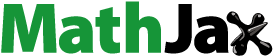
ABSTRACT
In this study, waste flax fibers with different levels of cellulosic and noncellulosic components were obtained by oxidative and alkali treatments and used to assess the influence of the fiber’s structural and chemical properties on the biosorption of lead ions. Scanning electron microscopy was used for the characterization of the flax fibers’ surface morphology, while the physicochemical properties of the fibers’ surface were determined by the streaming potential method, and Fourier transform infrared spectroscopy. Alkaline treatment decreased, while oxidative increased the crystallinity index of flax fibers by approximately 10%, due to the changes in the content of cellulosic components. Adsorption of lead ions was studied in detail, including kinetics, isotherms, and thermodynamic parameters analysis. It was shown that the amount and distribution of oxygen-containing groups, originating from cellulosic components, have the highest influence on the adsorption capacity of flax fibers, increasing Langmuir adsorption capacity from 12.76 mg g−1 for untreated to 21.9 and 79.9 mg g−1 for alkali-treated and oxidized fibers, respectively. Obtained negative values of ∆GӨ (−4.874 to −0.807 kJ mol−1) and ∆HӨ (−42.81 to −13.65 kJ mol−1) indicated that the adsorption of lead ions onto flax fibers is a spontaneous and endothermic process, which occurs through electrostatic attraction and ion-exchange. The results presented in this work demonstrate that the waste flax fibers of suitable chemical composition may be used as sustainable and renewable low-cost biosorbents.
摘要
在本研究中,通过氧化和碱处理获得了含有不同水平纤维素和非纤维素成分的废亚麻纤维,并用于评估纤维的结构和化学性质对铅离子生物吸附的影响. 采用扫描电子显微镜对亚麻纤维的表面形貌进行表征,同时采用流动电位法和傅里叶变换红外光谱法测定纤维表面的物理化学性质. 碱处理降低了亚麻纤维的结晶度指数,而氧化处理提高了约10%,这是由于纤维素组分含量的变化. 对铅离子的吸附进行了详细研究,包括动力学、等温线和热力学参数分析. 结果表明,来源于纤维素组分的含氧基团的数量和分布对亚麻纤维的吸附能力影响最大,将Langmuir吸附能力从未处理纤维的12.76 mg g-1提高到碱处理纤维和氧化纤维的21.9 mg g-1和79.9 mg g-1. 获得的负值∆G∞(-4.874至-0.807 kJ mol-1)和∆H∞(-42.81至-13.65 kJ mol-1)表明,铅离子在亚麻纤维上的吸附是一个自发的吸热过程,通过静电吸引和离子交换发生. 这项工作的结果表明,具有适当化学成分的废亚麻纤维可以用作可持续和可再生的低成本生物吸附剂.
Introduction
In recent years, textile products based on flax fibers, along with other natural cellulosic materials, have overturned the predominance of synthetic fibers. Owing to their good sorption and mechanical properties, as well as biocompatibility and biodegradability, flax fibers are widely used for the production of comfortable and anti-allergenic clothes and linen (Dalbaşı and Özçelik Kayseri Citation2019). These advantageous properties of flax fibers are derived from the specific cell wall structure, chemical composition, and position of the components within the fiber structure. Although several components may be included in the heterogeneous structure of the flax fiber, cellulose, hemicelluloses, and lignin are marked as the most important (Goudenhooft, Bourmaud, and Baley Citation2017). As the main constituent of the flax fiber structure, cellulose builds the microfibrils in the cell wall structure, while hydroxyl groups on the C2, C3, and C6 positions in cellulose monomer units are responsible for the formation of a supermolecular structure with amorphous and crystalline regions by creating intramolecular and intermolecular hydrogen bonds between the cellulose chains (Kostic et al. Citation2014). Hemicelluloses, which contain hydrophilic hydroxyl groups, are connected to the cellulose by hydrogen bonds and are deposited in the interfibrillar spaces of the primary and secondary walls. Lignin is a complex three-dimensional polyphenolic polymer with a high molecular weight and low reactivity, deposited in the middle lamellae, and the secondary wall (Pejić, Vukčević, and Kostić Citation2020). As mentioned previously, these main structural components contain specific functional groups (hydroxyl, carbonyl, and methoxyl) (Abutaleb et al. Citation2020), which, combined with the specific structure of flax fibers, promote their characteristics, especially their sorption properties. Therefore, this paper aims to correlate the fiber’s physicochemical characteristics with the process parameters obtained during the adsorption of lead ions on flax fibers, providing insight into the effect that the distribution and amount of cellulosic functional groups have on adsorption parameters. The presence of lead ions in the environment, especially in water, can originate from several natural (mineral dissolution, volcanic eruptions, forest fires, etc.) or anthropogenic (industrial sites, municipal waste, organic and mineral fertilizers, etc.) sources (Shirani et al. Citation2018). Lead ions represent permanent pollutants because they cannot be subjected to any degradation process, and their toxic effect on the environment is multiple due to bioaccumulative tendency and persistence. As a result of this, the concentration of lead ions in the soil, sediments, and waterways often exceeds the permissible levels. In that way, lead ions accumulate in the environment and in food chains, where they profoundly disrupt biological processes and even influence human health, which is reflected through the severe damage to the kidney, nervous system, reproductive system, liver, and brain. Therefore, it is essential to find the optimal method for removing lead ions from the environment, especially from water, since World Health Organization (WHO Citation2018) recommended the permissible level of lead ions in drinking water to be 0.01 mg L−1. Flax fiber samples of different chemical compositions, amounts, and availability of oxygen-containing groups were obtained by cheap and simple oxidative and alkali treatments (Lazić et al. Citation2018), and utilized as sustainable, economically, and environmentally friendly biosorbents for lead ion removal. At the same time, satisfying the need for a cleaner environment and circular economy, the reuse of waste flax fibers as adsorbents represents a special way of recycling, simultaneously decreasing the costs of waste disposal, and resolving environmental problems concerning water polluted with lead ions.
Materials and methods
Material preparation and characterization
The starting material in this investigation was short flax fibers (F) obtained from Banja Luka, the Republic of Srpska, Bosnia and Herzegovina. The chemical composition of raw flax fibers was as follows: α-cellulose, 75.81%; lignin, 4.03%; hemicelluloses, 7.84%. To obtain flax fiber samples with different contents of lignin and hemicelluloses, raw fibers were treated with 2% sodium chlorite and 18% sodium hydroxide. Oxidation and alkaline treatments were carried out for 30 min at room temperature and a 1:50 liquor ratio, to partially remove lignin and hemicelluloses, respectively. After the alkaline treatment, residual sodium hydroxide was neutralized with 1% acetic acid. Finally, oxidized (Fox) and alkali-treated (Fal) samples were washed and dried at 40°C overnight. The surface structure and morphology of raw and chemically treated flax fibers were examined by field emission scanning electron microscopy (FESEM, Mira3 Tescan), after sputtering with gold. The nature and type of functional groups present on the surface of the tested flax fibers were analyzed by attenuated total reflectance Fourier transform infrared spectroscopy (ATR-FTIR). The FTIR analysis was performed within a wavenumber range of 400–4000 cm−1 using the NicoletTM iSTM 10 FT-IR Spectrometer. Also, results obtained by the FTIR analysis were used to estimate the content of cellulose, hemicelluloses, and lignin on the flax fibers’ surface, through the ratios of hemicelluloses/cellulose and lignin/cellulose, as well as the surface total crystallinity index (TCI), i.e., the ratio between the crystalline and amorphous phase of cellulose present on the surface of the fibers. These estimations were made based on the ratio between the intensity of characteristic FTIR spectra bands (de Farias et al. Citation2017). Additionally, the content of the crystalline phase in the fiber structure was determined through iodine sorption. Iodine is absorbed in the amorphous phase of fiber, and iodine sorption values (ISV) (Vukcevic et al. Citation2014) represent the accessibility of cellulose to aqueous solutions. ISV is inversely proportional to the content of the fiber crystalline phase (Fakin et al. Citation2006), which can be given by the crystallinity index equation (XK, EquationEquation 1(1)
(1) )
where the ratio of ISV (in mg of iodine absorbed per 1 g of fibers) and 412 (in mg of iodine absorbed per 1 g of methylcellulose) determines the amorphous fraction. The quantitative analysis of functional groups was performed by the determination of carbonyl groups, with the aldehyde content in water-insoluble fractions measured according to literature (Röhrling et al. Citation2002), and the amount of carboxyl groups determined by the calcium-acetate method (Praskalo et al. Citation2009). The nature and amount of functional groups present on the surface of flax fibers affect their surface charge. Therefore, the SurPASS electrokinetic analyzer and the streaming potential method were used to determine the flax fibers’ zeta potential (ζ) and the isoelectric point (IEP), as described in the relevant literature (Kramar et al. Citation2018).
Adsorption experiments
The adsorption efficiency of tested flax fiber samples in removing lead ions was tested using aqueous solutions of Pb(NO)2. The adsorption was conducted in a batch system under constant shaking. Flax fibers (0.125 g) were soaked in 50 mL of a metal ion solution with different initial concentrations (5, 10, 15, and 25 mg L−1). The concentration of lead ions was determined with the atomic absorption spectrometer Pye Unicam SP9. The metal ion uptake (qe, mg g−1) was determined according to the equation (EquationEquation 2(2)
(2) ):
where Co and Ct (mg L−1) are the initial concentration, and the concentration of metal ions in the solution after a defined time, respectively; V (L) is the solution volume and m (g) is the weight of adsorbents (F, Fox, and Fal).
The influence of the solution’s pH on the adsorption capacities of flax fibers was examined through the adsorption of Pb2+ from an aqueous solution of different pH (3 to 7 by step of 1). The obtained results were compared with the activities (a) and speciation of lead ions present in the solution (obtained with the Visual Minteq 3.1 software), as well as the adsorbent’s pHIEP to determine the optimal pH value for adsorption experiments.
Pseudo-first order (Lagergren Citation1898) and pseudo-second order (Ho and Mckay Citation1999) kinetic models were applied to adsorption data to reveal the mechanism and the rate-controlling step of the lead ions’ adsorption onto the tested flax fiber samples. Pseudo-first-order and pseudo-second-order kinetics are given by EquationEquation 3(3)
(3) and Equation4
(4)
(4) , respectively:
where qe and qt are the amounts of lead ions adsorbed at equilibrium in mg g−1, and at time t in min, respectively, k1 is the pseudo-first-order rate constant (min−1) and k2 (g mg−1 min−1) is the pseudo-second-order rate constant.
Langmuir (Langmuir Citation1918) and Freundlich (Freundlich Citation1906) isotherm equations were applied for modeling the equilibrium adsorption data, and to assess the maximum adsorption capacities on untreated and chemically treated flax fibers. The Langmuir and Freundlich isotherms are given by EquationEquations (5)(5)
(5) and (Equation6
(6)
(6) ), respectively:
where qe is the amount of adsorbed metal ions (mg g−1), Ce is the metal ions equilibrium concentration (mg L−1), Qo is the amount of solute adsorbed per unit mass of adsorbent required for monolayer coverage of the surface (mg g−1), and b is a constant related to the heat of adsorption (L mg−1). The Freundlich constant, KF, indicates the adsorption capacity, while parameter nF indicates the heterogeneity of the adsorbent surface.
The dependence of flax fibers’ adsorption capacity on temperature was evaluated by conducting the adsorption experiments in a temperature-controlled water bath, at 25°C, 35°C, and 45°C. The thermodynamic parameters of the adsorption process were calculated by taking into account the activity of the positively charged Pb2+ species (Liu Citation2009) and using the following equations:
where ;
and
The values of ∆HӨ and ∆SӨ were estimated from the slopes and intercepts of lnKa vs. the 1/T plot, and the values of ∆GӨ were calculated from the corresponding values of ∆HӨ and ∆SӨ following Eq. 8.
Results and discussion
Characterization of flax fiber samples
Oxidative and alkaline treatments were applied to obtain flax fiber samples of different chemical compositions. Differences in α-cellulose, hemicelluloses, and lignin content, induced by the applied treatments are shown in .
As a consequence of oxidation, the lignin content of flax fibers decreases from 4.03% (F) to 0.29% (Fox), due to a considerable removal of lignin from the secondary wall and the middle lamellae. At the same time, this treatment slightly increases the content of α-cellulose and hemicelluloses. On the other hand, the applied alkaline treatment is responsible for a decrease in the content of hemicelluloses by about 63%, due to its removal from the interfibrillar and amorphous region of the flax fibers’ structure. This treatment also slightly decreases the lignin content.
The differences in lignin and hemicelluloses content, along with their location in the structure of the fiber, may affect the morphological and adsorption characteristics of samples F, Fox, and Fal. Changes in fibers’ structure induced by chemical treatments are shown in .
Removal of hemicelluloses caused relative peeling of the fibers’ surface, along with the formation of new open spaces between distinctly liberated elementary fibers in the structure of Fal (). The structure of the sample Fox () is characterized by partially separated elementary fibers, due to lignin removal. The observed smooth surface of the Fox sample is a consequence of intensive surface peeling and elimination of micro-pores, as well as the increased flexibility of the cell wall (Kostic et al. Citation2014).
The changes in flax fibers’ surface chemistry, which are the result of partial elimination of lignin and hemicelluloses, were analyzed by FTIR spectroscopy. The FTIR spectra of the row sample (F) and samples with a lower content of hemicelluloses (Fal) and lignin (Fox) are given in .
Figure 3. FTIR spectra of the row sample (F), and samples with a lower content of hemicelluloses (Fal) and lignin (Fox).
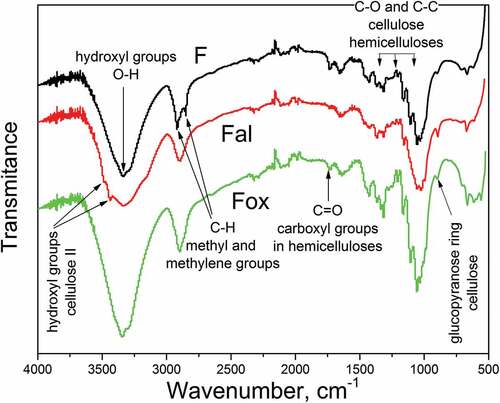
All analyzed samples exhibited a broad band around 3340 cm−1 which is attributed to the stretching of O-H bond in hydroxyl groups. Two shoulders were observed for sample Fal: the shoulder at 3478 cm−1 was assigned to hydroxyl groups in cellulose II, while the shoulder at 3436 cm−1 was attributed to the specific hydrogen bonds between O(3)H and O(5). These changes in the FTIR spectra of Fal were caused by the polymorphic transformation of cellulose I to the more reactive cellulose II, which occurred during alkali treatment (Oh et al. Citation2005). Two peaks, with maxima at 2850 cm−1 and 2920 cm−1, were attributed to the symmetrical and asymmetrical vibrations of the C-H bond in methyl and methylene groups (Zhang et al. Citation2015) of cellulose, hemicelluloses (Zhang et al. Citation2015), and lignin (Mohapatra and Malik Citation2015) in the structure of sample F. The differences in the FTIR spectra of the examined samples, observed in this wavenumber region, originate from the removal of hemicelluloses and lignin through applied chemical treatments. The peak near 1730 cm−1 was attributed to the C=O stretching of carboxyl or ester groups of hemicelluloses (Sawpan, Pickering, and Fernyhough Citation2011; Zhang et al. Citation2015), and its intensity decreases with the decrease in the content of hemicelluloses. The bands observed between 1365 and 1000 cm−1 are mainly assigned to C-O and C-C stretching in polysaccharides, cellulose, and hemicelluloses. A decrease in intensity, and disappearance of peaks in this wavenumber region, observed for sample Fal, appears to be the consequence of hemicelluloses removal. For all tested samples, a peak at 898 cm−1 was assigned to stretching in the glucopyranose ring, related to both amorphous and crystalline cellulose.
Results of the FTIR analysis confirmed that the changes in lignin and hemicelluloses content, along with the changes in the structure of flax fibers induced by oxidation and alkali treatment, lead to a different distribution of these constituents on the fiber surface. Based on these results, the ratios between lignin and cellulose, as well as the hemicelluloses and celluloses on the fiber surface, can be estimated. The peak at 1508 cm−1, which is associated with aromatic skeletal vibrations in lignin, was used for the quantification of lignin content. Cellulose content was quantified based on the intensity of the peak at 1317 cm−1, which is related to the CH2 in plane-angular vibration in crystalline cellulose, while the peak at 1730 cm−1 (attributed to the C=O stretching of carboxyl or ester groups of hemicelluloses) was used for quantification of hemicelluloses (de Farias et al. Citation2017). Also, the total crystallinity index (TCI) of flax fibers was determined by FTIR spectroscopy as the ratio of absorbance at 1368 cm−1 and 2885 cm−1, which were assigned to the in-the-plane CH bending and C-H symmetrical stretching in cellulose and hemicelluloses, respectively (Dai and Fan Citation2011). The obtained results are given in .
Table 1. Crystallinity index (XK) and total crystallinity index (TCI) of untreated, alkali, and oxidative treated flax fibers, and the ratio of different FTIR peaks for hemicelluloses and lignin content estimation.
The observed decreases in both Lignin/Cellulose and Hemicelluloses/Cellulose Ratios for Fal and Fox samples were the consequence of the lignin and hemicelluloses removal by applied treatments. These results indicate that more cellulose can be detected on the surface of the treated samples, although lignin and hemicelluloses are still present. Values of TCI indicate that the alkali treatment leads to a higher content of amorphous regions in cellulose, while the oxidative treatment enriches the fiber surface with crystalline cellulose through the depletion of the amorphous cellulose phases. The values of the crystallinity index (XK) (), obtained as a result of iodine absorption by flax fiber samples, showed the same trend: the content of the crystalline phases in the fiber structure increases after the oxidation of flax fibers and decreases after the alkaline treatment.
Quantitative information on functional groups present on the surface of flax fibers is given in . The applied alkali treatment significantly decreased the amount of aldehyde groups, while the number of carboxyl groups present on the Fal surface was only slightly changed. Therefore, it can be assumed that hemicelluloses are most likely the source of removed aldehyde and carboxyl groups, which corresponds with the results of the FTIR analysis, i.e., the changes in the wavenumber region of 1365–1000 cm−1 obtained for sample Fal. On the other hand, the oxidative treatment leads to the almost unchanged content of aldehyde groups and the formation of new carboxyl groups on the surface of Fox. Removal of lignin by oxidative treatment leads to the cleaning and reduction in surface roughness (), making cellulose on the fiber surface more exposed. Thus, the cellulose becomes more susceptible and available for oxidation, which leads to an increased number of surface functional carboxyl groups for sample Fox (Tarbuk et al. Citation2020).
Figure 4. Surface chemistry of tested flax fiber samples: a) amount of carbonyl and carboxyl groups and b) zeta potential as a function of pH.
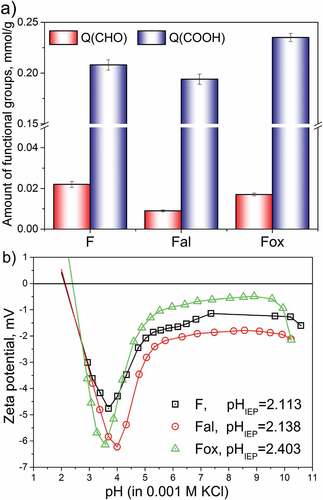
The presence and availability of cellulose, hemicelluloses, and lignin, accompanied by the presence of the characteristic surface group, lead to differences in the surface charge of flax fibers. Information about the surface charge of all tested samples was provided by zeta potential measurements. Depending on the pH value of the surrounding aqueous solution, the surface of flax fibers can be positively or negatively charged. At the pH values of an aqueous solution lower than the isoelectric point, the fiber surface is protonated, while in the solution with pH values higher than the isoelectric point, functional groups are deprotonated, and may attract and bind metal ions (Mihajlović et al. Citation2020; Vukcevic et al. Citation2014). The observed changes in structure and chemical composition of flax fiber samples, induced by applied treatments, lead to differences in the zeta potential value, especially in a basic range of pH (ζplateau) (). Compared to the untreated sample, F, the alkali-treated flax fibers showed a slightly more negative ζplateau value, which is probably a consequence of elementary fiber liberation and a more open structure of fibers (), since no new negatively charged groups have been incorporated. On the other hand, the slightly more positive ζplateau value obtained for the oxidized sample was most likely caused by partial liberation of elementary fibers and the highest degree of swelling (i.e., the highest ratio between the maximum negative zeta potential value at the acidic pH and the plateau value at the basic pH, ζmax/ζplateau), which was observed for sample Fox. The highest degree of swelling is most likely the consequence of lignin removal, which makes cellulose more exposed on the Fox surface. This increased presence of cellulose and hemicelluloses, along with the increased aldehyde and carboxyl group content (), resulted in the shifting of the isoelectric point of sample Fox toward a higher pH, compared to the IEP values of samples F and Fal. Based on the obtained IEP values, it is expected that in a solution with a pH higher than 2.5, the surfaces of all tested samples are negatively charged and ready for the adsorption of positive metal ions.
Adsorption of lead ions by flax fiber samples
The pH value of the aqueous solution affects the adsorption of lead ions by flax fibers through the ionization of surface oxygen-containing groups, along with the solubility and speciation of lead ions in the solution. illustrates the activity of different ion forms present in the lead ions aqueous solution, depending on the pH value of the solution. Below pH 5, hydronium ions showed the highest activity and were, therefore, in this pH region, more competitive than lead ions when it comes to adsorption onto flax fibers. In the solution with a pH higher than 8, lead ions precipitated as Pb(OH)2, while in the pH ranging from 5 to 7.5, Pb2+ ions showed the highest activity. Since the pHIEP values of flax fiber samples, ranging from 2.11 to 2.40, were lower than pH 5, the pH region (5–7.5) with the highest activity of Pb2+ was chosen as optimal for the adsorption onto flax fibers. In this pH region, hydronium ions show a low activity, lead ions are in a suitable form, and the surface functional groups are deprotonated and can attract and bind positive metal ions.
Figure 5. a) Speciation of lead ions in aqueous solution, and b) amount of lead ions adsorbed on the surface of flax fiber samples as a function of aqueous solution pH.
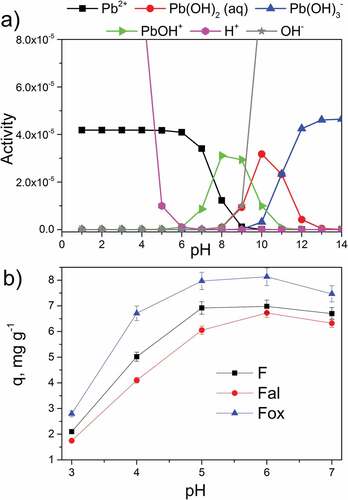
The effect of the solution’s pH on the adsorption of lead ions by flax fiber samples is illustrated in . For all tested pH values, the oxidized flax fibers (Fox) showed the highest adsorption efficiency, while the alkali treatment deteriorated adsorption efficiency compared to the untreated flax fibers. The obtained results confirm that for all tested samples, pH 6 is the most favorable for the adsorption of lead ions.
The effect of contact time, the concentration of lead ions in the solution, and solution temperature on the adsorption capacities of untreated, alkali, and oxidative treated flax are presented in . The increase in adsorption capacities with an increase in contact time, the concentration of lead ions and the temperature is noticeable for all tested samples. Sample Fox showed the highest adsorption capacity for lead ions, as well as the highest rate of adsorption for all tested temperatures (), most likely due to the increased amount of hemicelluloses () and the highest swelling of the fibers () induced by lignin removal.
Figure 6. Effect of contact time and temperature (a), and lead ions concentration (b) on adsorption capacities of flax fibers.
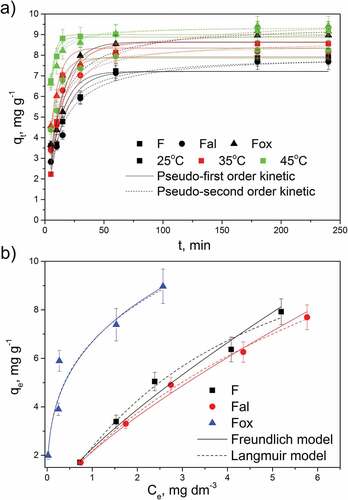
To obtain information about the adsorption rate, and the influence of the temperature increase on the adsorption rate, the experimental data were fitted with pseudo-first- and pseudo-second-order kinetic models, and the kinetic parameters are summarized in . The adsorption of lead ions onto flax fiber samples can be described equally well by both kinetic models. However, according to the coefficient of determination (R2), the adsorption data was slightly better fitted by the pseudo-second-order kinetic model. Lead ions adsorption was the fastest on sample Fox, increasing with the temperature for all samples, which was confirmed by the values of the rate constants (). For all tested temperatures, the most efficient adsorption, regarding rate and capacity, was observed for sample Fox, resulting from the partial liberation of elementary fibers, surface peeling, and increased accessibility of cellulose functional groups on the fiber surface. The observed increase in the adsorption capacities at higher temperatures () is the result of the increased diffusion, which facilitates the penetration of lead ions through the flax fibers’ structure. Nevertheless, the obtained results showed that alkaline-treated fibers (Fal), despite the complete liberation of elementary fibers, have the lowest adsorption capacity. This observation confirms that the availability and amount of oxygen-containing groups originating from cellulosic components (especially hemicelluloses) have a greater impact on the biosorption of lead ions than the fiber structure and morphology.
Table 2. Kinetic and thermodynamic parameters of lead ions adsorption on flax fiber samples on different temperatures (T, K).
The relation between the concentration of lead ions and its uptake degree by the solid phase was described by the Freundlich and Langmuir isotherm (). The parameters obtained by these isotherms can be used to clarify the distribution of lead ions between the liquid phase and the flax fibers’ surface. Ion distribution can be a consequence of several factors combined, such as the heterogeneity of the adsorbent surface, the type of surface coverage by ions, and the type of interaction between the adsorbent surface and lead ions. According to the Langmuir model, the monolayer adsorption of ions from the solution occurs on the surface of the adsorbent which is characterized by the homogeneous distribution of active sites with identical adsorption energy. On the other side, the Freundlich model provides information about the strength (parameter Kf) of the bond established between lead ions and the adsorbent surface and about the surface heterogeneity (nF). Model parameters for the system lead ions – tested flax fiber samples obtained through the nonlinear regression analysis of Freundlich and Langmuir isotherms, are given in .
Table 3. Model parameters for the system lead ions – tested flax fiber samples obtained through nonlinear regression analysis of applied isotherms.
Based on the coefficient of determination values, the adsorption of lead ions onto flax fiber samples at equilibrium shows good agreement with both isotherm models. Nevertheless, a slightly better agreement with the Langmuir isotherm model was noticed for samples F and Fal that, according to the nF value, have a more homogeneous surface than Fox. On the other hand, sample Fox showed a slightly better correlation with the Freundlich model and the nF value that indicates a heterogeneous surface. Values of parameter KF that indicates the capacity of the adsorbent showed that sample Fox has the highest adsorption capacity toward lead ions, which corresponds with maximum adsorption capacities obtained by the Langmuir isotherm. The better adsorption capacity of Fox with a more heterogeneous distribution of surface active sites is the consequence of the increased amount of surface oxygen-containing groups (), originating from the cellulose and hemicelluloses that become more available by lignin removal.
Adsorption experiments performed at different temperatures provided data for calculating the thermodynamic parameters (enthalpy ΔHӨ, entropy ΔSӨ, and Gibbs energy change ΔGӨ) of the adsorption of lead ions on untreated and chemically treated flax fibers (). The adsorption of lead ions onto the F, Fal, and Fox is an endothermic and spontaneous process because positive values were obtained for ΔHӨ and negative values were obtained for ΔGӨ. The positive values of ∆SӨ indicate that the disorder of the boundary surface between the solid and liquid phases, as well as the mobility of the ions in the solution and their affinity to the adsorbent surface, increases with the solution temperature. The values of enthalpy and Gibbs free energy can provide information about the physical or chemical nature of the adsorption process. The Gibbs energy change between −20 and 0 kJ mol−1 is characteristic for physical adsorption, while chemical adsorption is characterized by Gibbs energy change in the range from −400 to −80 kJ mol−1 (Vukčević et al. Citation2017). On the other hand, an enthalpy change between 2 and 21 kJ mol−1 points to physical adsorption, while enthalpy values from 80 to 200 kJ mol−1 indicate chemical adsorption. According to the values of the Gibbs energy change (), the adsorption of lead ions on flax fibers’ surface can be considered as physisorption. The values of enthalpy obtained for F and Fox favor neither physisorption nor chemisorption, while in the case of sample Fal, the enthalpy change suggested a mechanism of physisorption. It was shown that the amount and availability of surface functional groups affect the adsorption since sample Fox showed the highest adsorption capacity. It can be assumed that the adsorption of lead ions onto flax fibers may occur through an ion-exchange mechanism, which is confirmed by the positive values of ∆SӨ (). Additionally, having the pH adjusted to the optimal value of 6 in the adsorption solution, the surface of flax fiber samples is negatively charged and the adsorption of lead ions could occur due to the attractive electrostatic forces, favoring the ion-exchange mechanism. According to these results, the adsorption of Pb2+ ions onto flax fibers is a complex process, in which both physisorption and ion-exchange may participate. To assess whether the ion interaction between the lead ion and the surface oxygen-containing groups contributed to the adsorption mechanism, a FTIR analysis of flax fibers before and after adsorption was performed ().
Figure 7. ATR-FTIR spectra before and after lead ions adsorption onto a) F, b) Fal, and c) Fox sample.
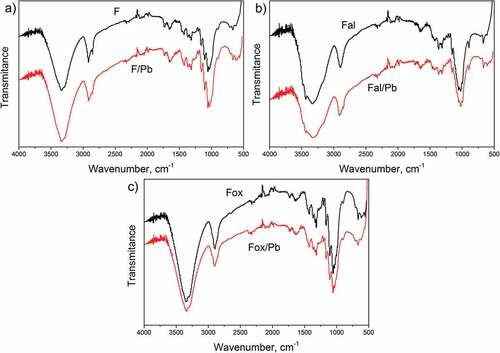
As can be seen from , lead ions adsorption generally does not influence the FTIR spectra of untreated, as well as chemically treated flax fibers, indicating the adsorption of lead ions does not change the chemical structure of flax fibers. However, slight changes in the intensities observed in the peaks around 3340 cm−1, 1730 cm−1, and in the region from 1365 to 1000 cm−1, suggest that surface hydroxyl and carboxyl groups participate in the binding of lead ions (Hokkanen, Bhatnagar, and Sillanpää Citation2016).
Reusability of flax fibers after adsorption
In order to satisfy the need for a cleaner environment and circular economy, it is necessary to find a way to reuse, or safely dispose of the waste flax fibers, separated after the adsorption of lead ions. Used flax fibers adsorbents can potentially be applied to improve soil quality through co-composting, due to the biodegradability of flax fibers. Taking into account the proposed concentration of lead in the agricultural soil (400 ppm) (EPA Citation2018), and the ion concentration in the used adsorbent (7.70–9.42 × 103 ppm), the compost with manure should be prepared in the ratio of 1:1000.
Conclusion
Removal of hemicelluloses and lignin by applied treatments induced the changes in a fiber structure by total or partial liberation of elementary fibers, as well as changes in the availability of components on the fiber surface. Despite the higher liberation of elementary fibers that enable the easier penetration and diffusion of metal ions through the fiber matrices, the alkali-treated sample showed lower adsorption capacity than the oxidized sample. Lignin removal increased the amount, and surface exposure of cellulose and hemicelluloses, making their surface functional groups more available for adsorption. It was shown that adsorption capacity was more dependent on the content of the cellulosic components, especially hemicelluloses, than the liberation of fiber structure. Adsorption and thermodynamic studies showed that lead ion adsorption onto flax fibers is a spontaneous and complex endothermic process that occurs through electrostatic attraction and ion-exchange. Results obtained in this work showed that waste flax fibers of appropriate chemical composition may be used as sustainable and renewable low-cost biosorbent.
Highlights
Waste flax fibers are used as a sustainable and renewable low-cost biosorbent
Flax oxidation leads to the formation of a structure that facilitates lead adsorption
Adsorption capacity depends on the distribution of cellulosic oxygen groups and content of hemicelluloses
The adsorption of lead onto flax fibers is a spontaneous and endothermic process
Flax fibers bind lead ions through the mechanism of physisorption and ion-exchange
Disclosure statement
No potential conflict of interest was reported by the author(s).
Additional information
Funding
References
- Abutaleb, A., A. Tayeb, M. Mahmoud, A. Daher, O. Desouky, O. Bakather, and R. Farouq. 2020. Removal and recovery of U(VI) from aqueous effluents by flax fiber: Adsorption, desorption and batch adsorber proposal. Journal of Advanced Research 22:153–14. doi:10.1016/j.jare.2019.10.011.
- Dai, D., and M. Fan. 2011. Investigation of the dislocation of natural fibres by Fourier-transform infrared spectroscopy. Vibrational Spectroscopy 55:300–06. doi:10.1016/j.vibspec.2010.12.009.
- Dalbaşı, E. S., and G. Özçelik Kayseri. 2019. A research on the comfort properties of linen fabrics subjected to various finishing treatments. Journal of Natural Fibers 18:909–22. doi:10.1080/15440478.2019.1675210.
- de Farias, J. G. G., R. C. Cavalcante, B. R. Canabarro, H. M. Viana, S. Scholz, and R. A. Simão. 2017. Surface lignin removal on coir fibers by plasma treatment for improved adhesion in thermoplastic starch composites. Carbohydrate Polymers 165:429–36. doi:10.1016/j.carbpol.2017.02.042.
- EPA. 2018. Lead in soil. U.S. Environmental Protection Agency, Region III. https://www.epa.gov/sites/default/files/2020-10/documents/lead-in-soil-aug2020.pdf
- Fakin, D., V. Golob, K. Stana Kleinschek, and A. Majcen Le Marechal. 2006. Sorption properties of flax fibers depending on pretreatment processes and their environmental impact. Textile Research Journal 76:448–54. doi:10.1177/0040517506062767.
- Freundlich, H. 1906. Adsorption in solutions. The Journal of Physical Chemistry 57:384–410.
- Goudenhooft, C., A. Bourmaud, and C. Baley. 2017. Varietal selection of flax over time: Evolution of plant architecture related to influence on the mechanical properties of fibers. Industrial Crops and Products 97:56–64. doi:10.1016/j.indcrop.2016.11.062.
- Hokkanen, S., A. Bhatnagar, and M. Sillanpää. 2016. A review on modification methods to cellulose-based adsorbents to improve adsorption capacity. Water Research 91:156–73. doi:10.1016/j.watres.2016.01.008.
- Ho, Y. S., and G. Mckay. 1999. Pseudo-second order model for sorption processes. Process Biochemistry 34 (5):451–65. doi:10.1016/S0032-9592(98)00112-5.
- Kostic, M., M. Vukcevic, B. Pejic, and A. Kalijadis. 2014. Hemp fibers: Old fibers - new applications. In Textiles: History, properties and performance and applications, ed. M. I. M. Mondal, 399–446. New York: Nova Science Publishers.
- Lagergren, S. 1898. Zur Theorieder sogennanten adsorption geloester stoffe, Kungliga Svenska Vetenskapsakademiens. Handlingar 24 (4):1–39.
- Langmuir, I. 1918. The adsorption of gases on plane surfaces of glass, mica and platinum. Journal of the American Chemical Society 40 (9):1361–403. doi:10.1021/ja02242a004.
- Lazić, B. D., B. M. Pejić, A. D. Kramar, M. M. Vukčević, K. Mihajlovski, J. D. Rusmirović, and M. M. Kostić. 2018. Influence of hemicelluloses and lignin content on structure and sorption properties of flax fibers (Linum usitatissimum L.). Cellulose 25 (1):697–709. doi:10.1007/s10570-017-1575-4.
- Liu, Y. 2009. Is the free energy change of adsorption correctly calculated? Journal of Chemical & Engineering Data 54:1981–85. doi:10.1021/je800661q.
- Mihajlović, S., M. Vukčević, B. Pejić, A. Perić Grujić, and M. Ristić. 2020. Application of waste cotton yarn as adsorbent of heavy metal ions from single and mixed solutions. Environmental Science and Pollution Research 27:35769–81. doi:10.1007/s11356-020-09811-z.
- Mohapatra, H. S., and R. K. Malik. 2015. Effect of microorganism on flax and linen. Journal of Textile Science and Engineering 6 (1):229. doi:10.4172/2165-8064.1000229.
- Oh, S. Y., D. I. Yoo, Y. Shin, and G. Seo. 2005. FTIR analysis of cellulose treated with sodium hydroxide and carbon dioxide. Carbohydrate Research 340:417–28. doi:10.1016/j.carres.2004.11.027.
- Pejić, B., M. Vukčević, and M. Kostić. 2020. Hemp fibers in Serbia: Cultivation, processing and applications. In Sustainable agriculture reviews 42, ed. G. Crini and E. Lichtfouse, 111–46. Switzerland: Springer Nature Switzerland AG.
- Praskalo, J., M. Kostic, A. Potthast, G. Popov, B. Pejic, and P. Skundric. 2009. Sorption properties of TEMPO-oxidized natural and man-made cellulose fibers. Carbohydrate Polymers 77:791–98. doi:10.1016/j.carbpol.2009.02.028.
- Röhrling, J., A. Potthast, T. Rosenau, T. Lange, A. Borgards, H. Sixta, and P. Kosma. 2002. A novel method for the determination of carbonyl groups in cellulosics by fluorescence labeling. 2. Validation and applications. Biomacromolecules 3:969–75. doi:10.1021/bm020030p.
- Sawpan, M. A., K. L. Pickering, and A. Fernyhough. 2011. Effect of various chemical treatments on the fibre structure and tensile properties of industrial hemp fibres. Composites, Part A 42:888–95. doi:10.1016/j.compositesa.2011.03.008.
- Shirani, Z., C. Santhosha, J. Iqbal, and A. Bhatnagar. 2018. Waste Moringa oleifera seed pods as green sorbent for efficient removal of toxic aquatic pollutants. Journal of Environmental Management 227:95–106. doi:10.1016/j.jenvman.2018.08.077.
- Tarbuk, A., K. Grgić, E. Toshikj, D. Domović, D. Dimitrovski, V. Dimova, and I. Jordanov. 2020. Monitoring of cellulose oxidation level by electrokinetic phenomena and numeric prediction model. Cellulose 27:3107–19. doi:10.1007/s10570-020-03028-6.
- Vukcevic, M., B. Pejic, M. Lausevic, I. Pajic-Lijakovic, and M. Kostic. 2014. Influence of chemically modified short hemp fiber structure on biosorption process of Zn2+ ions from waste water. Fibers and Polymers 15:687–97. doi:10.1007/s12221-014-0687-9.
- Vukčević, M., B. Pejić, I. Pajić-Lijaković, A. Kalijadis, M. Kostić, Z. Laušević, and M. Laušević. 2017. Influence of the precursor chemical composition on heavy metal adsorption properties of hemp (Cannabis Sativa) fibers based biocarbon. Journal of Serbian Chemical Society 82:1417–31. https://doi.org/10.2298/JSC170310080V
- WHO. 2018. A global overview of national regulations and standards for drinking-water quality. World Health Organization. https://apps.who.int/iris/rest/bitstreams/1135599/retrieve
- Zhang, H., R. Ming, G. Yang, Y. Li, Q. Li, and H. Shao. 2015. Influence of alkali treatment on flax fiber for use as reinforcements in polylactide stereocomplex composites. Polymer Engineering and Science 55:2553–58. doi:10.1002/pen.24147.