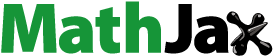
ABSTRACT
The interest in developing bio-based composite materials had grown up with the context of maintaining ecological integrity by introducing new eco-friendly materials. The present study investigated the suitability of a novel Furcraea selloa K. Koch leaf fiber (FSLF) for composite reinforcement instead of existing synthetic harmful fiber materials. The identification for exploration of novel natural fibers with significant properties is a great challenge for researchers, due to their accessibility in polymer composites eco-friendliness and sustainability. Indeed, physicochemical, thermal, mechanical, and morphological features were established through this study, low density (810 kg/m3), high crystallinity (56.7%), high thermal stability (350°C), good tensile strength (650 ± 23 MPa) were the notable features of FSLF that facilitate its further use for composite reinforcement. Moreover, good surface roughness with fewer impurities and low microfibril angle also prove its sustainability in composite reinforcement. This study suggests that the potential properties of FSLF would be a suitable alternative to commercially important synthetic fiber, in the highest seeking-fibers as reinforcement.
摘要
开发生物基复合材料的兴趣是在通过引入新的环保材料来保持生态完整性的背景下产生的. 本研究研究了一种新型 Furcraea selloa K.Koch叶纤维(FSLF)代替现有的合成有害纤维材料用于复合材料增强的适用性. 由于其在聚合物复合材料中的可及性、生态友好性和可持续性,对研究人员来说,识别和探索具有重要性能的新型天然纤维是一个巨大的挑战. 事实上,通过本研究确定了物理化学、热、机械和形态特征,低密度(810 kg/m3)、高结晶度(56.7%)、高热稳定性(350°C)和良好的拉伸强度(650 ± 23 MPa)是FSLF的显著特征,有助于其进一步用于复合材料增强. 此外,良好的表面粗糙度、较少的杂质和低的微纤维角也证明了其在复合材料增强中的可持续性. 这项研究表明,FSLF的潜在性能将是商业上重要的合成纤维的合适替代品,在作为增强材料的最高寻求纤维中.
Introduction
Natural fibers and their composites are getting more attention today due to increasing environmental concerns and stringent government policies. These products use renewable sources to replace petroleum-based components (Rajeshkumar et al. Citation2021). Moreover they are nontoxic, biodegradable, lightweight, lesser energy consumption during processing and machining (Gedik Citation2021). As a result, the growing demand for natural cellulosic fibers made many investigators to work on the same aspect to explore the novel fibre with desirable characteristic for composite reinforcing (Das and Chaudhary Citation2021; Pandit et al. Citation2021; Babu et al. Citation2022). The origin of fibres may vary in stalk, stem, fruit, bark, root, leaf, etc. according to the nature of the plant selected (Sundaram et al. Citation2021). Moreover, the availability of plant, length, and strength of the fibre are the other factors that affect its exploration at the commercial level (Saravana Kumaar et al. Citation2019). Natural sources are to be explored to introduce novel fibres with promising processing properties. The characteristics of the fibre and its binding properties determine the quality of a biocomposite (Dharmalingam et al. Citation2020). The various constituents of bio-fibre such as lignin, cellulose, wax, pectin, and hemicellulose give strength to the fibre. However easy availability, inexpensive nature, physicochemical and morphological features establishe preliminary identification of potential fibers (Amutha and Senthilkumar Citation2021; Raju et al. Citation2021). The composition of chemical components of the fibre is needed to be investigated to compare its properties with available ones. Other techniques such as FTIR, NMR spectroscopy, X-ray diffraction, TGA analysis are used to identify functional groups, crystalline structure, and thermal stability of the respective fibre (Prithiviraj and Muralikannan Citation2020; Raja et al. Citation2021a). The microscopic analytic methods such as SEM and AFM are used to examine the morphology of the fibre’s surface. Furthermore, the individual strength of the fibre are determined by a single fibre tensile test (Ramesh Babu and Rameshkannan Citation2021).
Furcraea selloa K.Koch (also known as wild sisal) is a monocarpic succulent species with a stem up to 1.5 m long endowed with a rosette having 30–50 leaves of about 2.5 meters of diameter (). The plant is harvested from the wild for the fiber obtained from its leaves for the production of cordages. The leaves are lanceolate and narrow at the base, rigid, fleshly, concave above and convex below. Margins have curved teeth or serrated edges that usually blooms starting from 8–10 years of age producing an erect panicle terminal inflorescences, flower (Divya et al. Citation2022). The tall flower spike bears many small flowers followed by bulbils which grow into new plants. Abundant availability in tropical regions, as well as the length of leaves are long and strong, it would be strengthening economics for farmers if the outlook is transformed in the perspective of worldwide preference for natural fibers in creating green composites. Considering these reasons, Furcraea selloa K.Koch leaf was chosen for the identification of fibre in this study.Fibres from FSLF were extracted and characterized with morphological, anatomical, crystalline, thermal and physicochemical properties to explore its novelty and adorability to utilize it as better reinforcement in bio-composites. On the basis of the characterization results, this unique FSLF fibre can be considered for use in the composites sector with other strong natural fibres like as jute, flax, hemp, and coir. It has the potential to replace the synthetic fibres that dominate the composites industry. Their biodegradability makes them more desirable in considering current environmental protection rules and in production of green composites for lightweight structural applications.
Materials and procedures
Fibre extraction
The FSLF was acquired from the village anjugramam, Kanyakumari district of Tamilnadu, India, and its leaf was separated and water sterilized to remove dust particles and other impurities. For the retting procedure, the collected leaf was soaked in freshwater for two weeks at room temperature to enhance microbial degradation (). After that fibers were separated from the leaf through combing and unwanted impurities were removed by washing many times with demineralized water (&d) (Soma Sundaram Pillai et al. Citation2021). The fibers were then dried in direct sunlight for approximately one week in order to get a fibre with no moisture content.
Physico-chemical analysis
The FSLF diameter was measured in four random places through an optical microscope and was compared in . The sample was analyzed for cellulose, hemicelluloses, and lignin to explore typical chemical content in the fiber. The cellulose, hemicelluloses, and lignin content were estimated through enough Kurshner-Hoffer method, NFT 12–008, APPITA P11s-78 standard method (Sreenivasan et al. Citation2011). The fibre’s density was analyzed by pycnometer and its moisture content was estimated using an electronic moisture tester (Sartorious, MA45). Conrad’s methodology was employed to find the wax percentage of the sample (Conrad Citation1944). The ash content present in the sample was determined using ASTM E1755–01 standard procedure (Saravana Kumaar et al. Citation2019).
Table 1. Relating FSLF’s physio-chemical, mechanical, crystalline, and thermal properties with other investigated fibers.
Fourier transform infrared analysis
The functional group distribution of the fiber sample area was evaluated by FT-IR (Shimadzu UV-1280) spectroscopy. A sample of powdered fibre was blended with potassium bromate, and then pelletized. The reaction was carried out using a spectral range between 500–4000 cm−1 along with a scan rate of 32 with an accuracy of 4 cm−1.
X-ray diffraction analyses
The crystallinity of the powdered sample to discover its crystalline nature and physical characteristics was analyzed by using a Bruker D2 Phaser diffractometer. This study used an intensity of wavelength of 0.154 mm with an operating range and step size of 10- 80°, 0.05°, respectively. From spectra, the crystallinity index (CI) and crystalline size (CS) fiber were computed (Segal et al. Citation1958) using Equationequation (1)(1)
(1) & Equationequation (2)
(2)
(2) .
Where I200 is the highest intensity of 200 lattice plane and Iam is an intensity of non- crystalline material (Indran, Edwin Raj, and Sreenivasan Citation2014)
In this equation, k is the Scherrer constant (0.89), ß is peak’s full width at half maximum radians, λ is the wavelength (0.1541 nm), and Bragg angle is represented as θ.
Nuclear magnetic resonance analyses
To determine the chemical makeup of natural fiber, NMR spectroscopy was conducted. It is done with the exposure of 13c nuclei on the cross-polarization mode at a frequency of 75.46 MHz along with an operating spinning rate of 10 kHz was used to avoid repeated absorption.
Thermogravimetric analysis
TGA (STA 449, NETZSCH, Germany) analysis of fiber was performed in this study to evaluate its thermal stability. A crucible was used to melt the fibre at the temperature from 10°- 600°C with a heating rate of 10°C/min. The nitrogen gas flow rate was kept constant at 20 ml/min to remain in an inert environment. During the process the rate change found in the material mass was calculated in terms of kinetic activation energy (EA) as per broido’s Equationequation (3)(3)
(3)
Here, T refers to the temperature in kelvin, y denotes normalized weights (wt/wi), i.e, wt specify sample weight at time t and wi symbolize initial sample weight K is constant of reaction rate, and R (8.32 J/mol) is the universal gas constant (A N and K J Citation2017).
Scanning electron microscope analysis
The longitudinal surface examination of the extracted fiber was investigated through SEM (ZEISS Gemini 360) at different magnifications. The analysis was accomplished in a beam of an electron with a speed of 3 kV. The specimens were platinum-plated to avoid the electron-beam charge.
Atomic force microscopy Analysis
AFM was employed to examine the surface roughness of the sample at a resolution to nano-scale. The roughness parameters were evaluated using AFM (Park XE-70) with scanner scale range of 10 × 10 μm in x,y-direction and at a resolution of 7 μm was used.
Energy dispersive X-Ray spectroscopy analysis
The elements contained on the exterior surface of the fiber were determined using an EDX (INCAPentaFET83) coupled to an SEM machine. The element distribution was observed at five distinct spots on the surface of the fibre, and corresponding average values were taken.
Results and discussion
Anatomy of FSLF fiber
The leaf cross-section viewed under an optical microscope () revealed that it possesses a circular shape with approximately 10 cm diameter. Different types of cells are aggregated in three layers epidermis, mesodermis, and endodermis. The epidermal layer consists of compactly arranged thick-walled cells mounted with cuticles. Mesodermis of FSLF was filled with large, circular, thin parenchyma cells referred to as ground tissue. There were five vascular bundles seen in the mesodermis, which are arranged in an arc shape. The bundles were collateral with a thick outer circular mass cup of sclerenchyma cells, the middle mass of phloem, and the inner conical segment of the phloem. The phloem is constituted by sieve tubes and parenchyma cells. The central axis is rich with loosely arranged parenchyma cells, which are termed as pith. The fibers are enclosed with xylem elements of the peduncle are originated from the secretory cavity. However, the diameter and strength of the fibre largely depend upon the maturation, aging, and environmental circumstances of the plant (Indran and Raj Citation2015).
Physical characteristics of FSLF
The density analysis revealed that FSLF possesses a low density of 810 kg/m3 which was advantageous than Sansevieria cylindrical (915 kg/m3), Sansevieria ehrenbergii (887 kg/m3), Tamarindus Indica L (1020–1270 kg/m3), Passiflora foetida (1828 kg/m3), etc. The density also lesser than that of glass (1400 kg/m3) and carbon fiber (1400–1820 kg/m3). An average diameter of FSLF was found to be 11.5 μm. compares the physical attributes of natural fibers to those of other synthetic and natural fibres. Resulted low density and the diameter of FSLF show its suitability for the production of lightweight materials.
Chemical properties of FSLF
Chemical constituents
The concentration of various constituents of FSLF is depicted in in comparison with other promising synthetic and natural fibers. Generally, fibers with high cellulose content and low hemicellulose content encounter good tensile properties. Since higher cellulose levels increase crystallinity and thermal stability while high hemicellulose content is responsible for easy degradation (Indran, Edwin Raj, and Sreenivasan Citation2014). The result indicates that FSLF with high cellulose percentage (70.1%) and low hemicellulose fraction (11.52%) pointed toward its good strength for reinforcement. The low lignin fraction (12.8%) observed in FSLF is advantageous in the context of high Lignin content retaining moisture that leads to the disintegration of composites (Somasundaram et al. Citation2022). The other imperative properties of FSLF are low wax (0.25%), ash (4.66%), and moisture content (5.12%). Fibres with low wax content ensure smoothness and roughness of the surface that is necessary for opposite adhesion between fibre and matrix (Sanjay et al. Citation2019). Likewise low ash content and moisture content of FSLF disclosed that the fibre possesses good quality and lifespan. Moreover, these suitable qualities of FSLF are supported by the findings of the following session.
Fourier transform infrared
The eight well-defined absorption peaks of the FT-IR spectrum represent various functional groups of cellulose, hemicelluloses, and lignins present in FSLF (). The extensive peak at 3780 cm−1 narrates the hydrogen-bonded O-H stretching of FSLF that is inclined by the hydroxyl group present in α cellulose (Jabli et al. Citation2018; Raja et al. Citation2019). The vibrational spectra at 2935 and 2844 cm−1 are assigned by – CH2OH and – CH2 stretching that intended the occurrence of cellulose, hemicelluloses, respectively (Paiva et al. Citation2007; Tka et al. Citation2018). The existence of OH bending at 1643 cm−1 also encodes the hemicellulose fraction of FSLF (Sebeia et al. Citation2019). A sharp band at 1508 cm−1 is accredited to C=C vibration intended by the presence of lignin (Jabli et al. Citation2017). A weak intensity peak 1450 cm−1 is qualified for CH2 bending due the cellulose existence (Belouadah, Ati, and Rokbi Citation2015). A bending frequency of C-O and O-H at 1023 cm−1 indicates the occurrence of lignin in the fiber (Raja et al. Citation2021b; Sanjay et al. Citation2019). The observed peaks along with the chemical constituents observed are listed in .
Table 2. FTIR peak observed for FSLF.
Xrd
The XRD analysis of FSLF is displayed in . Generally, the cellulose crystals orientation in the fiber to fibre axis influences crystallinity index, and crystalline size measures the condensed surface area of the fibre (Divya et al. Citation2022). Often these properties are allied with good tensile strength and reduced moisture absorption capacity. The X-ray diffractogram of FSLF displays two defined peaks at the lattice plane (2θ). The peak at 15° (110) indicates the amorphous phase of the fibre, whereas the high peak intensity at 22.2° (002) occurred due to the presence of crystalline cellulose phase. Following that, crystalline index (CI) and crystallite size (CS) of the fibre were calculated as 56.7% and 27.20 nm. The crystallinity index and crystalline size of FSLF was greater than that of Tamarindus Indica (55%, 5.73 nm), Ricinus communis (48.8%, 4.8 nm), Passiflora foetida (67.36%,4.23 nm), Dracaena reflexa (57.32%,19 nm), Coccinea grandis (52.1%, 13.3 nm), Saharan aloevera (56.5%,5.72 nm), banyan tree (6.28 nm), Tridaxprocumbens (34.4%, 25.75 nm) (Raja et al. Citation2021c; Sanjay et al. Citation2019). The observed crystalline properties of FSLF are strong enough to consider the fibre for composite reinforcements.
13C (CP-MAS) NMR
The FSLF nuclear magnetic resonance spectrum with high-resolution showed several peaks that correspond to various constituents present in the fiber (.). The acetyl groups of hemicellulose inclined with the first peak at 23.2 ppm (C1) in the spectra. Followed by the peak at 66.6 ppm (C6) indicate the occurrence of amorphous cellulose in the sample (Manimaran et al. Citation2020). The resonance peaks assigned between 73.7–75.5 ppm are associated with the C2 C3 and C5 carbon of carbon rings belonging to cellulose (Moshi et al. Citation2020). The carbon endorsed with the crystalline domain of cellulose FSLF was recognized between the peaks 84–89.7 ppm (C4) (Manimaran et al. Citation2018). A moderate peak located at 106 ppm is allotted to the anomeric carbon (C1) of cellulose present in the fibre. Consequent peaks ranging from 128–143 ppm represent aromatic carbons of lignin content in the material. This interpretation has been made through the earlier reports that had shown similar patterns for coconut fibre, cotton fibre, and Furcraea Foetida, sisal fibre (Moshi et al. Citation2020).
Thermal characteristics of FSLF
Generally, fibers with high thermal stability are most important in the forecast of high-temperature processing and application. The outcome of TGA is depicted in . The TGA and DTG (derivative of thermogravimetric) curves clearly present the degradation pattern of FSLF constituents. The first phase of degradation started at 65°C, which was due to the result of moisture loss. This followed by extensive degradation started from 170°C that represents hemicellulose degradation (Indran and Raj Citation2015). A considerable mass reduction at 250°C to 380°C was seen due to burn out of cellulose constituents, where the major peek at 350°C corresponds to extensive degradation of α cellulose (Manimaran et al. Citation2019). The subsequent peaks up to 509°C are due to the combined degradation of wax, lignin, and other components of FSLF. Therefore it is established from the TGA-DTA curves plot that FSLF endorses thermal stability of 350°C and a maximum degradation temperature of 509°C. Concurrent studies also reported similar thermal stability are Cissusquadrangularis stem (328.9°C), Furcraea Foetida (320°C), Passiflora foetida(320°C), Ficusreligiosa (325°C). The Kinetic activation energy is calculated in terms to establish minimal energy required for degradation under specific temperatures. The kinetic energy required for natural fibre is specified in a span of 60–170 KJ/mol (Saravanakumar et al. Citation2013). As per broido’s plot, kinetic activation energy (Ea) of FSLF is derived as 73.2 kJ/mol, confirming its stability to withstand the high temperature required for polymerization process during reinforcement (Indran and Raj Citation2015; Moshi et al. Citation2020).
Single fiber test
The tensile strength, Young’s modulus, and strain to the failure rate of raw FSLF were established through a single fiber tensile test (). A sample fibre with a 40 mm gauge length provided good tensile strength 468.32 ± 6.14MPa and Young’s modulus of 11.23 ± 1.54GPa in comparison with other gauge lengths. The tensile strength was more promising than that of typical potential fibers as Furcraea Foetida (623MPa), Sansevieria Ehrenbergii (585MPa), Ficus religiosa (433.32MPa), Red banana Peduncle (440MPa), Cocciniagrandis stem (424.2MPa), Cymbopogon flexuosus stem (419 ± 52.56MPa), Borassus fruit fibre (175.52MPa), while Young’s modulus was more significant than Furcraea Foetida (6.52GPa), Sansevieria Ehrenbergii (7.67GPa), Ficus religiosa (5.42GPa), Cissusquadrangularis root (7.04GPa), Sansevieria cylindrical (6.72GPa) Red banana Peduncle (1.8GPa). The sample shows the low strain to failure of 4.17% at a gauge length of 40 mm, which was significant over Tamarindus Indica L (6.42%), Saharan Aloevera (11.1%), Furcraea Foetida (10.322%), Coccinea grandis fibre (16%). These significant properties were enough to justify its use as a reinforcing material in composite manufacture.
Table 3. FSLF mechanical properties.
Surface morphology
The SEM and AFM analyses are excellent methods to determine the surface morphology of fibers. The SEM micrograph reveals the presence of microvoids and shallow pores that increases the interfacial bonding of fibre- matrix during composite framing () (Fiore, Scalici, and Valenza Citation2014). The extensive surface roughness was noted from the figure which provides increased contact surface area for matrix binding. The hemicellulose, starch grains, and wax constituents of the fibre belonging to the white portions displayed in the figure can be removed through pretreatment before reinforcement (Indran and Raj Citation2015; Sundaram et al. Citation2021). The microfibril angle (α) calculated from the image was 4.23°± 3.02 which was lower than that of Cissusquadrangularis stem (4.95°), Cissusquadrangularis root (5.15°), Prosopisjuliflora (10.64°), banana (11-12°), etc. It is also reported that lower microfibrils angle intends better mechanical properties in the fibre.
Atomic force microscopy is a promising method for determining the surface roughness of the fiber. 2D, 3D, line profile, and roughness parameters of FSLF are exposed in . An average surface roughness (Ra) 22.70 nm was more than Furcraea Foetida (18 nm), Acacia planifrons (0.708 nm), Dracaena reflexa (22.69 nm), Sida Cordifolia (6.71 nm), Chlorisbarbata (0.894 nm) (Senthamaraikannan and Kathiresan Citation2018). High surface roughness is linked with less surface impurity, which is a favorable property for fibre reinforcement. The negative surface skewness (Rsk) value of −0.416 was obtained that once again confirmed the porous nature of fibre surface (Babu et al. Citation2022). Roughness kurtosis (Rku) is another measure that determines the spiky and rough surface when the value is below or above 3. The Rku of FSLF was able to confirm the surface roughness with the value of 9.32. The other parameters as (Rrms/Rq) root mean square value, peak to valley height (Rt) (330.11 nm), and ten-point average roughness (Rz) (208.30 nm) distribution were calculated as 1.30 which also validate the favorable surface roughness of the fibre. (e) and depicts the chemical element distribution on the surface of FSLF obtained through EDX analysis in term of weight and atomic percent. Carbon, oxygen, and silica peaks were seen. The FSLF has traces of antimony, zirconium, and barium (less than 1 wt percent).
Figure 5. FSLF Fibre (a) 3D roughness surface texture, (b) 2D roughness surface texture, (c) 2D roughness measurement, (e) roughness parameters, and (f) EDX analysis.
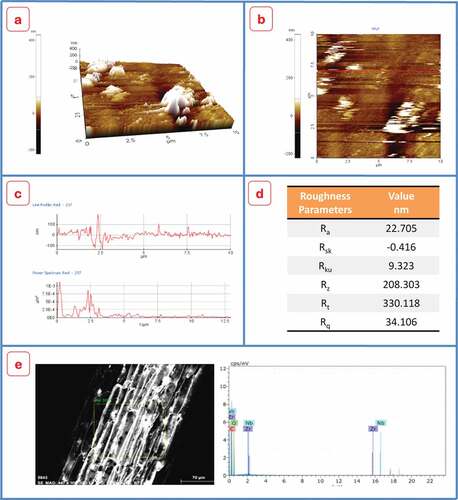
Table 4. FSLF’s EDX elemental composition compared to other natural fibers (Manimaran et al. Citation2019; Moshi et al. Citation2020).
Conclusion
The leaf fiber of Furcraea selloa K.Koch is a unique natural lignocellulosic fibre that can be extracted mechanically. The anatomical study on FSLF reveals a dense network of fibre cells that are oriented and bonded together by non-cellulosic components. SEM micrographs and AFM identify the roughness surface of the fibre which is well suitable for better adherence in the matrix polymer. The chemical, X-ray, and FTIR spectroscopic analyses indicated that the FSLF has a high concentration of cellulose (70.01%), with a CI of 56.7% respectively, comparable to other lignocellulosic fibers. The TGA enumerates FSLF are thermally stable upto 350°C and had 73.2 kJ/mol activation energy enabling the extrusion of polymer-based composites. Single fibres had Young’s modulus of 11.23 ± 1.54 GPa, a tensile strength of 468.32 ± 6.14 MPa, and a strain at failure of 4.17 ± 0.15% owing to their good potential to be a novel alternative for the preparation of high-performance composites. FSLF desirable reinforcement properties and nonpolluting nature create the dimension quality of the composite as reminds the quality of particular material of small scale to large-scale application.
Declaration of interest
Ethical Approval
Not Applicable
Competing interests
The author(s) declared no competing interest with respect to the research, authorship, and/or publication of this article.
Availability of data and materials
The data that support the findings of this study are available on request from the corresponding author. The data are not publicly available due to privacy or ethical restrictions.
Authors' contributions
S.Indran - Conceptualization, Investigation, Methodology, Writing Original Draft, Visualization and Data Curation, Project Administration, Supervision.
D.Divya – Resources, Formal analysis, Validation, Writing - Review & Editing.
S.Raja - Visualization and Supported for data interpretation.
M.R.Sanjay – Resources, Formal analysis, Validation.
Suchart Siengchin – Resources, Complete analysis of the research work
Acknowledgement
First author acknowledges RadoChemMAX, Nagercoil for providing research support to carry out this review work. The authors also acknowledge the Natural Composites Research Group Lab, Department of Materials and Production Engineering, The Sirindhorn International Thai-German Graduate School of Engineering, King Mongkut’s 13 University of Technology North Bangkok, Bangkok-10800, Thailand. for their support to complete this research work.
Additional information
Funding
References
- Amutha, V., and B. Senthilkumar. 2021. Physical, chemical, thermal, and surface morphological properties of the bark fiber extracted from acacia concinna plant. Journal of Natural Fibers 18 (11):1661–17. Taylor & Francis. doi:10.1080/15440478.2019.1697986.
- A N, B., and N. K J. 2017. Characterization of alkali treated and untreated new cellulosic fiber from Saharan aloe vera cactus leaves. Carbohydrate polymers 174:200–08. Elsevier Ltd. doi:10.1016/j.carbpol.2017.06.065.
- Babu, B. G., D. Princewinston, S. S. Saravanakumar, A. Khan, P. V. Aravind Bhaskar, S. Indran, and D. Divya. 2022. Investigation on the physicochemical and mechanical properties of novel alkali-treated phaseolus vulgaris fibers. Journal of Natural Fibers 19 (2):770–81. doi:10.1080/15440478.2020.1761930.
- Baskaran, P. G., M. Kathiresan, P. Senthamaraikannan, and S. S. Saravanakumar. 2018. Characterization of new natural cellulosic fiber from the bark of dichrostachys cinerea. Journal of Natural Fibers 15 (1):62–68. Taylor & Francis. doi:10.1080/15440478.2017.1304314.
- Belouadah, Z., A. Ati, and M. Rokbi. 2015. Characterization of new natural cellulosic fiber from lygeum spartum L. Carbohydrate Polymers 134:429–37. Elsevier Ltd. doi:10.1016/j.carbpol.2015.08.024.
- Bezazi, A., A. Belaadi, M. Bourchak, F. Scarpa, and K. Boba. 2014. Novel extraction techniques, chemical and mechanical characterisation of Agave americana L. natural fibres. Composites Part B: Engineering 66:194–203. Elsevier Ltd. doi:10.1016/j.compositesb.2014.05.014.
- Binoj, J. S., R. E. Raj, and S. Indran. 2018. Characterization of industrial discarded fruit wastes (Tamarindus Indica L.) as potential alternate for man-made vitreous fiber in polymer composites. Process Safety and Environmental Protection 116:527–34. Institution of Chemical Engineers. doi:10.1016/j.psep.2018.02.019.
- Conrad, C. M. 1944. Determination of wax in cotton ?ber: A new alcohol extraction method. Industrial and Engineering Chemistry 16 (12):745–48. doi:10.1021/i560136a007.
- Das, P. P., and V. Chaudhary. 2021. Moving towards the era of bio fibre based polymer composites. Cleaner Engineering and Technology 4:100182. Elsevier Ltd. doi:10.1016/j.clet.2021.100182.
- Dharmalingam, S., O. Meenakshisundaram, V. Elumalai, and R. S. Boopathy. 2020. An investigation on the interfacial adhesion between amine functionalized luffa fiber and epoxy resin and its effect on thermal and mechanical properties of their composites. Journal of Natural Fibers 18 (12):1–16. Taylor & Francis. doi:10.1080/15440478.2020.1726238.
- Divya, D., I. Suyambulingam, M. R. Sanjay, and S. Siengchin. 2022. Suitability examination of novel cellulosic plant fiber from Furcraea selloa K. Koch peduncle for a potential polymeric composite reinforcement. Polymer Composites 43 (7):1–21. doi:10.1002/pc.26683.
- Fatma, T., and S. Jahan. 2018. Extraction of unconventional bast fibers from kydia calycina plant and their characterisation. Journal of Natural Fibers 15 (5):697–706. Taylor & Francis. doi:10.1080/15440478.2017.1354745.
- Fiore, V., T. Scalici, and A. Valenza. 2014. Characterization of a new natural ?ber from Arundo Donax L. as potential reinforcement of polymer composites. Carbohydrate Polymers 106:77–83. Elsevier Ltd. doi:10.1016/j.carbpol.2014.02.016.
- Ganapathy, T., R. Sathiskumar, P. Senthamaraikannan, S. S. Saravanakumar, and A. Khan. 2019. Characterization of raw and alkali treated new natural cellulosic fibres extracted from the aerial roots of banyan tree. International Journal of Biological Macromolecules 138: 573–81. Elsevier B.V. doi:10.1016/j.ijbiomac.2019.07.136.
- Gedik, G. 2021. Extraction of new natural cellulosic fiber from Trachelospermum jasminoides (star jasmine) and its characterization for textile and composite uses. Cellulose 28 (11):6899–915. Springer Netherlands. doi:10.1007/s10570-021-03952-1.
- Indran, S., R. Edwin Raj, and V. S. Sreenivasan. 2014. Characterization of new natural cellulosic fiber from Cissus quadrangularis root. Carbohydrate polymers 110:423–29. Elsevier Ltd. doi:10.1016/j.carbpol.2014.04.051.
- Indran, S., and R. E. Raj. 2015. Characterization of new natural cellulosic fiber from Cissus quadrangularis stem. Carbohydrate polymers 117:392–99. Elsevier Ltd. doi:10.1016/j.carbpol.2014.09.072.
- Jabli, M., N. Tka, K. Ramzi, and T. A. Saleh. 2018. Physicochemical characteristics and dyeing properties of lignin-cellulosic fibers derived from Nerium oleander. Journal of molecular liquids 249:1138–44. doi:10.1016/j.molliq.2017.11.126.
- Jabli, M., N. Tka, G. A. Salman, A. Elaissi, N. Sebeia, and M. Hamdaoui. 2017. Rapid interaction, in aqueous media, between anionic dyes and cellulosic nerium oleander fibers modified with ethylene-diamine and hydrazine. Journal of molecular liquids 242:272–83. Elsevier B.V. doi:10.1016/j.molliq.2017.07.018.
- Jebadurai, S. G., R. E. Raj, V. S. Sreenivasan, and J. S. Binoj. 2019. Comprehensive characterization of natural cellulosic fiber from Coccinia grandis stem. Carbohydrate Polymers 207:675–83. Elsevier Ltd. doi:10.1016/j.carbpol.2018.12.027.
- Kathirselvam, M., A. Kumaravel, V. P. Arthanarieswaran, and S. S. Saravanakumar. 2019. Characterization of cellulose fibers in Thespesia populnea barks : Influence of alkali treatment. Carbohydrate Polymers 217:178–89. doi:10.1016/j.carbpol.2019.04.063.
- Maache, M., A. Bezazi, S. Amroune, F. Scarpa, and A. Dufresne. 2017. Characterization of a novel natural cellulosic fiber from Juncus effusus L. Carbohydrate Polymers 171:163–72. Elsevier Ltd. doi:10.1016/j.carbpol.2017.04.096.
- Manimaran, P., G. P. Pillai, V. Vignesh, and M. Prithiviraj. 2020. Characterization of natural cellulosic fibers from nendran banana peduncle plants. International Journal of Biological Macromolecules 162:1807–15. Elsevier B.V. doi:10.1016/j.ijbiomac.2020.08.111.
- Manimaran, P., S. P. Saravanan, M. R. Sanjay, S. Siengchin, M. Jawaid, and A. Khan. 2019. Characterization of new cellulosic fiber: Dracaena reflexa as a reinforcement for polymer composite structures. Journal of Materials Research and Technology 8 (2):1952–63. Brazilian Metallurgical, Materials and Mining Association. doi:10.1016/j.jmrt.2018.12.015.
- Manimaran, P., P. Senthamaraikannan, M. R. Sanjay, M. K. Marichelvam, and M. Jawaid. 2018. Study on characterization of Furcraea foetida new natural fiber as composite reinforcement for lightweight applications. Carbohydrate Polymers 181:650–58. Elsevier Ltd. doi:10.1016/j.carbpol.2017.11.099.
- Mayandi, K., N. Rajini, P. Pitchipoo, J. T. W. Jappes, A. V. Rajulu, et al. 2016. Extraction and characterization of new natural lignocellulosic fiber Cyperus pangorei. International Journal of Polymer Analysis and Characterization 21 (2):175–83. doi:10.1080/1023666X.2016.1132064.
- Moshi, A. A. M., D. Ravindran, S. R. S. Bharathi, S. Indran, S. S. Saravanakumar, and Y. Liu. 2020. Characterization of a new cellulosic natural fiber extracted from the root of Ficus religiosa tree. International Journal of Biological Macromolecules 142: 212–21. Elsevier B.V. doi:10.1016/j.ijbiomac.2019.09.094.
- Paiva, M. C., I. Ammar, A. R. Campos, R. B. Cheikh, and A. M. Cunha. 2007. Alfa fibres : Mechanical, morphological and interfacial characterization. Composites Science and Technology 67 (6):1132–38. doi:10.1016/j.compscitech.2006.05.019.
- Pandit, P., M. D. Teli, K. Singha, S. Maiti, and S. Maity. 2021. Extraction and characterization of novel Sterculia foetida fruit shell fibre for composite applications. Cleaner Engineering and Technology 4:100194. Elsevier Ltd. doi:10.1016/j.clet.2021.100194.
- Prithiviraj, M., and R. Muralikannan. 2020. Investigation of optimal alkali-treated perotis indica plant fibers on physical, chemical, and morphological properties. Journal of Natural Fibers 00: 1–14. Taylor & Francis. doi:10.1080/15440478.2020.1821291.
- Raja, S., R. Rajesh, S. Indran, D. Divya, and G. Suganya Priyadharshini. 2021a. Characterization of industrial discarded novel Cymbopogon flexuosus stem fiber: A potential replacement for synthetic fiber. Journal of Industrial Textiles 51 (1_suppl):1207S–34S. doi:10.1177/15280837211007507.
- Raja, S., R. Rajesh, S. Indran, D. Divya, and G. Suganya Priyadharshini. 2021b. Characterization of industrial discarded novel Cymbopogon flexuosus stem fiber: A potential replacement for synthetic fiber. Journal of Industrial Textiles 51 (1_suppl):1–28. doi:10.1177/15280837211007507.
- Raja, S., R. Rajesh, S. Indran, D. Divya, and G. Suganya Priyadharshini. 2021c. Characterization of industrial discarded novel Cymbopogon flexuosus stem fiber: A potential replacement for synthetic fiber. Journal of Industrial Textiles 51 (1_suppl):1207 S–34S. doi:10.1177/15280837211007507.
- Raja, S., M. Ravichandran, R. S. R. Isaac, and N. Abilash. 2019. A review: Sources of silica from agro waste and its extraction methods. Journal of Advanced Research in Dynamical and Control Systems 11:765–72.
- Rajeshkumar, G., G. L. Devnani, J. P. Maran, M. R. Sanjay, S. Siengchin, N. A. Al-Dhabi, and K. Ponmurugan. 2021. Characterization of novel natural cellulosic fibers from purple bauhinia for potential reinforcement in polymer composites. Cellulose 28 (9):5373–85. Springer Netherlands. doi:10.1007/s10570-021-03919-2.
- Raju, J. S. N., M. V. Depoures, J. A. B. I. H. U. L. L. A. Shariff, and S. U. D. H. I. R. Chakravarthy. 2021. Characterization of natural cellulosic fibers from stem of symphirema involucratum plant. Journal of Natural Fibers 00: 1–16. Taylor & Francis. doi:10.1080/15440478.2021.1875376.
- Ramesh Babu, S., and G. Rameshkannan. 2021. Aerial root fibres of ficus amplissima as a possible reinforcement in fibre-reinforced plastics for lightweight applications: physicochemical, thermal, crystallographic, and surface morphological behaviours. Journal of Natural Fibers 00:1–16. Taylor & Francis. doi:10.1080/15440478.2021.1958422.
- Sanjay, M. R., P. Madhu, M. Jawaid, P. Senthamaraikannan, S. Senthil, and S. Pradeep. 2018. Characterization and properties of natural fiber polymer composites: A comprehensive review. Journal of Cleaner Production 172:566–81. Elsevier B.V. doi:10.1016/j.jclepro.2017.10.101.
- Sanjay, M. R., S. Siengchin, J. Parameswaranpillai, M. Jawaid, C. I. Pruncu, and A. Khan. 2019. A comprehensive review of techniques for natural fibers as reinforcement in composites: Preparation, processing and characterization. Carbohydrate Polymers 207:108–21. doi:10.1016/j.carbpol.2018.11.083.
- Saravana Kumaar, A., A. Senthilkumar, T. Sornakumar, S. S. Saravanakumar, and V. P. Arthanariesewaran. 2019. Physicochemical properties of new cellulosic fiber extracted from Carica papaya bark. Journal of Natural Fibers 16 (2):175–84. Taylor & Francis. doi:10.1080/15440478.2017.1410514.
- Saravanakumar, S. S., A. Kumaravel, T. Nagarajan, P. Sudhakar, and R. Baskaran. 2013. Characterization of a novel natural cellulosic fiber from Prosopis juliflora bark. Carbohydrate Polymers 92 (2):1928–33. Elsevier Ltd. doi:10.1016/j.carbpol.2012.11.064.
- Sebeia, N., M. Jabli, A. Ghith, Y. El Ghoul, and F. M. Alminderej. 2019. Populus tremula, Nerium oleander and Pergularia tomentosa seed fibers as sources of cellulose and lignin for the bio-sorption of methylene blue. International Journal of Biological Macromolecules 121:655–65. Elsevier B.V. doi:10.1016/j.ijbiomac.2018.10.070.
- Segal, C. J. J., and M. Conrad. 1958. Empirical Method for Estimating the Degree of Crystallinity of Native Cellulose Using the X-Ray Diffractometer. Text. Res. J 29 (10):786–794.
- Segal, L., J. J. Creely, A. E. Martin, and C. M. Conrad. 1959. An empirical method for estimating the degree of crystallinity of native cellulose using the x-ray diffractometer. Textile Research Journal 29 (10):786–94. doi:10.1177/004051755902901003.
- Senthamaraikannan, P., and M. Kathiresan. 2018. Characterization of raw and alkali treated new natural cellulosic fiber from Coccinia grandis.L. Carbohydrate Polymers 186:332–43. Elsevier Ltd. doi:10.1016/j.carbpol.2018.01.072.
- Soma Sundaram Pillai, R., R. Rajamoni, I. Suyambulingam, I. Rajamony Suthies Goldy, and D. Divakaran. 2021. Synthesis and characterization of cost-effective industrial discarded natural ceramic particulates from Cymbopogon flexuosus plant shoot for potential polymer/metal matrix reinforcement. Polymer Bulletin 79 (10):8765–806. Springer Berlin Heidelberg. doi:10.1007/s00289-021-03913-5.
- Somasundaram, R., R. Rajamoni, I. Suyambulingam, D. Divakaran, S. Mavinkere Rangappa, and S. Siengchin. 2022. Utilization of discarded Cymbopogon flexuosus root waste as a novel lignocellulosic fiber for lightweight polymer composite application. Polymer Composites 43 (5):2838–53. doi:10.1002/pc.26580.
- Sreenivasan, V. S., S. Somasundaram, D. Ravindran, V. Manikandan, and R. Narayanasamy. 2011. Microstructural, physico-chemical and mechanical characterisation of Sansevieria cylindrica fibres – an exploratory investigation. Materials & Design 32 (1):453–61. Elsevier Ltd. doi:10.1016/j.matdes.2010.06.004.
- Sundaram, R. S., R. Rajamoni, I. Suyambulingam, and R. Isaac. 2021. Comprehensive characterization of industrially discarded cymbopogon flexuosus stem fiber reinforced unsaturated polyester composites: effect of fiber length and weight fraction. Journal of Natural Fibers 19 (13):1–16. Taylor & Francis. doi:10.1080/15440478.2021.1944435.
- Teixeira, E. D. M., A. C. Corre, A. Manzoli, F. D. L. Leite, L. Henrique, and C. Mattoso. 2010. Cellulose nanofibers from white and naturally colored cotton fibers. cellulose 17 (3):595–606. doi:10.1007/s10570-010-9403-0.
- Tka, N., M. Jabli, T. A. Saleh, and G. A. Salman. 2018. Amines modified fibers obtained from natural Populus tremula and their rapid biosorption of Acid Blue 25. Journal of molecular liquids 250:423–32. Elsevier B.V. doi:10.1016/j.molliq.2017.12.026.
- Vijay, R., D. Lenin Singaravelu, A. Vinod, M. R. Sanjay, S. Siengchin, M. Jawaid, A. Khan, and J. Parameswaranpillai. 2019. Characterization of raw and alkali treated new natural cellulosic fibers from Tridax procumbens. International Journal of Biological Macromolecules 125:99–108. Elsevier B.V. doi:10.1016/j.ijbiomac.2018.12.056.