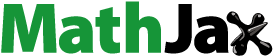
ABSTRACT
The significant environmental pollution caused by synthetic fibers drew researchers’ attention to the development of eco-friendly reinforcement for composite products. This study seeks to identify an alternative novel natural fiber from the Cryptostegia Grandiflora (CG) plant as a reinforcement material for bio composites. The fiber chemical compositions, X-ray diffraction, Fourier Transform Infrared spectroscopy (FTIR), Scanning Electron Microscopy, Thermo Gravimetric Analysis, and tensile test for single fiber are all examined. The fiber had a cellulose content of 79.20% and a crystallinity index of 62%. Thermo Gravimetric Analysis confirms that Cryptostegia Grandiflora fiber (CGF) can withstand temperatures as high as 230°C. It has a density of 1.02 g/cc and a tensile strength of 791 MPa on average. This novel Cryptostegia Grandiflora fiber will undoubtedly be used as reinforcement material in bio-composite materials.
摘要
合成纤维造成的严重环境污染引起了研究人员对开发复合材料产品的环保增强材料的关注. 本研究旨在确定一种新的天然纤维,作为生物复合材料的增强材料. 对单纤维的化学成分、X射线衍射、傅里叶变换红外光谱(FTIR)、扫描电子显微镜、热重分析和拉伸试验进行了检查. 该纤维的纤维素含量为79.20%,结晶度指数为62%. 热重分析证实,大花隐球菌纤维(CGF)可以承受高达230°C的温度. 其密度为1.02 g/cc,平均抗拉强度为791MPa. 这种新型的黄花隐球菌纤维无疑将被用作生物复合材料的增强材料.
Introduction
The last three decades have been the golden era of material development in engineering applications, with the use of synthetic fibers increasing in composite products; it provides good strength and a significant contribution to ancillary products. Now that we know it causes high carbon emissions, we are focusing on developing eco-friendly composite products for engineering applications. As a result, natural fiber has the potential to replace synthetic fiber in composite industries while also being biodegradable (Belaadi et al. Citation2014; Jawaid et al. Citation2014; Kommula et al. Citation2013; Reddy et al. Citation2014; Sudhakara et al. Citation2013).
Composites are classified into three types: polymer matrix, metal matrix, and ceramic matrix composites. Due to its advantageous qualities, such as strength, durability, and elasticity, synthetic fiber is reinforced with a matrix in polymer matrix composites. However, synthetic fiber is non-biodegradable and releases more carbon into the environment. As a result, much research is being conducted on environmentally friendly natural fibers as suitable substitutes for man-made fibers in polymer matrix composites (Jayaramudu et al. Citation2014; Reddy et al. Citation2012).
Usually, natural fibers are taken from several parts of the plant including, seeds, stems, and leaves (Thakur, Kumari Thakur, and Kumar Gupta Citation2014). The geological aspect of the plant determines the chemical composition of the fibers, which influences the plant fiber’s properties (Madhu et al. Citation2019). The percentage of cellulose in natural fiber determines its strength (Kumar and Singha Citation2010). Natural fiber-reinforced composite properties are based on fiber selection and the matrix utilized during fabrication (Vijay et al. Citation2022). Surprisingly, the modulus of some natural fibers is higher than that of manmade fibers (Kommula et al. Citation2013). Many researchers and scientists are working to develop novel fibers with reinforcement polymer matrices that are used in aircraft, automobiles, sports, food packaging, and construction (Reddy et al. Citation2015).
Natural fibers are hydrophilic because they are made up of cellulose and hemicellulose layers. Natural fibers’ inherent hydrophilicity makes it possible for them to absorb moisture. The hydrophilic property reduces the interaction adhesion of reinforcement with resin, lowering composite performance (Asyraf et al. Citation2022; Bahrain et al. Citation2022).
In the United States of America, composite construction materials for building construction are made from a straw. Many auto parts are made of natural composites based on fibers like flax, jute, and sisal. The interior door panel of the Mercedes-Benz S-Class was made in Germany in 1999 with 35% Baypreg F semi-rigid (PUR) elastomer and 65% flax, jute, and sisal blend. An epoxy matrix containing hemp was also used in the door panel products of Mercedes-Benz E-Class vehicles.
Toyota Motor Corporation has developed an eco-plastic material made from sugar cane for car interior panels. Audi AG introduced polyurethane-reinforced eco-friendly door panels made of a flax/sisal composite in 2000. Natural fiber plays an important role in the creation of green composites in the twenty-first century, so researchers must lay a solid foundation in order to create new opportunities for natural fiber composites (Sanjay et al. Citation2016).
This study aims to extract a novel potential fiber from the stems of the plant Cryptostegia Grandiflora and investigate its properties for reinforcement in biocomposites. Cryptostegia Grandiflora is a rubber vine native to southwest Madagascar that belongs to the Apocynaceae family. As a shrub, a Cryptostegia Grandiflora can grow up to 2 meters (6 feet) tall, but as a vine, it can grow up to 30 meters (100 feet) long with the support of another plant. Cryptostegia Grandiflora plants are predicted to live up to 80 years. It can produce over 8000 seeds in a single reproductive cycle and produce seeds twice every year. It is widespread in worldwide but it is eradicated as it is an invasive species (Bekele, Seifu, and Ayenew Citation2019; Luizza et al. Citation2016; Rodríguez-Estrella et al. Citation2010). The purpose of this study is to identify the fundamental characteristics of Cryptostegia Grandiflora fiber using chemical composition analysis, X-ray diffraction analysis (XRD), Fourier transforms infrared spectroscopy (FTIR), Scanning Electron Microscopy (SEM), thermal analysis, and a single fiber tensile test.
Materials and methods
Fiber extraction
The CG plant stems are obtained in the Virudhunagar District of Tamil Nadu, India. The CG plant stem has a length of 6–10 feet. The stems are cut to the desired length with a knife before being manually peeled for fiber extraction. These peeled parts are immersed in water at room temperature for 7–10 days. The peeling parts become wet during the water-retting process. Since gum is present in the extracted fibers, they are roughly washed to eliminate unwanted particles. The bundles of dried fibers are collected for further research after they are dried in sunlight for at least 2–4 days to reduce the moisture content. The extracted processes of CG fibers are shown in .
Physical and chemical analysis
This study evaluates the percentage values of hemicellulose, cellulose, wax, ash, moisture content, pectin, lignin, density, and diameter. The weight percentage of cellulose is calculated using Kurcshner and Hoffer’s method, while the percentage of hemicellulose is measured using the NFT 12–008 standard. CGF’s lignin content is calculated using the APPITA P11s-78 method. The ash and moisture content is determined using the IS 199 method. The Conrad method is used to determine the wax percentage. The fiber density of Cryptostegia Grandiflora is calculated using the ASTM D 2320–98 (2003) standard (Manimaran et al. Citation2018). The apparent fiber diameter is measured using a Leica DM750 M optical microscope. A total of 30 specimens are used to account for natural fiber variability, and an average value is reported.
X-ray diffraction analysis (XRD)
To determine the CGF’s phase identification, an X-Ray diffraction analyzer with a monochromatic intensity of CuKα radiation is used. The crystallinity index (CI) is calculated using Equationequation 1(1)
(1) .
In Equationequation (1)(1)
(1) , H22.6 and H16.61 represent the values of the height of the peaks at 2θ = 22.6 and 2θ = 16.61. Scherrer’s formula is used to calculate the crystallinity size (CS).
In Equationequation (2)(2)
(2) , K is the Scherrer’s constant (0.89), the wavelength is the symbol of λ and β is the peak’s full width at half maximum (Hyness et al. Citation2018).
Fourier Transform Infrared Spectrometry (FTIR)
FTIR spectroscopy is performed to determine the functional groups in the chemical composition of the fiber. The spectrometer is kept at 30 degrees Celsius and 65% relative humidity. The spectrum is scanned at 32 scans per minute in the range of 4000–400 cm−1 with a resolution of 2 cm−1.
Surface morphological analysis
A Scanning electron microscope (SEM) test is performed by the Carl Zeiss model to study the surface morphology, surface roughness, cell wall structure, and other constituents of the Cryptostegia Grandiflora fiber. SEM images with different magnifications are useful in analyzing the surface morphology of fibers.
Thermal Analysis (TA)
Thermo Gravimetric analyzer is used to calculate the thermal stability of the Cryptostegia Grandiflora fiber on Perkin Elmer (STA 8000). The requisite amount of CGF is weighted and kept in an alumina crucible within a heating chamber. The temperature maintained for the fiber at a constant rate of 5°C/min from 30°C to 700°C in an inert atmosphere. Nitrogen gas is used as an inert environment. The weight loss in the fiber was observed when the temperature is raised (Narayanasamy et al. Citation2020).
Tensile test
To test the tensile strength of CGF, a 100 g load cell ZwickRoell Universal Testing Machine is used undergoing the ASTM D 3822–07 standards (Baskaran et al. Citation2018). The test is carried out at a temperature of 21°C and relative humidity of approximately 63%. A gauge length of 50 mm and a crosshead speed of 1 mm/min are used to test 30 single fibers and the average value is reported.
Result and discussion
Physical and chemical analysis
It is preferable to have a smaller diameter of fiber in order to get better mechanical properties (Ganapathy et al. Citation2019). The CGF’s diameter is 117 µm. The diameter of the fiber is mainly controlled by soil conditions, regional climatic conditions, plant age, and extraction methods (Premalatha et al. Citation2021; Vinod et al. Citation2020). The CGF’s diameter is measured using an optical microscope as shown in .
CGF has a lower density (1.02 g/cc) than flax (1.54 g/cc), hemp (1.47 g/cc), jute (1.44 g/cc), kenaf (1.2 g/cc), ramie (1.5 g/cc), sisal (1.45 g/cc), and bamboo (0.6–1.1 g/cc). CG fiber could lead to the development of lightweight composites (Célino et al. Citation2014). The CGF contains cellulose (79.20%), which provides good strength, stiffness, structural stability, and high hydrolysis resistance, which is higher than that of other natural fibers such as Abaca (56–63%), Flax (64–71%), Hemp (70.2%), Jute (61%), and Sisal (67–78%) (Alias et al. Citation2021; Raja et al. Citation2021; Vinod et al. Citation2020). CGF contains hemicellulose (17.20%), which acts as a reinforcing matrix between the cellulose microfibrils. It has hemicellulose content similar to Abaca, Flax, Hemp, and Ramie (Raja et al. Citation2021; Vinod et al. Citation2020). The lignin (2.50%) protects the plant against the biological attack and provides stiffness to the plant. When compared to sisal (1.5%) and curaua (1%) fibers, the wax percentage (0.54%) is low (Raja et al. Citation2021). While preparing the composites, helps with the interfacial adhesion between the matrix and fiber. Also, the CGF contains pectin (3.60%), moisture (10.20%), and ash (3.20%). The low percentage of ash increases the fire-resistant property of the fiber. These results show that CG fibers are useful for the fabrication of lightweight composite products. In , the chemical composition of CGF is documented.
Table 1. Chemical composition of Raw CG fibers.
XRD analysis
shows the X-ray diffractograms of CGF.
The crystalline peak is identified for CGF, the highest peak is found at 2θ = 22.6° whereas the lowest is at 2θ = 16.61°. As per the high peak, the fiber has a crystallographic plane of cellulose – I and the lower intensity proves the fiber contains a more amorphous region which includes amorphous cellulose, lignin, hemicellulose, and pectin (Mayandi et al. Citation2016). CGF has a calculated crystallinity index of 62%, which is higher than the crystallinity indexes of other natural fibers such as Calotropis gigantea (56.08%), Coir (57%), and Dracaena reflexa (57.32%). Also lower than Banyan tree aerial root (72.47%) and Hemp (87.87%). A high crystallinity index indicates crystalline nature, which is advantageous for using fiber as potential reinforcement in composites (Ganapathy et al. Citation2019; Raja et al. Citation2021; Vinod et al. Citation2020). The calculated crystallite size of Cryptostegia Grandiflora fiber is 20.6 nm.
FTIR analysis
In the FTIR analysis, the chemical functional group observed in the FTIR peak once again confirms the existence of cellulose, wax, hemicellulose and lignin. The peak intensities in the region of 613, 667, 896, 1061, 1161, 1240, 1371, 1431, 1647, 1742, 2887, 2940 and 3416 cm−1 are associated with the different significant modifications, according to this analysis. CGF’s, FTIR peaks are shown in .
The OH bonding of cellulose is attributed to the broad absorption peak at 613 cm−1 (Ramasamy, Obi Reddy, and Varada Rajulu Citation2018). The large absorption peak at 667 cm−1 is attributed to cellulose bonding due to out-of-plane COH bonding. Hemicellulose is found to have characteristic peaks at 896 cm−1, which correspond to CCH, COC, and CCO stretching vibration and deformation of cellulose (Senthilkumar et al. Citation2019). The lignin’s symmetric COH stretching is represented by the observable peak at 1061 cm−1 (Hyness et al. Citation2018). The CO stretching vibration of an acetyl group in lignin is accountable for the absorbance peak at 1240 cm−1 (Sumrith et al. Citation2020). The wide and intense absorption peaks seen at 1647 cm−1 represent the CO stretching vibration of lignin composition (Manimaran et al. Citation2018). The occurrence of the CO group in hemicelluloses is shown by the highest concentration at 1742 cm−1 (Sumrith et al. Citation2020). The CH bonding vibrations from CH2 group cellulose and hemicellulose correlate to the peak at 2887 cm−1 (Atiqah et al. Citation2018). The CH stretching vibrations in cellulose and hemicellulose recorded the largest proportion at 2940 cm−1; whereas OH stretching vibrations of cellulose’s hydrogen bond of hydroxyl groups produced the highest proportion at 3416 cm−1 (Kumar et al. Citation2018).
Surface morphological analysis
shows the irregular surface of the fiber, and the impurities and organic ingredients found on it.
The hemicellulose and lignin influence the uneven surface caused by the extraction process and the impurities present on the surface of the fiber (Narayanasamy et al. Citation2020). FTIR and XRD analysis show that hemicellulose and lignin are present on the fiber surface. Hence, chemical treatment is required to remove these fiber impurities and improve interfacial adhesion with the polymer matrix before the preparation of polymer matrix composites.
Thermal analysis
The TGA curve of the CG fiber and its DTG derivative showed in .
Thermal stability is an important factor to determine the use of natural fiber as reinforcement in biocomposites (Fiore, Scalici, and Valenza Citation2014). The hydrophilic nature of natural fibers has been noted by several authors as an initial mass loss (6.65%) between 30°C and 110°C due to the evaporation of moisture content from the fiber (Belouadah, Ati, and Rokbi Citation2015; Fiore, Scalici, and Valenza Citation2014). Then, the thermal stability of Cryptostegia Grandiflora fiber observed up to 230°C is evidenced by the DTG curve. Thermal degradation begins with a higher degree of chain branching and a more diverse set of polysaccharides in hemicellulose, which leads to a lower degree of polymerization, causing hemicellulose to degrade and the linear polymeric chain of cellulose to degrade (Nurazzi et al. Citation2021). The second mass loss of 62% is discovered between 230°C and 340°C, indicating the start of CGF degradation. This is due to the degradation of hemicelluloses, pectin, and cellulose’s glycoside bonds. The peak found at about 300°C suggests that cellulose I and α –cellulose may have decomposed. Similar peaks for jute, bamboo, hemp and kenaf fibers have been recorded in the literature at 298.2, 321, 308, and 307.2°C, respectively (Indran, Edwin Raj, and Sreenivasan Citation2014). At 455°C decomposition of lignin occurs (Maache et al. Citation2017). According to TGA and DTG studies, it is concluded that Cryptostegia Grandiflora fibers are suitable for low-temperature composite applications.
Tensile test
The tensile properties of raw CGF are reported in .
Table 2. Tensile properties of Raw CG fibers.
When subjected to a tensile load, the fibers of Cryptostegia Grandiflora exhibit nonlinear deformation after linear deformation. This nonlinear deformation is caused by a weakened primary cell wall and delamination between fiber cells (Belouadah, Ati, and Rokbi Citation2015). CGF has a tensile strength of 791 MPa, which is greater than sugar palm (156.96 MPa), jute (690 MPa), pineapple (627 MPa), and sisal (535 MPa), but less than flax (1035 MPa) (Nurazzi et al. Citation2021). Various combinations of cellulose with other constituents in fibers factor in variations in the mechanical properties of natural fibers (Ding et al. Citation2022).
The mechanical properties of natural fibers are determined by the cellulose percentage, microfibril angle, and degree of polymerization (Santhanam et al. Citation2016). When the fiber attained ultimate tensile strength, failure occurs immediately due to the presence of lignin in the fibers, resulting in brittle fracture (Vijay et al. Citation2022). The elongation at break of Cryptostegia Grandiflora fiber is 3.1%, comparable to flax (3.2%), ramie (3.8%), and Hemp (1.6–4%) (Nurazzi et al. Citation2021). Hence, Cryptostegia Grandiflora fiber can be used as a potential reinforcement in composites. illustrates the tensile properties of the CG fiber.
Conclusion
A new natural cellulosic fiber from the plant Cryptostegia Grandiflora is extracted and its properties are studied. Chemical analysis showed the cellulose and density content of Cryptostegia Grandiflora fiber to be 79.20% and 1.02 g/cc respectively. XRD and FTIR studies confirm the chemical functional groups and semi-crystalline structure of CGF. Also, the calculated crystallinity index from the X-ray diffraction pattern is 62%. Uneven and rough surfaces of CGF were observed in SEM images, indicating improved mechanical bonding with the matrix in the composite. The fibers can withstand up to 230°C, making them suitable for composite reinforcements. Cryptostegia Grandiflora fiber has a tensile strength of 791 MPa. The detailed characterization studies on Cryptostegia Grandiflora fiber conclude that it is desirable for the development of lightweight composites for automobile interiors and packaging applications due to its significant mechanical, thermal, physical, and chemical properties.
Highlights of this article works
A novel natural fiber extracted from Cryptostegia Grandiflora for reinforcement of polymer materials.
The characterization studies like XRD, FTIR, & TGA were performed on novel natural fibers of Cryptostegia Grandiflora.
Physical, Mechanical and Morphological studies were performed in first time on Cryptostegia Grandiflora natural fibers.
Based on results the new natural fibers from Cryptostegia Grandiflora is suitable for Reinforcement of Polymer composites materials.
Acknowledgement
This research work is a part of the Department of Science and Technology(DST)-Science for Equity, Empowerment and Development (SEED) Division-Science, Technology and Innovation (STI) Hub Project (DST File no. DST/SEED/SCSP/STI/2019/127) and the authors would like to thank DST for the sanction of the project and authors would also like to thank Kalasalingam Academy of Research and Education for the support to carry out the research work.
Disclosure statement
No potential conflict of interest was reported by the author(s).
Additional information
Funding
References
- Alias, A. H., M. N. Norizan, F. A. Sabaruddin, M. R. M. Asyraf, M. N. F. Norrrahim, A. R. Ilyas, A. M. Kuzmin, M. Rayung, S. S. Shazleen, A. Nazrin, et al. 2021. Hybridization of MMT/Lignocellulosic fiber reinforced polymer nanocomposites for structural applications: A review. Coatings 11 (11):1355. MDPI. doi:10.3390/coatings11111355.
- Asyraf, M. R. M., M. Rafidah, S. Ebadi, A. Azrina, and M. R. Razman. 2022. Mechanical properties of sugar palm lignocellulosic fiber reinforced polymer composites: A review. Cellulose 29 (12):6493–12. Springer Science and Business Media B.V. doi:10.1007/s10570-022-04695-3.
- Atiqah, A., M. Jawaid, M. R. Ishak, and S. M. Sapuan. 2018. Effect of alkali and silane treatments on mechanical and interfacial bonding strength of sugar palm fibers with thermoplastic polyurethane. Journal of Natural Fibers 15 (2):251–61. Taylor and Francis Inc. doi:10.1080/15440478.2017.1325427.
- Bahrain, S. H. K., N. R. N. Masdek, J. Mahmud, M. N. Mohammed, S. M. Sapuan, R. A. Ilyas, A. Mohamed, M. A. Shamseldin, A. Abdelrahman, and M. R. M. Asyraf. 2022. Morphological, physical, and mechanical properties of sugar-palm (Arenga pinnata (Wurmb) Merr.)-reinforced silicone rubber biocomposites. Materials 15 (12):4062. MDPI. doi:10.3390/ma15124062.
- Baskaran, P. G., M. Kathiresan, P. Senthamaraikannan, and S. S. Saravanakumar. 2018. Characterization of new natural cellulosic fiber from the bark of dichrostachys cinerea. Journal of Natural Fibers 15 (1):62–68. Taylor and Francis Inc. doi:10.1080/15440478.2017.1304314.
- Bekele, T., A. Seifu, and A. Ayenew. 2019. Impacts of invasive plant, cryptostegia Grandiflora, on species diversity and composition of invaded areas in East Shewa Zone, Ethiopia. International Journal of Agriculture Innovations and Research 7: www.biosecurity.qld.gov.au.
- Belaadi, A., A. Bezazi, M. Bourchak, F. Scarpa, and C. Zhu. 2014. Thermochemical and statistical mechanical properties of natural sisal fibers. Composites Part B: Engineering 67: 481–89. Elsevier Ltd. doi:10.1016/j.compositesb.2014.07.029.
- Belouadah, Z., A. Ati, and M. Rokbi. 2015, August. Characterization of new natural cellulosic fiber from lygeum spartum L. Carbohydrate Polymers 134:429–37. ( Elsevier Ltd). doi:10.1016/j.carbpol.2015.08.024.
- Célino, A., S. Fréour, F. Jacquemin, and P. Casari. 2014. The hygroscopic behavior of plant fibers: A review. Frontiers in Chemistry 1: JAN Frontiers Media S. A. 10.3389/fchem.2013.00043.
- Ding, L., X. Han, L. Cao, Y. Chen, Z. Ling, J. Han, S. He, and S. Jiang. 2022. Characterization of natural fiber from manau rattan (Calamus Manan) as a potential reinforcement for polymer-based composites. Journal of Bioresources and Bioproducts 7 (3):190–200. KeAi Communications Co. doi:10.1016/j.jobab.2021.11.002.
- Fiore, V., T. Scalici, and A. Valenza. 2014. Characterization of a new natural fiber from Arundo Donax L. as potential reinforcement of Polymer Composites. Carbohydrate Polymers 106 (1):77–83. doi:10.1016/j.carbpol.2014.02.016.
- Ganapathy, T., R. Sathiskumar, P. Senthamaraikannan, S. S. Saravanakumar, and A. Khan. 2019, October. Characterization of raw and alkali treated new natural cellulosic fibers extracted from the aerial roots of Banyan tree. International Journal of Biological Macromolecules 138:573–81. ( Elsevier B.V). doi: 10.1016/j.ijbiomac.2019.07.136.
- Hyness, N. R. J., N. J. Vignesh, P. Senthamaraikannan, S. S. Saravanakumar, and M. R. Sanjay. 2018. Characterization of new natural cellulosic fiber from heteropogon contortus plant. Journal of Natural Fibers 15 (1):146–53. Taylor and Francis Inc. doi:10.1080/15440478.2017.1321516.
- Indran, S., R. Edwin Raj, and V. S. Sreenivasan. 2014, September. Characterization of new natural cellulosic fiber from cissus quadrangularis root. Carbohydrate polymers 110:423–29. ( Elsevier Ltd). doi:10.1016/j.carbpol.2014.04.051.
- Jawaid, M., N. S. Alothman Othman, Y. A. Shekeil, M. T. Paridah, and H. P. S. Abdul Khalil. 2014. Effect of chemical modifications of fibers on tensile properties of epoxy hybrid composites. International Journal of Polymer Analysis and Characterization 19 (5):391–403. Taylor and Francis Inc. doi:10.1080/1023666X.2014.904081.
- Jayaramudu, J., G. Siva Mohan Reddy, K. Varaprasad, E. R. Sadiku, S. S. Ray, and A. Varada Rajulu. 2014. Mechanical properties of uniaxial natural fabric Grewia Tilifolia reinforced epoxy based composites: Effects of chemical treatment. Fibers and Polymers 15 (7):1462–68. Korean Fiber Society. doi:10.1007/s12221-014-1462-7.
- Kommula, V. P., K. Obi Reddy, M. Shukla, T. Marwala, and A. Varada Rajulu. 2013. Physico-chemical, tensile, and thermal characterization of Napier grass (Native African) fiber strands. International Journal of Polymer Analysis and Characterization 18 (4):303–14. doi:10.1080/1023666X.2013.784935.
- Kumar, R., N. Rajesh Jesudoss Hynes, P. Senthamaraikannan, S. Saravanakumar, and M. R. Sanjay. 2018. Physicochemical and thermal properties of ceiba pentandra bark fiber. Journal of Natural Fibers 15 (6):822–29. Taylor and Francis Inc. doi:10.1080/15440478.2017.1369208.
- Kumar, V., and A. S. Singha. 2010. Natural fibres-based polymers: Part I—Mechanical analysis of Pine needles reinforced biocomposites. Bulletin of Materials Science 33 (3):257–64. doi:10.1007/s12034-010-0040-x.
- Luizza, M. W., T. Wakie, P. H. Evangelista, and C. S. Jarnevich. 2016. Integrating local pastoral knowledge, participatory mapping, and species distribution modeling for risk assessment of invasive rubber vine (Cryptostegia Grandiflora) in Ethiopia’s Afar Region. Ecology and Society 21(1): Resilience Alliance. doi:10.5751/ES-07988-210122.
- Maache, M., A. Bezazi, S. Amroune, F. Scarpa, and A. Dufresne. 2017, September. Characterization of a novel natural cellulosic fiber from Juncus Effusus L. Carbohydrate Polymers 171:163–72. ( Elsevier Ltd). doi:10.1016/j.carbpol.2017.04.096.
- Madhu, P., M. R. Sanjay, P. Senthamaraikannan, S. Pradeep, S. S. Saravanakumar, and B. Yogesha. 2019. A review on synthesis and characterization of commercially available natural fibers: Part-I. Journal of Natural Fibers 16 (8):1132–44. Taylor and Francis Inc. doi:10.1080/15440478.2018.1453433.
- Manimaran, P., P. Senthamaraikannan, M. R. Sanjay, M. K. Marichelvam, and M. Jawaid. 2018, February. Study on characterization of furcraea foetida new natural fiber as composite reinforcement for lightweight applications. Carbohydrate polymers 181:650–58. ( Elsevier Ltd). doi:10.1016/j.carbpol.2017.11.099.
- Mayandi, K., N. Rajini, P. Pitchipoo, J. T. W. Jappes, and A. Varada Rajulu. 2016. Extraction and characterization of new natural lignocellulosic fiber cyperus pangorei. International Journal of Polymer Analysis and Characterization 21 (2):175–83. Taylor and Francis Inc. doi:10.1080/1023666X.2016.1132064.
- Narayanasamy, P., P. Balasundar, S. Senthil, M. R. Sanjay, S. Siengchin, A. Khan, and A. M. Asiri. 2020, May. Characterization of a novel natural cellulosic fiber from calotropis Gigantea fruit bunch for ecofriendly polymer composites. International Journal of Biological Macromolecules 150:793–801. ( Elsevier B.V). doi:10.1016/j.ijbiomac.2020.02.134.
- Nurazzi, N. M., M. R. M. Asyraf, S. Fatimah Athiyah, S. S. Shazleen, S. Ayu Rafiqah, M. M. Harussani, S. H. Kamarudin, M. R. Razman, M. Rahmah, E. S. Zainudin, et al. 2021. A review on mechanical performance of hybrid natural fiber polymer composites for structural applications. Polymers 13 (13):2170. MDPI AG. doi:10.3390/polym13132170.
- Premalatha, N., S. S. Saravanakumar, M. R. Sanjay, S. Siengchin, and A. Khan. 2021. Structural and thermal properties of chemically modified luffa cylindrica fibers. Journal of Natural Fibers 18 (7):1038–44. Bellwether Publishing, Ltd. doi:10.1080/15440478.2019.1678546.
- Raja, K., B. Prabu, P. Ganeshan, V. S. Chandra Sekar, and B. NagarajaGanesh. 2021. Characterization studies of natural cellulosic fibers extracted from shwetark stem. Journal of Natural Fibers 18 (11):1934–45. Taylor and Francis Ltd. doi:10.1080/15440478.2019.1710650.
- Ramasamy, R., K. Obi Reddy, and A. Varada Rajulu. 2018. Extraction and characterization of calotropis gigantea bast fibers as novel reinforcement for composites materials. Journal of Natural Fibers 15 (4):527–38. Taylor and Francis Inc. doi:10.1080/15440478.2017.1349019.
- Reddy, K., C. U. M. Obi, K. Ramakrishna Reddy, M. Shukla, E. Muzenda, and A. Varada Rajulu. 2015. Effect of chemical treatment and fiber loading on mechanical properties of borassus (Toddy Palm) Fiber/Epoxy Composites. International Journal of Polymer Analysis and Characterization 20 (7):612–26. Bellwether Publishing, Ltd. doi:10.1080/1023666X.2015.1054084.
- Reddy, K., B. A. Obi, K. R. N. Reddy, Y. E. Feng, J. Zhang, and A. Varada Rajulu. 2014. Extraction and characterization of novel lignocellulosic fibers from thespesia lampas plant. International Journal of Polymer Analysis and Characterization 19 (1):48–61. doi:10.1080/1023666X.2014.854520.
- Reddy, K. O., M. Shukla, C. Uma Maheswari, and A. Varada Rajulu. 2012. Evaluation of mechanical behavior of chemically modified borassus fruit short Fiber/Unsaturated polyester composites. Journal of Composite Materials 46 (23):2987–98. doi:10.1177/0021998312454032.
- Rodríguez-Estrella, R., J. Juan Pérez Navarro, B. Granados, and L. Rivera. 2010. The distribution of an invasive plant in a fragile ecosystem: The rubber vine (Cryptostegia Grandiflora) inoases of the Baja California Peninsula. Biological invasions 12 (10):3389–93. doi:10.1007/s10530-010-9758-z.
- Sanjay, M. R., G. R. Arpitha, L. Laxmana Naik, K. Gopalakrishna, and B. Yogesha. 2016. Applications of natural fibers and its composites: An overview. Natural Resources 07 (03):108–14. Scientific Research Publishing, Inc. doi:10.4236/nr.2016.73011.
- Santhanam, K., A. Kumaravel, S. S. Saravanakumar, and V. P. Arthanarieswaran. 2016. Characterization of new natural cellulosic fiber from the ipomoea staphylina plant. International Journal of Polymer Analysis and Characterization 21 (3):267–74. Taylor and Francis Inc. doi:10.1080/1023666X.2016.1147654.
- Senthilkumar, K., N. Rajini, N. Saba, M. Chandrasekar, M. Jawaid, and S. Siengchin. 2019. Effect of alkali treatment on mechanical and morphological properties of pineapple leaf fiber/polyester composites. Journal of Polymers and the Environment 27 (6):1191–201. Springer New York LLC. doi:10.1007/s10924-019-01418-x.
- Sudhakara, P., A. P. Kamala Devi, C. Venkata Prasad, K. Obi Reddy, L. Dong Woo, B. S. Kim, and J. I. Song. 2013. Thermal, mechanical, and morphological properties of maleated polypropylene compatibilized borassus fruit fiber/polypropylene composites. Journal of Applied Polymer Science 128 (2):976–82. doi:10.1002/app.38135.
- Sumrith, N., L. Techawinyutham, M. R. Sanjay, R. Dangtungee, and S. Siengchin. 2020. Characterization of alkaline and silane treated fibers of ‘water hyacinth plants’ and reinforcement of ‘water hyacinth fibers’ with bioepoxy to develop fully biobased sustainable ecofriendly composites. Journal of Polymers and the Environment 28 (10):2749–60. Springer. doi:10.1007/s10924-020-01810-y.
- Thakur, V. K., M. Kumari Thakur, and R. Kumar Gupta. 2014. Review: Raw natural fiber–based polymer composites. International Journal of Polymer Analysis and Characterization 19 (3):256–71. doi:10.1080/1023666X.2014.880016.
- Vijay, R., J. D. James Dhilip, S. Gowtham, S. Harikrishnan, B. Chandru, M. Amarnath, and A. Khan. 2022. Characterization of natural cellulose fiber from the barks of Vachellia Farnesiana. Journal of Natural Fibers 19 (4):1343–52. Taylor and Francis Ltd. doi:10.1080/15440478.2020.1764457.
- Vinod, A., M. R. Sanjay, S. Suchart, and P. Jyotishkumar. 2020. renewable and Sustainable Biobased Materials: An assessment on biofibers, biofilms, biopolymers and biocomposites. Journal of Cleaner Production 258:120978. Elsevier Ltd. doi:10.1016/j.jclepro.2020.120978.