Vooijs MA, et al. LC3/GABARAP family proteins: autophagy-(un)related functions. FASEB J. 2016 Dec;30(12):3961–3978. DOI:10.1096/fj.201600698R
- Mizushima N, Yoshimori T, Levine B. Methods in mammalian autophagy research. Cell. 2010 Feb 5;140(3):313–326.
- Komori T. Regulation of bone development and extracellular matrix protein genes by RUNX2. Cell tissue res. 2010 Jan;339(1):189–195. DOI:10.1007/s00441-009-0832-8
- Siqueira MF, Flowers S, Bhattacharya R, et al. FOXO1 modulates osteoblast differentiation. Bone. 2011 May 1;48(5):1043–1051. DOI:10.1016/j.bone.2011.01.019
- Iyer S, Han L, Ambrogini E, et al. Deletion of FoxO1, 3, and 4 in osteoblast progenitors attenuates the loss of cancellous bone mass in a mouse model of type 1 diabetes. J Bone Miner Res. 2017 Jan;32(1):60–69. DOI:10.1002/jbmr.2934
- Kim HN, Iyer S, Ring R, et al. The role of FoxOs in bone health and disease. Curr Top Dev Biol. 2018;127:149–163.
- Wang S, Deng Z, Ma Y, et al. The role of autophagy and mitophagy in bone metabolic disorders. Int J Biol Sci. 2020;16(14):2675–2691.
- Liu Z, Li T, Zhu F, et al. Regulatory roles of miR-22/redd1-mediated mitochondrial ROS and cellular autophagy in ionizing radiation-induced BMSC injury. Cell Death Amp Dis. 2019 Mar 7;10(3):227. DOI:10.1038/s41419-019-1373-z
- Ma Y, Qi M, An Y, et al. Autophagy controls mesenchymal stem cell properties and senescence during bone aging. Aging Cell. 2018 Feb;17(1). DOI:10.1111/acel.12709
- Xiao L, Xiao Y. The autophagy in osteoimmonology: self-eating, maintenance, and beyond. Front Endocrinol (Lausanne). 2019;10:490.
- Yun HR, Jo YH, Kim J, et al. Roles of autophagy in oxidative stress. Int J Mol Sci. 2020 May 6;21(9). DOI:10.3390/ijms21093289
- Hariharan N, Maejima Y, Nakae J, et al. Deacetylation of FoxO by sirt1 plays an essential role in mediating starvation-induced autophagy in cardiac myocytes. Circ Res. 2010 Dec 10;107(12):1470–1482. DOI:10.1161/circresaha.110.227371
- Lin Q, Fan C, Gomez-Arroyo J, et al. HIMF (Hypoxia-induced mitogenic factor) signaling mediates the HMGB1 (high mobility group box 1)-dependent endothelial and smooth muscle cell crosstalk in pulmonary hypertension. Arteriosclerosis Thrombosis Vasc Biol. 2019 Dec;39(12):2505–2519. DOI:10.1161/atvbaha.119.312907
- Cheng Z. The FoxO-autophagy axis in health and disease. Trends Endocrinol Metab. 2019 Sep;30(9):658–671. DOI:10.1016/j.tem.2019.07.009
- Wang S, Xia P, Huang G, et al. FoxO1-mediated autophagy is required for NK cell development and innate immunity. Nat Commun. 2016 Mar 24;7:11023. DOI:10.1038/ncomms11023
- Ohsumi Y, Mizushima N. Two ubiquitin-like conjugation systems essential for autophagy. Sem in Cell Develop Biolog. 2004 Apr;15(2):231–236. DOI:10.1016/j.semcdb.2003.12.004
- Shen M, Cao Y, Jiang Y, et al. Melatonin protects mouse granulosa cells against oxidative damage by inhibiting FOXO1-mediated autophagy: implication of an antioxidation-independent mechanism. Redox Biol. 2018 Sep;18:138–157. DOI:10.1016/j.redox.2018.07.004