ABSTRACT
In order to overcome the disadvantage of highly flammable and poor mechanical performance of cellulose aerogel, herein, cellulose nanofibrils (CNF) were cross-linked by melamine formaldehyde (MF), and further cross-linked and surface decorated by methyltrimethoxysilane (MTMS) to obtain flame retarded compound aerogels. FT-IR spectra confirmed that cross-linking reaction occurred between MF/MTMS and CNF matrix. The morphological analysis demonstrated that the pore size shrinked, whereas the pore amount of compound aerogels increased after cross-linking, thereby resulting in the improvement of mechanical performance. Via double cross-linking, the compound aerogels also exhibited excellent hydrophobicity (contact angle up to 132.3°) and flame retardant properties. The limited oxygen index (LOI) value of double cross-linked specimen (Si-CNF/MF) increased from 19.5% to 37.1%, and the vertical combustion test (UL-94) reached V-0 grade. Microcalorimetry measurement showed that the peak heat release rate (pHRR) and total heat release (THR) of Si-CNF/MF decreased by 50.6% and 64.3% in comparison with pure CNF aerogel, respectively. The analysis results of char residue showed that both condensed phase and gas phase flame retardant mechanisms occurred during combustion, and synergistic effect existed between MF and MTMS. Moreover, the very low thermal conductivity of compound aerogels permitted their application as heat preservation materials.
摘要
为了克服纤维素气凝胶高度易燃和力学性能差的缺点,本文采用三聚氰胺-甲醛(MF)交联纤维素纳米纤维(CNF),然后用甲基三甲氧基硅烷(MTMS)进一步交联和表面修饰,得到阻燃复合气凝胶. 红外光谱证实,MF/MTMS和CNF基质之间发生了交联反应. 形态分析表明,交联后复合气凝胶的孔径缩小,而孔量增加,从而导致力学性能的提高. 通过双重交联,复合气凝胶还表现出优异的疏水性(接触角高达132.3°)和阻燃性能. 双交联试样(Si-CNF/MF)的极限氧指数(LOI)值从19.5%增加到37.1%,垂直燃烧试验(UL-94)达到V-0级. 微量热测定结果表明,Si-CNF/MF的峰值热释放速率(pHRR)和总热释放量(THR)分别比纯CNF气凝胶降低50.6%和64.3%. 对残炭分析结果表明,燃烧过程中既有凝聚相阻燃机制,也有气相阻燃机制; MF和MTMS之间存在协同效应. 此外,复合气凝胶的极低导热性允许其作为保温材料应用.
Introduction
Cellulose is the largest renewable resource in the world. Due to the characteristics such as biodegradability, low cost and renewability, cellulose-based materials have become a hot research field in biomass materials (Siqueira, Bras, and Dufresne Citation2010, Mokhena et al. Citation2020). As the third generation of novel aerogels after silica aerogels and polymer-based aerogels, cellulose aerogel exhibits many advantages, such as good biocompatibility, reproducibility and degradability (Rahimi et al. Citation2017). At present, the application of cellulose aerogel has been extended to many fields, such as sewage treatment, green energy storage, biomedicine and aerospace, indicating its great development potential (Hamedi et al. Citation2013; Sai et al. Citation2015; Valo et al. Citation2013). However, as a natural polymer material, cellulose exhibits very low LOI value and high heat release capacity, thus resulting in the widespread fire safety hazards for cellulose-based products (Nabipour et al. Citation2020). Simultaneously, as a three-dimensional nanoporous material, the internal structure of cellulose aerogel is mainly formed by hydrogen bonding and physical entanglement between cellulose molecular chains (Seddiqi et al. Citation2021). The low density and high porosity of cellulose aerogel determine its relatively low mechanical performance (Fischer et al. Citation2006). Thus, the flammability and poor mechanical performance of cellulose aerogel limits its practical application.
Many researches have focused on the flame retardant modification of cellulose aerogels. Among them, the incorporation of inorganic flame retardants is an efficient method. Fan et al (Fan et al. Citation2017) prepared cellulose nanofibrils (CNF)/boehmite (AlOOH) compound aerogels via hydrothermal method. The well dispersion of spherical AlOOH particles with an average diameter of 0.5 μm significantly improved the flame retardant properties of compound aerogel. Moreover, many inorganic additives with nanoscale, such as graphene oxide (Wicklein et al. Citation2015) and 1T phase molybdenum disulfide (MoS2) (Yang et al. Citation2017), can improve the flame retardant properties of compound aerogels more efficiently. Another approach is to introduce flame retardant elements or groups into cellulose molecular chains via chemical reactions. For example, flame retarded cellulose aerogel with excellent elasticity and self-extinguishment could be fabricated via the reaction between CNF and N-methylol-dimethphospylpropionamide (MDPA), and further cross-linked by 1,2,3,4-butane tracarboxylic acid (BTCA) (Guo et al. Citation2018). Moreover, the flame-resistant properties of cellulose aerogel can be further enhanced by the combination of chemical decoration and inorganic flame retardants. Using cellulose nanofibrils prepared from phosphorylated pulp fibers (P-CNF) and microfibrous sepiolite clay, the resultant nanostructured cellulose aerogels showed excellent flame-retardant properties such as self-extinguishing behavior and extremely low heat release rates (Ghanadpour et al. Citation2018). As mentioned above, the flame retardant properties of cellulose aerogels can be improved by various methods. However, the comprehensive properties of cellulose aerogel should also be considered in order to apply it in more fields. For example, aerogels with high hydrophobicity can be applied to oil-water separation, and aerogels with high thermal insulation properties can be used in thermal insulation materials (Yang et al. Citation2022). Thus, more modification approaches for cellulose aerogels based on its comprehensive properties, including mechanical performance, thermal insulation and hydrophobic properties, et al., should be explored and investigated.
Melamine-formaldehyde (MF) resin is a nitrogen-containing flame retardant with high efficiency, and it can react with the hydroxyl groups on the surface of cellulose to form crosslinking structure (Nishida et al. Citation2019), thus improving the flame retardant properties and mechanical performance of cellulose aerogels. Besides, as a commonly used surface modification method, silylation can not only improve the flame retardancy of aerogels, but also endow them with hydrophobicity in a certain degree (Kim, Youn, and Song Citation2018). Thus, herein, MF and Methyl trimethoxysilane (MTMS) were used as flame retardant and synergistic flame retardant, respectively, to prepare CNF aerogel via a simple crosslinking reaction in this paper. The process flow is shown in . The mechanical performance, hydrophobic property, thermal properties and flame retardancy of obtained CNF aerogels were investigated. Moreover, the chemical structure and microstructure of aerogel specimens were investigated by FT-IR and SEM. Furthermore, the combustion parameters were analyzed by micro combustion calorimetry (MCC) in order that we can obtain some information from the view of flame retardant mechanism.
Experimental
Materials
Cellulose nanofibrils suspension (solid content was 1 wt%) were purchased from Guilin Qihong Technology Co., Ltd. Methyl trimethoxysilane (MTMS, Analytical Reagent), melamine (Analytical Reagent), Sodium hydroxide (Analytical Reagent), Formaldehyde (37.0%), hydrochloric acid (36.0–38.0%) and potassium bromide (spectroscopic grade), were purchased from Tianjin Damao Chemical Reagent Factory.
Fabrication of compound aerogels
Preparation of MF precursor solution
5.0 g of melamine and 10.0 g formaldehyde solution (37 wt%) were mixed using a magnetic agitator at 85℃ until the melamine was completely dissolved in formaldehyde solution. And then, the pH value was adjusted to about 9.0 by 0.05 M NaOH solution dropwise, reacting for 30 min under stirring until the solution was transparent. The resulting transparent solution is the MF precursor solution.
Preparation of compound aerogels
MF precursor solution was added into CNF suspension, and stirring with high-speed shear emulsifier (FJ200-S, Shanghai specimen Instrument Co., Ltd, China) under 1500 r/min for 5 hours to make it uniformly dispersed. And then, the pH value of suspension was adjusted to 4.0 using 0.1 M hydrochloric acid solution. Subsequently, a certain amount of MTMS was added to suspension and stirred at high speed for 2 hours to guarantee the reaction between MTMS and CNF. Finally, the obtained suspension was poured into the mold and prefrozen in cold trap, and freeze-dried for 48 hours. Finally, the compound aerogels could be obtained.
The components of all prepared aerogel specimens are shown in . The specimen containing without any additives but only pure CNF was prepared as a control specimen.
Table 1. Components of compound aerogel specimens.
Characterizations
The dried specimens were mixed with potassium bromide and pressed into tablets. Then analysis was performed by the FT-IR (VERTEX-70, Bruker, Germany) in the range of 4000–400 cm−1 with a resolution of 4 cm−1 and a scan number of 32 times. According to the frequency change of the characteristic absorption band, the changes of the groups and bonds of the composite aerogel were observed, and then the chemical structure was analyzed.
The morphology of specimens before or after combustion was observed using a HITACHI S-4800 SEM (Hitachi, Tokyo, Japan) apparatus at 5.0 kV accelerating voltage. The elemental components of specimens were measured by energy dispersive X-ray spectroscopy (EDX) equipped in SEM apparatus. Before measurement, the dried specimens were dispersed on double-sided conductive tape and sprayed with gold in vacuum. It was used to characterize the changes in surface morphology and three-dimensional structure of aerogels before and after modification.
The compression properties of specimen were measured using a universal testing machine (AI-7000-NGD, High Speed Railway Testing Instrument Co., Ltd, Taiwan, China) at a compression speed of 5 mm min−1, and compressed into the height equivalent to 60% of original specimen. Quintuplet specimens for mechanical performance were tested.
The hydrophobicity of compound aerogels was measured using an optical contact angle instrument (CAM200, KSV Co., Ltd, Finland) under 23℃ and 53% RH.
The thermal conductivity of dried specimens was measured according to ISO 22,007-2:2008 using a thermal constant analyzer (TPS2200, Kegonas Co., Ltd, Sweden). Quintuplet specimens were tested.
The apparent density of dried specimens was calculated from the mass and volume of specimen. Triplet specimens were measured and calculated.
Thermogravimetric (TG) curves were recorded by a STA 449F3 thermal analyzer (Netzsch, Germany) at a temperature range of 30℃-700℃ using a heating rate of 10 ℃/min under nitrogen atmosphere of 30 mL min−1. Characterization of thermal stability of composite aerogels according to the change process of composite aerogel mass with temperature.
The limited oxygen index (LOI) tests were measured according to GB/T2406–2009 on a HC-2C oxygen index meter (Shangyuan Testing Equipment Co., Ltd, Jiangsu Province, China). The UL-94 vertical burning tests were measured according to GB/T2408–2008 on a vertical burning apparatus (Tech-GBT2048–002, Taisi Testing Instrument Technology Co., Ltd, Jiangsu Province, China). The specimens used for the combustion tests were as follows: diameter, 10 mm; length 100 mm. Quintuplet specimens were tested.
Micro calorimeter test was conducted on a FTT0001 micro calorimeter (Fire Testing Technology Co., Ltd, U.K.) according to ASTM D7309–2007. 2–10 mg of aerogel specimens was measured at a temperature range from 75℃ to 700℃ using a heating rate of 5℃/min under nitrogen atmosphere of 80 mL min−1. Various parameters, including heat release rate (HRR), total heat release (THR), peak of heat release rate (pHRR), were determined and recorded. The microcalorimeter can not only visually and intuitively test the changes of the flame retardant properties of composite aerogels, but also quantitatively analyze these changes.
Results and discussion
Morphological characterization
The SEM images of pure and compound CNF aerogels are shown in . It can be found that all the CNF aerogels showed three-dimensional honeycomb pore structure, which was caused by the squeezing effect of ice crystals formed during freezing process. Simultaneously, as can be seen from , pure CNF aerogel exhibited larger inner cavity structure than those of MF/MTMS cross-linked aerogels, can be attributed to the cross-linking reaction between MF/MTMS and the hydroxyl groups on the surface of CNF matrix. Moreover, it can be seen from that the aerogels modified by MTMS exhibited more uniform surface void size distribution, which can be attributed to the substitution reaction between silane coupling agent and the hydroxyl groups on the surface of CNF matrix. When CNF matrix was cross-linked by MF, the pore structure of compound aerogels became denser in comparison with that of pure CNF aerogel []. The morphological characteristics of compound aerogels proved the chemical reaction between MF/MTMS and CNF matrix, which was consistent with the results of IR spectra in the next part. Besides, it can be found that the compound aerogels with the incorporation of MF and MTMS exhibited shrinked size and increased number, whereas the irregular pore structure compared with other specimens, as can be seen from . This may be attributed to the relative high crosslinking degree and hydrophobicity of compound aerogels caused by the synchronous addition of MF and MTMS, which hindered the growth of ice crystals during freezing process, and resulting in the irregular morphology of aerogel pores.
IR analysis
plots the infrared spectra of pure and compound CNF aerogels. The characteristic peaks of pure CNF aerogels were located at 3427 cm−1, 1606 cm−1, 1120 cm−1 and 895 cm−1, which corresponded to the stretching vibration peaks of hydrogen associative hydroxyl groups, -CHO, C-O-C and beta-glycoside bonds between sugar units, respectively (Elanthikkal et al. Citation2010; Nelson and O’Connor Citation1964). Additionally, two characteristic peaks at 2934 cm−1 and 1425 cm−1 were associated with the stretching and shearing vibration of asymmetric -CH2-, respectively (Zhong et al. Citation2016). With the incorporation of MTMS, an absorption peak at 1274 cm−1 which represents the stretching vibration of Si-C groups could be observed in the spectra of Si-CNF and Si-CNF/MF aerogels. Moreover, significant flexural vibration peaks of Si-O-Si at 780 cm−1 could be also observed in these two spectra. The appearance of these peaks indicated that MTMS has been successfully grafted onto the skeleton structure of cellulose. Meanwhile, the characteristic peaks at 1530 cm−1 and 816 cm−1 related to 1,3,5-triazine ring structure of MF and its characteristic stretching vibration, respectively, which indicated that the carboxylic acid groups of CNF reacted with the amine groups of MF to form amide bond (Wu et al. Citation2013). Besides, the flexural vibration peak of -OH shifted from 3427 cm−1 to 3390 cm−1. This was attributed to the reduction of hydroxyl groups on the surface of CNF which caused by the replacement of free water by bound water molecules during the preparing process of CNF aerogel. This also further indicated the reaction between MF/MTMS and CNF.
Mechanical performance
plots the stress-strain curves of pure and compound CNF aerogels. All the specimens exhibited typical elastic foaming behavior. Test results for mechanical performance show that all the compound aerogels exhibited higher compressive strength, compression modulus and specific modulus compared with pure CNF aerogels. The compressive modulus and specific modulus of compound aerogels incorporated with MF (CNF/MF) increased to 15.27 KPa and 1885.19 KPa/g cm−3, which were about 2.0 and 1.5 times than that of pure CNF aerogels, respectively. This might be attributed to the relative stable network structure of compound aerogel cause by the crosslinking reaction between MF and CNF matrix. Besides, the compression modulus (13.68 KPa) and specific modulus (1537.08 KPa/g cm−3) of compound aerogel with the incorporation of MTMS (Si-CNF) were also significantly higher than those of pure CNF aerogels. This is attributed to the strengthened cellulose skeleton structure of compound aerogel which caused the attachment of MTMS on the surface of CNF. Moreover, the compound aerogel synchronous modified by MTMS and MF (Si-CNF/MF) exhibited better mechanical performance than those of aerogels incorporated with individual MTMS or MF. The compression modulus and specific modulus of Si-CNF/MF increased to 22.91 KPa and 2268.32 KPa/g cm−3, equivalenting 3.0 and 1.8 times as those of pure CNF aerogel, respectively.
Contact angle
The hydrophobic properties of pure and compound CNF aerogels were characterized by contact angle measurement, and the corresponding images are shown in . As can be seen from , water droplets were instantly absorbed on the surface of pure CNF aerogel, which was attributed to the natural hydrophilicity of CNF (Joly, Gauthier, and Escoubes Citation1996). After treated by MTMS, the contact angle between Si-CNF and water increased to 126.2°, as shown in . This demonstrated that the surface of Si-CNF was converted from hydrophilic to hydrophobic via the modification of hydrophobic silane coupling agent. Additionally, the contact angle between CNF/MF and water also exhibited significant increase compared with that of pure CNF, i.e., 85.7 ° []. As a thermoset resin with hydrophobicity in a certain degree, the introduction of MF, as well as the increase of crosslinking degree for CNF matrix, obviously improved the hydrophobicity of compound aerogel. Furthermore, Si-CNF/MF exhibited the highest contact angle among all the aerogel specimens, i.e., 132.3 °, as shown in . It indicated that better hydrophobicity of compound aerogels could be realized via combined treatment, i.e., double cross-linking and decoration. Simultaneously, the remarkable enhancement of hydrophobicity could expand the utilization fields of obtained compound aerogels.
Flame retardant properties
lists the LOI and UL-94 results of pure and compound CNF aerogels. As can be seen from , the LOI value of pure CNF aerogel was only 19.5%, and the UL-94 grade was NR, indicating that pure CNF aerogel was highly flammable. When MF and/or MTMS were incorporated, the flame retardant properties of compound aerogels were significant improved. Typically, the LOI value of CNF/MF-2 increased to 28.5% only by the addition of 0.25 g MF. Possible reason is that MF is a gas phase flame retardant, a mass of nonflammable gas could be produced, and diluting the flammable gas during combustion process. However, the UL-94 grade of CNF/MF still maintained at NR, and only UL-94 V1 grade could be achieved by higher MF loading (CNF/MF-1). Besides, the LOI value of aerogels modified by MTMS (Si-CNF and Si-CNF-1) reached 30.4% and 33.7%, respectively, and the UL-94 grade of Si-CNF promoted to V1 level even under a relatively low MTMS loading. The flame resistance of MTMS mainly manifests as condensed flame retardant mechanism, which can also be proved by the char residue amount obtained by TG analysis (). It seems that MTMS exhibited higher flame retardant efficiency than MF. Furthermore, when MF and MTMS were synchronous added into CNF matrix, the flame retardant properties of compound aerogel exhibited significant improvement. The LOI value and UL-94 grade of Si-CNF/MF reached 37.1% and V0 level, respectively, which indicated that a synergistic effect might be existed between MF and MTMS.
Table 2. LOI and UL-94 test results of pure and compound CNF aerogels.
Table 3. Thermogravimetric data, thermal conductivity and apparent density of pure and compound CNF aerogels.
Thermal stability
TG and DTG curves of pure and compound CNF aerogels are shown in . The relevant parameters are listed in . As can be seen from and , the initial decomposition temperature (T5 wt%) occurred below 200 ℃, which might be caused by the weight loss of bound water and volatiles with small molecule. Moreover, a more significant weight loss occurred in the temperature range from 200℃ to 400℃, corresponding to the main thermal degradation phase. Pure CNF aerogel exhibited relative low thermal stability, the T5 wt% was only 59.3℃, and the temperature in maximum thermal decomposition rate (Tmax) was 303.8℃. Compared with pure CNF aerogel, both T5 wt% and Tmax of compound aerogels exhibited significant improvement. Typically, the T5 wt% of Si-CNF increased from 59.3℃ to 181.4℃, and the Tmax of CNF/MF increased from 303.8℃ to 328.1℃. The main reason is that the skeleton structure of pure CNF aerogel was combined via hydrogen bonding, whereas the skeleton structures of compound aerogels were cross-linked via covalent bonding, and to form a more stable network structure. Furthermore, the maximum thermal decomposition rates of compound aerogels are significantly lower than that of pure CNF aerogel. Especially, Si-CNF/MF exhibited the lowest maximum thermal decomposition rate, as can be seen from . This demonstrated that the thermal stability of compound aerogels is effective improved by crosslinking and decoration. Besides, the carbon residues at 700℃ of Si-CNF and CNF/MF increased from 18.53% for pure CNF to 53.84 wt% and 37.84 wt%, respectively, which indicated that both MTMS and MF could effectively promote the carbonization capacity of CNF matrix. The significant increase of carbon residue also favored the improvement of flame retardant properties. Additionally, the carbon residue of Si-CNF/MF (46.75 wt%) was lower than that of Si-CNF (53.84 wt%). This is mainly due to the loss of volatile components which decomposed by MF and the oxidation of some relative stable structures during combustion, and resulting in the decrease of carbon residue. (Nguyen et al. Citation2014).
Carbon residue analysis
The microstructure of char residue can provide some information about the flame retardant mechanism during combustion process. The SEM images of char residues after combustion for pure and compound CNF aerogels are shown in . As can be seen in , the three-dimensional pore structure of pure CNF aerogel was completely destroyed after combustion. Cellulose is a highly flammable materials. Additionally, due to the light and polyporous structure, the external oxygen and the combustible gases generated during combustion process could be rapid diffused, further accelerated the combustion rate, and resulted in a large amount of irregular char residue. When MTMS was incorporated into compound aerogel, an integral and relative compact carbon layer after combustion for Si-CNF can be observed, as shown in . This integral carbon layer could hinder the propagation of oxygen and heat during combustion process, as well as the oxidative pyrolysis of CNF aerogel matrix. As mentioned above, Si-CNF exhibited the highest char residue at 700℃. Thus, the improvement of flame retardant properties for Si-CNF can be attributed to the condensed flame retardant mechanism. is the image of char residue for CNF/MF, a large number of bulges can be observed on the surface of carbon layer. This was caused by the nonflammable gas ammonia generated by MF, which could dilute the flammable gas and oxygen during combustion. This can also explain the relative low char residue of CNF/MF in comparison with that of Si-CNF. With the synchronous addition of MF and MTMS, Si-CNF/MF also maintained an integral and relative compact carbon layer similar to Si-CNF after combustion, as well as many bulges similar to CNF/MF, as can be seen from . Therefore, it is reasonable to be inferred that the improvement of flame retardant properties for Si-CNF/MF was derived from both condensed phase and gas phase flame retardant mechanism.
Figure 5. SEM images of carbon residue, elemental distribution and FT-IR spectra of pure and compound CNF aerogels after combustion.
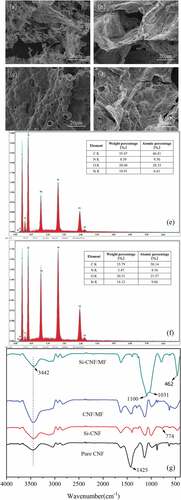
The element components of Si-CNF/MF before and after combustion were characterized by EDS. The results are shown in . The C atomic percentage of Si-CNF/MF increased slightly after combustion, which might due to the degradation of cellulose skeleton (Jiang et al. Citation2017). Simultaneously, the O atomic percentage of Si-CNF/MF decreased from 28.60 wt% to 20.51 wt% after combustion, which might be caused by the generation of noninflammable gases (H2O, CO2, et al.) during combustion. Additionally, C atomic percentage decreased from 8.39 wt% to 3.47 wt%. This could be attributed to the generation of volatile gas ammonia. This result is consistent with the analysis results of TGA and SEM images for carbon layer. Besides, The Si atomic percentage increased from 10.91 wt% to 16.12 wt% after combustion, which further proved the condensed phase flame retardant mechanism of MTMS.
plots the FT-IR spectra of char residues of pure and compound CNF aerogels after combustion. As shown in , the strong absorption near 3442 cm−1 is attributed to the stretching vibration of -NH2 and -OH groups (Saake et al. Citation2005). The absorption intensity of this peak for CNF/MF is obviously stronger than those of pure CNF and Si-CNF which was due to the coincidence of -NH2 and -OH bonds. The bending band at 1425 cm−1 is connected with the intermolecular hydrogen generated by -CH2- groups in non-crosslinked structure (Hamedi et al. Citation2013). The weak absorption strength of this peak for compound specimens might be due to the increased crosslinking density. The strong absorption peak near 1031 cm−1 related to the stretching vibration of C-O bond (Ciolacu, Ciolacu, and Popa Citation2011; Kondo and Sawatari Citation1996; Mohamad Haafiz et al. Citation2013). The increased absorption strength of this peak for Si-CNF/MF indicated the enhanced capacity to retain element C after double cross-linking. Besides, a new absorption peak at 774 cm−1 which due to the stretching vibration of Si-C can be observed in the spectra of Si-CNF and Si-CNF/MF (Tsiouris, Wheeler, and Lester Citation2001). Moreover, the absorption peaks around 1100 cm−1 and 462 cm−1 are caused by the asymmetric vibration of Si-O-Si bonds (Shao et al. Citation2012). The significantly increased absorption strength of these peaks for Si-CNF/MF demonstrated the formation of compact carbon layer after combustion, as well as the synergistic effect between MF and MTMS, which is also consistent with the results of TGA analysis.
Thermal conductivity
The thermal insulation performance of aerogel is another important parameter for its practical application. Therefore, the apparent density and thermal conductivity of pure and compound CNF aerogels were measured, and the results are listed in . As shown in , pure CNF aerogels exhibited ultra-low density (0.0061 g/cm3). After cross-linked by MF and/or MTMS, various degrees of increase in apparent density for compound CNF aerogels could be observed, i.e., 0.0089–0.01 g/cm3, which were attributed to the increase of crosslinking density and the decrease of pore size. Although the apparent density increased in a certain degree, the thermal conductivities of compound CNF aerogels always maintained in a relative low level. It is worth mentioning that even the double cross-linked specimen (Si-CNF/MF) exhibited much lower thermal conductivity (0.0369 W/mˑK) than previous reported value of flame-retarded cellulose aerogel (0.073 W/mˑK) (Han et al. Citation2015). This indicated that the addition of MF and/or MTMS exhibited no significant influence on the thermal insulation performance of compound CNF aerogels, and promising the application of flame retarded compound CNF aerogels as thermal insulation materials.
Micro calorimeter analysis
In order to further evaluate the flame retardancy of compound CNF aerogels, the combustion parameters, including heat release rate (HRR), total heat release (THR) et al, were measured by MCC. The HRR results are shown in . The THR test results show that pure CNF, Si-CNF, CNF/MF and Si-CNF/MF are 9.8, 4.3, 5.7 and 3.5, respectively. Compared with pure CNF aerogel, both pHRR and THR of compound CNF aerogels decreased significantly, which was caused by the crosslinking and/or decoration. Typically, the pHRR and THR of Si-CNF/MF decreased by 50.6% and 64.3%, respectively, which were mainly attributed to the condensed phase flame retardant effect of MTMS and gas phase flame retardant effect of MF. Moreover, it is known by the data of pHRR and THR for compound aerogels, the flame retardant efficiency of MTMS and MF were strengthened by the combined utilization, indicating that a synergistic effect existed between these two flame retardants. Furthermore, the temperature at pHRR (TpHRR) of all the compound CNF aerogels increased in comparison with pure CNF aerogel, which is consistent with the TGA results above. This demonstrated that the combustion temperature of compound CNF aerogels was efficient delayed by the addition of MF and/or MTMS. This favors the practical utilization of compound CNF aerogels as a flame retardant material.
Discussion in flame retardant mechanism
Relatively high loading of flame retardants was needed in order to achieve UL-94 V0 grade for compound aerogels. This was mainly attributed to the huge specific surface area of aerogel and the highly flammable characteristic of cellulose. Based on the results of flame retardant tests and carbon residue analysis, relevant flame retardant mechanism can be proposed and discussed, and shown in .
MF was composed by triazine rings with high crosslinking degree. Further condensation and/or crosslinking occurred, and to form a carbon layer which could act as a barrier between cellulose skeleton and flame during combustion. Simultaneously, a large number of nonflammable gas ammonia was produced by the decomposing of MF, which could dilute the concentration of oxygen and flammable gas produced by cellulose pyrolyzation. Whereas, the individual addition of MF was insufficient to form a compact carbon layer to retain the nonflammable gas on the surface of cellulose during combustion. When MTMS was incorporated, a strengthened carbon layer could be formed due to the generation of incombustible products such as SiC and SiO2, thereby synchronous improving the flame retardant efficiency of condensed and gas phases. Therefore, a synergistic effect between MF and MTMS existed during combustion, and the enhanced flame retardant properties of compound aerogels were mainly due to the strengthened carbon layer structure in condensed phase mechanism.
Conclusions
In this paper, CNF was successive double cross-linked and surface decorated by MF and MTMS to obtain flame retarded compound aerogels. Cross-linking reaction occurred between MF/MTMS and CNF matrix. The pore size of compound aerogels shrinked, whereas the pore amount increased after cross-linking, thereby resulting in the improved mechanical performance. Typically, the compression modulus and specific modulus of Si-CNF/MF increased to 22.91 KPa and 2268.32 KPa/g cm−3, equivalenting 3.0 and 1.8 times as those of pure CNF aerogel, respectively.
Moreover, the compound aerogels exhibited excellent flame retarded properties and hydrophobic property (contact angle up to 132.3°). The LOI value of Si-CNF/MF increased from 19.5% to 37.1%, and the UL-94 grade reached V-0 level. Significant decrease in pHRR and THR for Si-CNF/MF (50.6% and 64.3%, respectively) also demonstrated the excellent flame retardant properties of double cross-linked compound aerogel. Both condensed phase and gas phase flame retardant mechanisms occurred during combustion, and synergistic effect might be existed between MF and MTMS. The excellent flame retardancy, hydrophobicity and thermal insulation properties of the aerogel provide a new idea for the preparation of water-oil separation materials or thermal insulation materials.
Highlights
An interpenetrating network structure could be formed via double cross-linking, which was beneficial to the improvement of mechanical performance of cellulose aerogel.
A hydrophobic surface could be formed by the introduction of MTMS, which broaden the application of cellulose aerogels.
N/Si synergistic effect could be obtained via the incorporation of MF and MTMS, and resulting in the improved flame retardancy of cellulose aerogel (UL-94 V0 rate).
Author contributions
All authors contributed to the study conception and design. Material preparation, data collection and analysis were performed by Siqian Zhang and Jiachen He. The first draft of the manuscript was written by Siqian Zhang and Xiaopeng Yue. All authors commented on previous versions of the manuscript. All authors read and approved the final manuscript.
Disclosure statement
The authors declare that they have no conflict of interest. This article does not contain any studies with human participants or animals performed by any of the authors. Informed consent was obtained from all individual participants included in the study.
Additional information
Funding
References
- Ciolacu, D., F. Ciolacu, and V. I. Popa. 2011. Amorphous cellulose—structure and characterization. Cellulose Chemistry and Technology 45:13–15.
- Elanthikkal, S., U. Gopalakrishnapanicker, S. Varghese, and J. T. Guthrie. 2010. Cellulose microfibres produced from banana plant wastes: Isolation and characterization. Carbohydrate Polymers 80 (3):852–59. doi:10.1016/j.carbpol.2009.12.043.
- Fan, B., S. Chen, Q. Yao, Q. Sun, and C. Jin. 2017. Fabrication of cellulose nanofiber/AlOOH aerogel for flame retardant and thermal insulation. Materials 10 (3):311. doi:10.3390/ma10030311.
- Fischer, F., A. Rigacci, R. Pirard, S. Berthon-Fabry, and P. Achard. 2006. Cellulose-based aerogels. Polymer 47:7636–45. doi:10.1016/j.polymer.2006.09.004.
- Ghanadpour, M., B. Wicklein, F. Carosio, and L. Wagberg. 2018. All-natural and highly flame-resistant freeze-cast foams based on phosphorylated cellulose nanofibrils. Nanoscale 10:4085–95. doi:10.1039/c7nr09243a.
- Guo, L., Z. Chen, S. Lyu, F. Fu, and S. Wang. 2018. Highly flexible cross-linked cellulose nanofibril sponge-like aerogels with improved mechanical property and enhanced flame retardancy. Carbohydrate Polymers 179:333–40. doi:10.1016/j.carbpol.2017.09.084.
- Hamedi, M., E. Karabulut, A. Marais, A. Herland, G. Nyström, and L. Wågberg. 2013. Nanocellulose aerogels functionalized by rapid layer-by-layer assembly for high charge storage and beyond. Angewandte Chemie International Edition 52 (46):12038–42. doi:10.1002/anie.201305137.
- Han, Y., X. Zhang, X. Wu, and C. Lu. 2015. Flame retardant, heat insulating cellulose aerogels from waste cotton fabrics by in situ formation of magnesium hydroxide nanoparticles in cellulose gel nanostructures. ACS Sustainable Chemistry & Engineering 3 (8):1853–59. doi:10.1021/acssuschemeng.5b00438.
- Jiang, Y., W. Zhou, M. Jiang, P. Liu, and J. Xu. 2017. Flame retardant study of formalized polyvinyl alcohol fiber coated with melamine formaldehyde resins and the synergistic effect of copper ions. Polymer Degradation and Stability 144:331–43. doi:10.1016/j.polymdegradstab.2017.08.014.
- Joly, C., R. Gauthier, and M. Escoubes. 1996. Partial masking of cellulosic fiber hydrophilicity for composite applications. Water sorption by chemically modified fibers. Journal of Applied Polymer Science 61 (1):57–69. doi:10.1002/(SICI)1097-4628(19960705)61:1<57:AID-APP7>3.0.CO;2-T.
- Kim, H., J. R. Youn, and Y. S. Song. 2018. Eco-friendly flame retardant nanocrystalline cellulose prepared via silylation. Nanotechnology 29:455702. doi:10.1088/1361-6528/aadc87.
- Kondo, T., and C. Sawatari. 1996. A Fourier transform infra-red spectroscopic analysis of the character of hydrogen bonds in amorphous cellulose. Polymer 37:393–99. doi:10.1016/0032-3861(96)82908-9.
- Mohamad Haafiz, M. K., S. J. Eichhorn, A. Hassan, and M. Jawaid. 2013. Isolation and characterization of microcrystalline cellulose from oil palm biomass residue. Carbohydrate Polymers 93 (2):628–34. doi:10.1016/j.carbpol.2013.01.035.
- Mokhena, T. C., and M. J. John. 2020. Cellulose nanomaterials: New generation materials for solving global issues. Cellulose 27 (3):1149–94. doi:10.1007/s10570-019-02889-w.
- Nabipour, H., S. Nie, X. Wang, L. Song, and Y. Hu. 2020. Highly flame retardant zeolitic imidazole framework-8@cellulose composite aerogels as absorption materials for organic pollutants. Cellulose 27 (4):2237–51. doi:10.1007/s10570-019-02860-9.
- Nelson, M. L., and R. T. O’Connor. 1964. Relation of certain infrared bands to cellulose crystallinity and crystal lattice type. Part II. A new infrared ratio for estimation of crystallinity in celluloses I and II. Journal of Applied Polymer Science 8 (3):1325–41. doi:10.1002/app.1964.070080323.
- Nguyen, S. T., J. Feng, S. K. Ng, J. P. W. Wong, V. B. C. Tan, and H. M. Duong. 2014. Advanced thermal insulation and absorption properties of recycled cellulose aerogels. Colloids and Surfaces. A, Physicochemical and Engineering Aspects 445:128–34. doi:10.1016/j.colsurfa.2014.01.015.
- Nishida, M., T. Tanaka, T. Miki, Y. Hayakawa, and K. Kanayama. 2019. Integrated analysis of modified Japanese cypress using solid-state NMR spectra and nuclear magnetic relaxation times. Cellulose 26:3625–42. doi:10.1007/s10570-019-02330-2.
- Rahimi, S. K., R. Aeinehvand, K. Kim, and J. U. Otaigbe. 2017. Structure and biocompatibility of bioabsorbable nanocomposites of aliphatic-Aromatic copolyester and cellulose nanocrystals. Biomacromolecules 18:2179–94. doi:10.1021/acs.biomac.7b00578.
- Saake, B., C. Altaner, S.-J. Lee, and J. Puls. 2005. Endoglucanase degradation and enzyme-aided characterization of cellulose acetates. Macromolecular Symposia 223 (1):137–50. doi:10.1002/masy.200550510.
- Sai, H., R. Fu, L. Xing, J. Xiang, Z. Li, F. Li, and T. Zhang. 2015. Surface modification of bacterial cellulose aerogels’ web-like skeleton for oil/water separation. ACS Applied Materials & Interfaces 7 (13):7373–81. doi:10.1021/acsami.5b00846.
- Seddiqi, H., E. Oliae, H. Honarka, J. Jin, L. C. Geonzon, R. G. Bacabac, and J. Klein-Nulend. 2021. Cellulose and its derivatives: Towards biomedical applications. Cellulose 28:1893–931. doi:10.1007/s10570-020-03674-w.
- Shao, Z., G. Wu, X. Cheng, and Y. Zhang. 2012. Rapid synthesis of amine cross-linked epoxy and methyl co-modified silica aerogels by ambient pressure drying. Journal of Non-Crystalline Solids 358 (18–19):2612–15. doi:10.1016/j.jnoncrysol.2012.06.013.
- Siqueira, G., J. Bras, and A. Dufresne. 2010. Cellulosic bionanocomposites: A review of preparation, properties and applications. Polymers-Basel 2:728–65. doi:10.3390/polym2040728.
- Tsiouris, M., M. D. Wheeler, and M. I. Lester. 2001. Activation of the CH stretching vibrations in CH[sub 4]–OH entrance channel complexes: Spectroscopy and dynamics. The Journal of Chemical Physics 114 (1):187–97. doi:10.1063/1.1328747.
- Valo, H., S. Arola, P. Laaksonen, M. Torkkeli, L. Peltonen, M. B. Linder, R. Serimaa, S. Kuga, J. Hirvonen, and T. Laaksonen. 2013. Drug release from nanoparticles embedded in four different nanofibrillar cellulose aerogels. European Journal of Pharmaceutical Sciences 50 (1):69–77. doi:10.1016/j.ejps.2013.02.023.
- Wicklein, B., A. Kocjan, G. Salazar-Alvarez, F. Carosio, G. Camino, M. Antonietti, and L. Bergstrom. 2015. Thermally insulating and fire-retardant lightweight anisotropic foams based on nanocellulose and graphene oxide. Nature Nanotechnology 10 (3):277–83. doi:10.1038/nnano.2014.248.
- Wu, Y., Y. Li, L. Qin, F. Yang, and D. Wu. 2013. Monodispersed or narrow-dispersed melamine–formaldehyde resin polymer colloidal spheres: Preparation, size-control, modification, bioconjugation and particle formation mechanism. Journal of Materials Chemistry B 1 (2):204–12. doi:10.1039/c2tb00043a.
- Yang, L., A. Mukhopadhyay, Y. Jiao, Q. Yong, L. Chen, Y. Xing, J. Hamel, and H. Zhu. 2017. Ultralight, highly thermally insulating and fire resistant aerogel by encapsulating cellulose nanofibers with two-dimensional MoS2. Nanoscale 9:11452–62. doi:10.1039/c7nr02243c.
- Yang, W., C. Wei, A. Yuen, B. Lin, G. Yeoh, H. Lu, and W. Yang. 2022. Fire-retarded nanocomposite aerogels for multifunctional applications: A review. Composites Part B: Engineering 237:109866. doi:10.1016/j.compositesb.2022.109866.
- Zhong, C., C. Wang, F. Wang, H. Jia, P. Wei, and Y. Zhao. 2016. Application of tetra-n-methylammonium hydroxide on cellulose dissolution and isolation from sugarcane bagasse. Carbohydrate Polymers 136:979–87. doi:10.1016/j.carbpol.2015.10.001.