Abstract
Objective: Derive lower leg injury risk functions using survival analysis and determine injury reference values (IRV) applicable to human mid-size male and small-size female anthropometries by conducting a meta-analysis of experimental data from different studies under axial impact loading to the foot–ankle–leg complex.
Methods: Specimen-specific dynamic peak force, age, total body mass, and injury data were obtained from tests conducted by applying the external load to the dorsal surface of the foot of postmortem human subject (PMHS) foot–ankle–leg preparations. Calcaneus and/or tibia injuries, alone or in combination and with/without involvement of adjacent articular complexes, were included in the injury group. Injury and noninjury tests were included. Maximum axial loads recorded by a load cell attached to the proximal end of the preparation were used. Data were analyzed by treating force as the primary variable. Age was considered as the covariate. Data were censored based on the number of tests conducted on each specimen and whether it remained intact or sustained injury; that is, right, left, and interval censoring. The best fits from different distributions were based on the Akaike information criterion; mean and plus and minus 95% confidence intervals were obtained; and normalized confidence interval sizes (quality indices) were determined at 5, 10, 25, and 50% risk levels. The normalization was based on the mean curve. Using human-equivalent age as 45 years, data were normalized and risk curves were developed for the 50th and 5th percentile human size of the dummies.
Results: Out of the available 114 tests (76 fracture and 38 no injury) from 5 groups of experiments, survival analysis was carried out using 3 groups consisting of 62 tests (35 fracture and 27 no injury). Peak forces associated with 4 specific risk levels at 25, 45, and 65 years of age are given along with probability curves (mean and plus and minus 95% confidence intervals) for PMHS and normalized data applicable to male and female dummies. Quality indices increased (less tightness-of-fit) with decreasing age and risk level for all age groups and these data are given for all chosen risk levels.
Conclusions: These PMHS-based probability distributions at different ages using information from different groups of researchers constituting the largest body of data can be used as human tolerances to lower leg injury from axial loading. Decreasing quality indices (increasing index value) at lower probabilities suggest the need for additional tests. The anthropometry-specific mid-size male and small-size female mean human risk curves along with plus and minus 95% confidence intervals from survival analysis and associated IRV data can be used as a first step in studies aimed at advancing occupant safety in automotive and other environments.
Introduction
In order to determine human tolerance to injury, it is important to understand field data, propose hypotheses for traumas encountered in real-world events based on current knowledge of their potential mechanisms, conduct experiments to reproduce such injuries in a laboratory, obtain biomechanical metrics for quantification, and derive risk curves using statistical techniques. Focusing on leg injuries in motor vehicle environments, studies have been published regarding their prevalence and mechanism (Austin Citation2012; Burgess et al. Citation1995; Dischinger et al. Citation1994, 2004; Eigen and Glassbrenner Citation2003; Estrada et al. Citation2004; Lestina et al. Citation1992). Field data show that 3-point belt–restrained front seat occupants in frontal impacts sustain fractures to the foot–ankle distal tibia complex. Recent field data analysis using the United States extracted from the NASS-CDS for the years 1994 to 2007 and confined to vehicle model years 1995 or greater show that belted front seat occupants in frontal and offset impacts sustain more foot–ankle and knee injuries than pelvic injuries (Rudd Citation2009). Injuries are focused on the calcaneus and tibia complex with and without the involvement of their articular surfaces. The study focused specifically on leg and foot–ankle injuries and occupant factors. These injuries increased with increasing levels of toe pan intrusion into the occupant compartment space, implying the direct transmission of contact loading to the leg. The author commented that despite improvements in vehicle structures and restraint components for enhanced performance in the US New Car Assessment Program and compliance tests, the risk of sustaining foot–ankle injuries merits further work, and this includes an examination of risk curves for this body region (FMVSS-208 2000).
Similar findings have also been reported in earlier studies. States (Citation1986) suggested direct contact load transmission via the vehicle interior as the cause for foot–ankle injuries. Morgan et al. (Citation1991) reported that contact with foot controls accounts for approximately one half of foot–ankle injuries. Pattimore et al. (Citation1991) reported that intrusion and contact with the vehicular interior are causal factors. Similar results were reported in a review of patient records (Lestina et al. Citation1992). Dischinger et al. (Citation1994) examined lower extremity injury data from 42 frontal seat occupants in nonejection and nonrollover crashes. Foot–ankle injuries accounted for approximately two thirds of all lower extremity traumas. Impact loading in the axial mode due to direct contact with the vehicle interior was attributed to be the cause of approximately 70% of all injuries. Kuppa and Sieveka (Citation1995) examined vehicle frontal crash tests to associate the dynamic behavior of the brake pedal/toe pan with axial loads transmitted through the feet of the Hybrid III dummy. Fildes et al. (Citation1995) reported that compression and crushing are frequent mechanisms of foot and ankle trauma. Jibril et al. (Citation1998) examined 11 to 1 o’clock frontal impacts with change in velocities ranging from 24 to 40 km/h and found that crashes with 150 mm intrusion are nearly 5 times more likely to receive at least one moderate to serious leg injury for front seat occupants than occupants with < 25 mm of intrusion. More recently, from a query of the non-population-based U.S. Crash Injury Research and Engineering Network's database, Dischinger et al. (Citation2004) reported that intrusion to the occupant space and contact with the interior component of the vehicle induce direct loading to the leg in frontal impacts.
These briefly described earlier and recent field-based studies clearly show that fractures to anatomical regions of the foot–ankle–leg complex—that is, calcaneus, distal tibia, and pylon fractures, which include the intra-articular surface of this region—are attributed to contact-induced axial loading from the vehicle interior component to the dorsal surface of the foot. Laboratory studies have been conducted by researchers to reproduce these injuries in postmortem human subjects (PMHS) and biomechanical data have been reported, which include the maximum axial load sustained by the specimen and its demographics, such as age, total body mass, and sex. However, data analysis has been largely limited to fundamental mean and standard deviation–type force magnitudes for fracture, double-censored risk curve estimation, or survival analysis without combining data from different groups (Begeman and Aekbote Citation1996; Funk et al. Citation2002; Kitagawa et al. Citation1998; McKay and Bir Citation2009; Roberts et al. Citation1993; Yoganandan, Pintar, et al. Citation1996; Yoganandan et al. Citation1997). Because of the availability of a relatively large body of published data for approximately 2 decades, it is important and timely to synthesize and examine the risk of lower leg injury associated with axial loading to the foot–ankle–tibia complex. Therefore, the objective of the present study is to conduct a meta-analysis using published specimen-specific data to derive injury probability/risk curves using survival analysis techniques, evaluate force magnitudes associated with different risk levels for different human age groups, and derive injury risk curves applicable to mid-size male and small-size female automotive frontal impact “dummy age” and anthropometrics.
Methods
Information was gathered from axial loading studies that reported injuries to the foot–ankle–leg complex; that is, calcaneus and/or distal tibia fractures with and without joint involvements. Studies conducted by Begeman and Aekbote (Citation1996), Yoganandan et al. (1996), Kitagawa et al. (Citation1998), Funk et al. (Citation2002), and Mackay and Bir (2009) were considered for the analysis. The reader is referred to original papers for details of experiments and schematics of the setup. Briefly, studies reported specimen-specific information such as age, maximum axial force for sub-injury- and injury-producing tests, and injuries to components described above.
Studies conducted by Yoganandan et al. (1996; group A) used a pendulum device for impacting the prealigned below-knee intact foot–ankle–tibia–fibula complex. The proximal end of each specimen was rigidly attached to a load cell. A repeated testing protocol was used. Radiography, computed tomography scanning, and gross dissection procedures following the final test were used to identify injuries. Specimen age and peak axial load data were used in the current analysis. A similar approach was used in the Begeman and Aekbote (Citation1996) study (group B), wherein the lower legs were sectioned at mid-tibia, a pendulum device was used, and a load cell was proximally attached to gather force data. Dissection procedures were used to identify injuries to the specimen following the final test. The protocol included repeated tests on some specimens. The Kitagawa et al. (Citation1998) study (group C) used a similar setup and instrumentation as in Begeman and Aekbote (Citation1996). However, only one test was conducted on each specimen regardless of the injury outcome. The Funk et al.'s (2002) study (group D) used the same single loading protocol to each specimen, resulting in injuries or no injury. Loads were delivered using a compound pendulum or a pneumatic impactor and forces were recorded by a load cell implanted into the shaft of the tibia. Mackay and Bir (2009; group E) used a linear impactor to apply dynamic loading to the dorsal surface of the foot and implanted a load cell at the mid-shaft of the tibia, similar to the previously cited study, to record the transmitted forces. Further details of end constraints used in these studies are provided in the Discussion section.
For survival analysis, specimen age and load cell data on an individual test basis were obtained along with the outcome of the experiment: whether the specimen sustained injury or remained intact. Analysis of injury forces indicated that the groups D and E were different (P <.050) from others. They were not considered for further analysis. The maximum force recorded by the load cell was considered as the primary variable and age was treated as the covariate. The applied censoring scheme was as follows. If the specimen was subjected to only one impact test, force was considered right censored if the outcome was associated with no injury and was considered left censored if injury was the outcome. If multiple tests were conducted on the same specimen, data were considered right censored for noninjury tests that produced the greatest force and left censored for tests that resulted in injury to the foot–ankle complex. In other words, an interval censoring scheme was adopted for these specimens in the survival analysis. The parameters of the parametric survival analysis were estimated using the maximum likelihood approach. Weibull, log-normal, normal, and log-logistic distributions, routinely used in impact biomechanics literature, were considered in the present analysis (Funk et al. Citation2002; Kuppa et al. Citation2003; McKay and Bir Citation2009; Pintar et al. Citation1997; Yoganandan et al. Citation2013; Yoganandan et al. Citation1996; Yoganandan et al. Citation1996). The best fit from these probability distributions was based on statistical outcomes, such as the corrected Akaike information criterion. Based on the likelihood parameter of the statistical analysis, this criterion is an indicator of parsimony of the data because it takes into account the number of variables used in the statistical model (Petitjean et al. Citation2015). The distribution with the lowest criterion represented the best-fit optimum fracture function. Plus and minus 95% confidence intervals were obtained for the selected probability distribution. The relative size of the interval was determined at predetermined risk levels. The normalized confidence interval size, the quality index, was defined as the width at a given injury probability level relative to the mean force value at the same risk. The resulting fraction was expressed as a percentage of the mean. The index was determined at 5, 10, 25, and 50% probability levels. All quality indices were determined at 25, 45, and 65 years of age.
Table 1. Summary of data from different groups of studies
The resulting PMHS-specific data were normalized using the mid-size male and small female automotive anthropometries using the equal stress equal velocity approach to derive dummy size-specific risk curves and obtain forces at discrete probability levels (Eppinger Citation1976; Yoganandan et al. Citation2014). The rationale for selecting this approach is described in the Discussion section. Normalization was accomplished using a mass of 76 kg representing the mass of the mid-size male dummy (Test device for Human Occupant Restraint [THOR] dummy and currently used Hybrid III dummy) and 49 kg representing the mass of a small-size female dummy used in frontal impact crashworthiness studies. The dummy age was set at 45 years, according to crashworthiness literature (Kuppa et al. Citation2003). Statistical analyses were conducted using R software (R Core Team 2014).
Results
The mean age for the 5 groups A, B, C, D, and E was 51.7 ± 15.9, 60.1 ± 7.1, 71.1 ± 6.7, 63.5 ± 8.5, and 66.6 ± 12.0 years, respectively. The mean total body mass for groups A, B, D, and E was 79.0 ± 13.5, 72.6 ± 16.5, 68.0 ± 13.1, and 66.5 ± 15.1 kg, respectively (). Group C did not report this variable. Out of the 114 data points in the entire ensemble from these 5 studies, 76 specimens sustained fractures of the calcaneus and or distal tibia with and without joint involvement. The other 38 tests did not result in injuries. Groups A, B, C, D, and E had 13, 7, 15, 30, and 11 fracture-producing tests and 13, 14, 1, 4, and 6 non-injury-producing tests, respectively. The mean forces in non-injury-producing tests for these groups were 4,510, 5,828, 8,152, 4,378, and 5,376 N, and mean forces in injury-producing tests were 7,821, 7,591, 7,645, 5,730, and 4,529 N, respectively. Standard deviation data are included in .
The following analysis is based on data from groups A–C (62 tests: 35 injury and 27 no injury). The Akaike information criterion of 69.46 was the lowest for the Weibull distribution and, hence, it was selected for deriving survival-based risk curves. Human injury probability curves for 25, 45, and 65 years are shown in , 2, and 3, respectively. Plus and minus 95% confidence intervals are also included in these illustrations. Forces corresponding to 25, 45, and 65 years at 5% injury probabilities were 5,425, 4,282, and 3,380 N; at 10% injury probabilities were 6,373, 5,031, and 3,971 N; at 25% injury probabilities were 7,980, 6,299 and 4,972 N; and at 50% injury probabilities were 9,715, 7,669, and 6,054 N, respectively. These data along with plus and minus 95% confidence intervals are summarized in . The quality index data at these risk levels and for all ages are summarized in . For all age groups, the indices ranged from 25.0 to 55.2% at the 50% risk level, and they showed an increasing trend regardless of age at lower probabilities. The index was in the 65–75% range for the lowest probability for all ages. It should be noted that a lower quality index indicates a tighter fit; that is, closer confidence interval. Mean risk curves are compared for all ages (Figure 4).
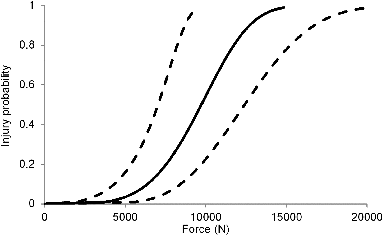
Table 2. Summary of forces (N) at discrete risk levels for different age groups
Data normalization can be accomplished using the equal stress equal velocity or impulse momentum method (Eppinger Citation1976; Mertz Citation1984; Yoganandan et al. Citation2014). Because the total body mass on a specimen-by-specimen was not available, PMHS data from group C were not used for normalizing data to represent the female and male dummy anthropometries. The mean normalizing factors were 1.035 for the male and 0.762 for the female dummy sizes. The resulting risk curve representing the 45-year-old male dummy is shown in along with plus and minus 95% confidence intervals. Forces of 3,912, 4,730, 6,164, and 7,772 N were associated with probabilities of 5, 10, 25, and 50% of lower leg injuries, respectively. For the female dummy size and at the selected 45 years of age, forces of 2,926, 3,537, 4,609, and 5,812 N were associated with probabilities of 5, 10, 25, and 50% of lower leg injuries, respectively. includes the respective confidence intervals for both dummies. The risk curves along with plus and minus 95% confidence intervals for the 45-year-old dummy age are shown in and 5b for the 50th percentile male and 5th percentile female dummy sizes.
where x represents the axial force, coefficients α and λ are statistical parameters for the Weibull distribution, and P refers to injury risk at the chosen force magnitude (Eq. 1). Values of these coefficients were as follows: a = 9.2641, 9.0281, and 8.7921 for the 25-, 45-, and 65-year-old PMHS curves and 9.0547 and 8.7642 for the mid-size male and small size female anthropometries, and λ values were 4.4643 and 3.7879 for PMHS and dummy sizes, respectively.
Table 3. Quality indices at different injury probability levels
Discussion
As indicated in the introductory paragraphs, although human lower leg injury biomechanical studies have been conducted for over 2 decades, data analyses have been largely limited to fundamental mean and standard deviation–type force magnitudes for fracture, double-censored risk curves, or survival analysis without combining data from different groups. Hence, the objective of the present study was to conduct an analysis of biomechanical data focused on axial loading to PMHS lower legs and derive injury probability curves using survival analysis. Although a recent article processed data using survival analysis, it only considered data from the 1996 publication (Yoganandan et al. Citation2014). In other words, experiments conducted by subsequent researchers were not included. It can therefore be considered that the cited study is a first attempt to process initial data using survival analysis. In contrast, the present analysis considered supplementary data with the recognition that later researchers have provided additional specimen-specific information for further processing. This was the impetus for the present research.
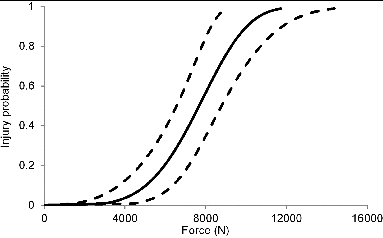
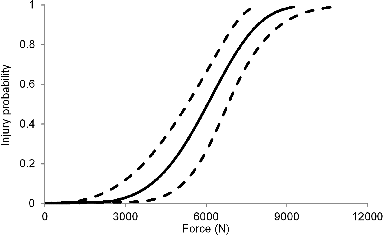
Calcaneus and/or tibia injuries, alone or in combination and with and without the involvement of the adjacent articular complexes, were included in the injury group. Hence, results from this study are applicable in general to all such injuries. However, the severity of injuries or scoring based on the Abbreviated Injury Scale and/or impairment were not considered in the present study (Association for the Advancement of Automotive Medicine 2008). The current analysis parallels other studies wherein axial loading injuries to these bony complexes are grouped, although risk curves have not been derived in all studies on an individual basis (Begeman and Aekbote Citation1996; Funk et al. Citation2002; Kitagawa et al. Citation1998; McKay and Bir Citation2009; Roberts et al. Citation1993; Seipel et al. Citation2001; Yoganandan et al. Citation1996; Yoganandan et al. Citation1997, 2000).
Constraints at the proximal end were not identical, although several groups of studies were available. In group A, the preparation was mounted on a minisled such that upon impact with the pendulum device, the specimen traveled along the rail, simulating a free-end condition. In group B, the tibia and fibula complex was potted in an alloy and fixed to a rigid loading frame via a 6-axis load cell. This resulted in a fixed-end condition. In group C, the proximal end of the tibia was fixed in an epoxy block, simulating knee entrapment with the vehicle interior in a frontal impact. The epoxy block was rigidly attached to the testing table, thus acting as immovable end condition. In group D, the prepared specimen was such that the 90° flexed knee was constrained in a block and the partial femur was tied to a bolt to minimize knee flexion. In group E, the preparation consisted of the partial femur, which was attached to a custom femur pot to interface with the Hybrid III dummy at its mid-thigh, resulting in a partially restrained condition upon impact to the dorsal surface of the foot. The influence of differing end conditions on forces and resulting injury risk curves were not investigated in the current study. Computational modeling may discern their effects and this is considered as a future study.
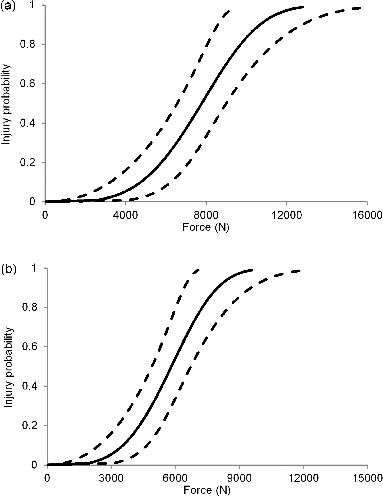
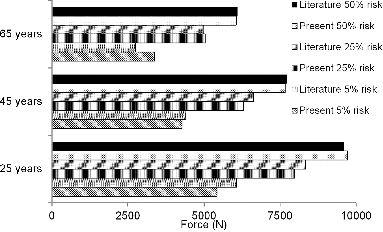
Some previous leg studies have derived probability curves based on differing approaches. For example, a logistic regression result of 8.0 kN (at 50% risk) reported in Begeman and Aekbote's (1996) study is close to the average fracture force of 7,591 N for fractured specimens. To the best of the authors’ knowledge, the previous authors did not include age as a variable in their statistical analysis. The average age was 58.5 years for their group of 7 fractured specimens. In contrast, fractured specimens used in Yoganandan et al.'s (1996) study responded with a mean force of 7,821 N and the mean age for these samples was 54.5 years. These authors developed age-based lower leg injury risk curves by assuming a distribution and treating data as doubly censored; that is, right censoring for all subinjury tests and left censoring for injured specimens. In other words, data were not treated as interval censored despite repeated tests on the same specimen. The study revealed that a force of 8.0 kN was associated with 50% risk for a 45-year-old PMHS specimen. The current survival analysis, which considered both force and age as primary and covariables, showed that the force level of 7,669 N corresponds to a 50% risk of lower leg injury at this age. Fracture forces at the mid-probability level are close to the average forces from actual experimental data from these studies.
Table 4. Forces (N) at different risk level for the 2 dummy anthropometries
It is possible to compare injury probability curves from the current survival analysis with curves presented in the literature. shows a comparison of data for all ages at 5, 25, and 50% probability levels between the present survival analysis and the data from Yoganandan et al.'s (1996) study. As can be seen, differences in the forces were generally low between the 2 methods at the 50% probability level, suggesting that a simple analysis with left and right censoring schemes may be used for leg injury prediction if the tolerance criterion is to be based at mid-level risk; that is LD50 value. However, variations in the mean values of data were larger at lower probabilities (lower tail of the curve) with the present survival analysis, and this phenomenon was found to be true for all 3 age groups (). The survival analysis is considered more accurate and better represents the underlying distribution because it accounts for interval censoring in addition to other statistical features. From this perspective, force magnitudes at lower risk levels from the present study are reliable estimates of human lower leg injury tolerance. Recent studies have indicated the need to obtain robust injury criteria for specifications at lower probability levels (Prasad et al. Citation2010). Therefore, it is important to have confidence in tolerance values at lower levels, which include the tail ends of the curve, for crashworthiness advancements. Because the present survival analysis was based on both statistical and biomechanical aspects, combined force data from different studies with the same injury mechanisms (all injured specimens sustained fractures to the calcaneus and/or tibia components with or without articular joint involvements), considered age as a covariate, and selected PMHS injuries that are identifiable/diagnosable using clinical evaluations and x-rays and computed tomography scans, current force results at lower probabilities represent improvement in injury criteria assessments for the lower leg trauma under axial loading.
The following inferences can be drawn based on the quality index parameter. It should be acknowledged that the index is a measure of data spread at the plus and minus 95% confidence interval at a given risk. As shown in , for 45 and 65 years of age and at the 50% risk level, the indices were the lowest (25–29%). However, for all ages, the index gradually increased as one progressed from 50 to 5% risk level, and the risk curve for 25 years of age responded with the greatest index ranging from 55 to 74% at the lowest risk level (). Lower index values indicate improved tightness of the fit of the confidence interval bounding the mean force curve. Looser bounds (higher magnitudes of the index) occurring for 25 years of age can be attributed to the limited number of specimens tested at younger ages in PMHS experiments. Although automotive studies and standards are generally focused on protecting adult male populations as represented by the use of the 45-year-old dummy in motor vehicle standards in the United States and elsewhere, other areas such as safety of military personnel, who are generally younger compared to the civilian motor vehicle populations, need a robust criteria at lower age groups (Kuppa et al. Citation2003; Owens et al. Citation2008; Yoganandan et al. Citation2013). The present analysis clearly indicates a need to conduct additional experiments to obtain a robust criterion to derive human tolerance and injury criteria for the younger group. From this perspective, these results might have opened new avenues to advance safety in other environments. In addition to these limitations, because both sexes are included in the current analysis and because the physiology and response of the human musculoskeletal system are sex specific, in order to fully delineate the tolerance, tests and outputs should be categorized based on sex (Pintar et al. Citation1998; Stemper et al. Citation2003).
The normalization was done using the equal stress equal velocity approach, which considers mass as the sole variable (Eppinger Citation1976). Approaches such as the impulse momentum technique use additional parameters (Mertz Citation1984; Yoganandan et al. Citation2014). They were not used in this analysis due to a lack of data such as impulse and characteristic lengths or deformations of each PMHS. For the mid-size male and small female anthropometries, data from both sexes were combined, representing an initial step for the derivation of injury reference values (IRVs) for the 2 dummies. This approach of combining data from both sexes has also been used to determine response corridors for males and females in side impacts (Kuppa et al. Citation2003; Maltese et al. Citation2002; Yoganandan and Pintar Citation2005). The respective injury risk curves for these anthropometries ( and 5b) and forces associated at specific probabilities () can be used as a first step as an indicator of IRV for automotive crash test devices, although data from group C were excluded because of lack of mass information. Once additional and sex-specific tests are conducted and data are gathered to use the impulse momentum approach for normalization, it will be possible to derive more robust curves and redefine IRV.
It should be noted that the dummy leg is stiffer than the human leg because it is infrangible. The increased stiffness should be taken into account while developing actual risk curves for dummies. To achieve this aim, matched-pair tests can be conducted under identical inputs using 2 types of surrogates: the biological surrogate (PMHS) acting as a model that produces injury and noninjury data points and the dummy surrogate (Hybrid III, for example) producing peak forces corresponding to injury and noninjury data. Using dummy-produced peak forces and biological surrogate–produced injury outcomes, risk curves can be derived for specific dummies. The stiff nature of the dummy results in increased forces compared to the human cadaver surrogate. In other words, the transformation function/ratio for the physical surrogate exceeds unity. Although not specific to the current data set, the transformation ratio can reach up to 3 times the PMHS force depending on loading conditions. This needs further research.
The present study selected PMHS leg injury studies published in the literature that provided specimen-specific data such as dynamic peak force, age, total body mass, and injuries for survival analyses. Calcaneus and/or tibia injuries, alone or in combination and with and without the involvement of the adjacent articular complexes, were included in the injury group. These injuries are more likely to lead to long-term disability, impairment, and higher societal costs. Isolated talus injuries were not found in this group, although fractures of the talus occurred in association with other trauma. In order to develop risk curves for this bone, targeted experimental data are needed, and this would be a future study topic. Injury and noninjury tests were included and censoring was based on the number of tests on each specimen and its injury outcomes after each test. The Weibull distribution was effective to describe the risk curves. Human injury probability curves were presented at 25, 45, and 65 years along with plus and minus 95% confidence intervals. Risk curves were also derived for applications to mid-size male and small-size female dummy sizes using normalized forces and assuming the dummy age to be 45 years. Quality indices increased (less tightness of fit) with decreasing age and risk level for all age groups and for both dummy sizes, suggesting the need to conduct additional tests to improve the robustness of the probability curves at their left tail ends. These risk curves at different age groups based on data from different groups of researchers constituting the largest body of data can be used as initial human tolerances to lower leg injury from axial loading. The anthropometry-specific mid-size male and small-size female mean risk curves along with plus and minus 95% confidence intervals from the survival analysis and associated IRV data at discrete probability levels can be used as a first step in studies aimed at advancing occupant safety in motor vehicle and other environments.
Funding
This material is the result of work supported with resources and the use of facilities at the Zablocki VA Medical Center, Milwaukee, Wisconsin, and the Medical College of Wisconsin. Narayan Yoganandan, Mike Arun, and Frank Pintar are part-time employees of the Zablocki VA Medical Center, Milwaukee, Wisconsin. Any views expressed in this article are those of the authors and not necessarily representative of the funding organizations.
References
- Association for the Advancement of Automotive Medicine. The Abbreviated Injury Scale. Des Plaines, IL: Author; 2008.
- Austin RA. Lower Extremity Injuries and Intrusion in Frontal Crashes. Washington, DC: National Center for Statistics and Analysis, NHTSA; 2012.
- Begeman PC, Aekbote K. Axial load strength and some ligament properties of the ankle joint. In: Grimm MJ, ed. 6th Injury Prevention Through Biomechanics Symposium. Detroit, MI: Wayne State University; 1996:125–135.
- Burgess AR, Dischinger PC, O’Quinn TD, Schmidhauser CB. Lower extremity injuries in drivers of airbag-equipped automobiles: clinical and crash reconstruction correlations. J Trauma. 1995;38:509–516.
- Dischinger PC, Burgess AR, Cushing BM, et al. Lower Extremity Trauma in Vehicular Front-seat Occupants: Patients Admitted to a Level I Trauma Center. Warrendale, PA: Society of Automotive Engineers; 1994.
- Dischinger PC, Read KM, Kufera JA, et al. Consequences and costs of lower extremity injuries. Annu Proc Assoc Adv Automot Med. 2004;48:339–353.
- Eigen A, Glassbrenner D. The Relationship Between Occupant Compartment Deformation and Occupant Injury. Washington, DC: NHTSA; 2003. Report No. DOT HS 809 676.
- Eppinger R. Prediction of thoracic injury using measurable experimental parameters. In: Proceedings of the International Conference of Experimental Safety Vehicles, Washington, DC, June 5–9, 1976. Washington, DC: NHTSA; 1976;770–779.
- Estrada LS, Alonso JE, McGwin GJr, Metzger J, Rue LW III. Restraint use and lower extremity fractures in frontal motor vehicle collisions. J Trauma. 2004;57:323–328.
- Fildes B, Lenard J, Lane J, Seyer K. Lower limb injury in frontal crashes. Paper presented at: International Conference on Pelvic and Lower Extremity Injuries; 1995; Washington, DC.
- FMVSS-208. 2000. Code of Federal Regulations, Part 572.208.
- Funk JR, Crandall JR, Tourret LJ, et al. The axial injury tolerance of the human foot/ankle complex and the effect of Achilles tension. J Biomech Eng. 2002;124:750–757.
- Jibril A, Prasad P, Prybylski J, Parekh I, Grush ES. Logistic regression analysis of lower limb injuries in frontal crashes. Paper presented at: 16th International Technical Conference on the Enhanced Safety of Vehicles; 1998; Ontario, Canada.
- Kitagawa Y, Ichikawa H, King AI, Levine RS. A severe ankle and foot injury in frontal crashes and its mechanism. Paper presented at 42nd Stapp Car Crash Conference, Tempe, AZ, November 2–4, 1988. Warrendale, PA: Society of Automotive Engineers; 1998.
- Kuppa S, Eppinger RH, McKoy F, Nguyen T, Pintar FA, Yoganandan N. Development of side impact thoracic injury criteria and their application to the modified ES-2 dummy with rib extensions (ES-2re). Stapp Car Crash J. 2003;47:189–210.
- Kuppa S, Sieveka EM. Dynamic motion of the floor pan and axial loading through the feet in frontal crash tests. Paper presented at: International IRCOBI Conference on the Biomechanics of Impact; 1995; Brunnen, Switzerland.
- Lestina DC, Kuhlmann TP, Keats TE, Alley RM. 1992. Mechanisms of fracture in ankle and foot injuries to drivers in motor vehicle crashes. Paper presented at 36th Stapp Car Crash Conference, Seattle, WA, November 2–4, 1992. Warrendale, PA: Society of Automotive Engineers; 16:59–68.
- Maltese MR, Eppinger RH, Rhule HH, Donnelly BR, Pintar FA, Yoganandan N. Response corridors of human surrogates in lateral impacts. Stapp Car Crash J. 2002;46:321–351.
- McKay BJ, Bir CA. Lower extremity injury criteria for evaluating military vehicle occupant injury in underbelly blast events. Stapp Car Crash J. 2009;53:229–249.
- Mertz HJ. A Procedure for Normalizing Impact Response Data. Warrendale, PA: Society of Automotive Engineers; 1984.
- Morgan RM, Eppinger RH, Hennessey BC. Ankle joint injury mechanism for adults in frontal automotive impact. Paper presented at 35th Stapp Car Crash Conference, San Diego, CA, November 18–20, 1991. Warrendale, PA: Society of Automotive Engineers; 1991:189–198.
- Owens BD, Kragh JF Jr, Wenke JC, Macaitis J, Wade CE, Holcomb JB. Combat wounds in operation Iraqi Freedom and operation Enduring Freedom. J Trauma. 2008;64:295–299.
- Pattimore D, Ward E, Thomas P, Bradford M. The nature and cause of lower limb injuries in car crashes. Paper presented at: Stapp Car Crash Conference; 1991; San Diego, CA.
- Petitjean A, Torselli X, Yoganandan N, Pintar FA. Normalization and scaling for human response corridors and development of injury risk curves. In: Yoganandan N, Nahum AM, Melvin JW, eds. Accidental Injury: Biomechanics and Prevention. 3rd ed. New York, NY: Springer; 2015:769–792.
- Pintar FA, Yoganandan N, Hines MH, et al. Chestband analysis of human tolerance to side impact. Paper presented at: Stapp Car Crash Conference; 1997; Lake Buena Vista, FL.
- Pintar FA, Yoganandan N, Voo L. Effect of age and loading rate on human cervical spine injury threshold. Spine (Phila Pa 1976). 1998;23:1957–1962.
- Prasad P, Mertz HJ, Dalmotas DJ, Augenstein JS, Diggs K. Evaluation of the field relevance of several injury risk functions. Stapp Car Crash J. 2010;54:49–72.
- R CoreTeam. R: A Language and Environment for Statistical Computing. Vienna, Austria: R Foundation for Statistical Computing; 2014.
- Roberts D, Donnelly B, Severin C, Medige J. Injury Mechanisms and Tolerance of the Human Ankle Joint. Atlanta, GA: Centers for Disease Control; 1993.
- Rudd RW. Updated Analysis of Lower Extremity Injury Risk in Frontal Crashes in the United States. Paper presented at: 21st International Technical Conference on the Enhanced Safety of Vehicles; 2009; Stuttgart, Germany.
- Seipel RC, Pintar FA, Yoganandan N, Boynton MD. Biomechanics of calcaneal fractures: a model for the motor vehicle. Clin Orthop Relat Res. 2001;388:218–224.
- States JD. Adult occupant injuries of the lower limb. Paper presented at: Symposium on Biomechanics and Medical Aspects of Lower Limb Injuries; 1986; San Diego, CA.
- Stemper BD, Yoganandan N, Pintar FA. Gender dependent cervical spine segmental kinematics during whiplash. J Biomech. 2003;36:1281–1289.
- Yoganandan N, Arun MW, Pintar FA. Normalizing and scaling of data to derive human response corridors from impact tests. J Biomech. 2014;47:1749–1756.
- Yoganandan N, Arun MW, Pintar FA, Szabo A. Optimized lower leg injury probability curves from postmortem human subject tests under axial impacts. Traffic Inj Prev. 2014S151–S156.
- Yoganandan N, Arun MW, Stemper BD, Pintar FA, Maiman DJ. Biomechanics of human thoracolumbar spinal column trauma from vertical impact loading. Ann Adv Automot Med. 2013;57:155–166.
- Yoganandan N, Haffner M, Pintar FA. Hampson D: Facial injury: a review of biomechanical studies and test procedures for facial injury assessment. J Biomech. 1996;29:985–986.
- Yoganandan N, Pintar FA. Deflection, acceleration, and force corridors for small females in side impacts. Traffic Inj Prev. 2005;6:379–386.
- Yoganandan N, Pintar FA, Boynton M, et al. Dynamic axial tolerance of the human foot–ankle complex. Paper presented at 40th Stapp Car Crash Conference, New Mexico, November 4–6, 1996. Warrendale, PA: Society of Automotive Engineers; 1996:207–218.
- Yoganandan N, Pintar FA, Kumaresan S, Boynton M. Axial impact biomechanics of the human foot–ankle complex. J Biomech Eng. 1997;119:433–437.
- Yoganandan N, Pintar FA, Seipel R. Experimental production of extra- and intra-articular fractures of the os calcis. J Biomech. 2000;33:745–749.
- Yoganandan N, Stemper BD, Pintar FA, Maiman DJ, McEntire BJ, Chancey VC. Cervical spine injury biomechanics: applications for under body blast loadings in military environments. Clin Biomech (Bristol, Avon). 2013;28:602–609.