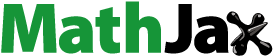
ABSTRACT
Stent grafts play an important role in sealing vessel perforations, ruptures or aneurysms for remodeling vascular access, but maintaining long-term patency remains a major challenge after implantation. Silk fibroin (SF) is an ideal vascular graft material with excellent biocompatibility and physicochemical properties. In this study, we developed a series of composite grafts consisting of regenerated SF (RSF) and silk fabric, prepared using braiding technology and layer-by-layer (LBL) self-assembly, and investigated morphology, water permeability and cytotoxicity. The results showed that silk fabrics were covered effectively and tightly by RSF films, and the anti-water permeability of grafts was closely related to fabric structural parameters and self-assembly layers, in which the braiding angle of 90° and number of axial yarns above 60 threads/10 cm obtained very low water leakage. In particular, the graft prepared by 1 × 2 silk yarn had a thinner and more uniform thickness (70–80 μm), and showed lower water permeability (8.8 mL/min·cm2). Tests on L929 fibroblast cells showed that grafts had no significant cytotoxicity, and unreacted substances could be removed by LBL rinsing. These composite grafts may be promising biomaterials for the repair and regeneration of vascular access.
摘要
支架覆膜在封堵血管穿孔、破裂或动脉瘤以重塑血管通路方面发挥着重要作用,但在植入后保持长期通畅仍是一大挑战. 丝素蛋白(SF)是一种理想的血管移植物材料,具有良好的生物相容性和物理化学性能. 在本研究中,我们开发了一系列由再生SF(RSF)和真丝织物组成的复合覆膜,使用编织技术和层层(LBL)自组装制备,并研究了形态学、水渗漏性和细胞毒性. 结果表明,RSF膜有效且紧密地覆盖了真丝织物,覆膜的抗水渗漏性与织物结构参数和自组装层数密切相关,其中编织角为90°,轴向纱线密度超过60根/10 cm时,覆膜的水渗漏性非常低. 特别是由1 × 2丝线制备的覆膜具有更薄、更均匀的厚度(70–80 μm),并且表现出更低的水渗漏性(8.8 mL/min·cm2). 对L929成纤维细胞的测试表明,覆膜没有明显的细胞毒性,未反应的物质可以通过LBL清洗去除。这些复合覆膜可能是血管通路修复和再生的有前途的生物材料.
Introduction
Cardiovascular diseases are a major cause of morbidity and mortality globally, threatening people’s lives and health. Atherosclerosis is the underlying pathology in most cases of cardiovascular dysfunction, which arises from endothelium stimuli that are strongly related to hyperglycemia and disorders of lipid metabolism, eventually resulting in occlusive artery diseases (including coronary heart disease, stroke and peripheral artery disease) (Grover and Mackman Citation2020).
Current clinical treatment methods mainly include traditional surgical bypass and interventional therapy, of which interventional therapy is the more commonly used treatment, and its clinical progress is due to the development of stents. Bare metal stents (BMS), the earliest generation of vascular stents, possess excellent mechanical properties to effectively restore and maintain the patency of occlusive vessels (Tammareddi, Sun, and Li Citation2016). However, the incidence of in-stent restenosis is up to 30% within 6–8 months post-procedure (Cassese et al. Citation2014). Thus, drug-eluting stents (DES) have been developed to address these issues, which deliver bioactive drugs to inhibit neointimal hyperplasia, reducing the occurrence of restenosis compared with BMS. Roopmani et al. (Citation2019) reported a dual drug (atorvastatin and fenofibrate)-loaded poly(L-lactide-co-caprolactone) coating DES, which had a cytostatic effect on human vascular smooth muscle cells, but promoted the growth and migration of human umbilical vein endothelial cells. Qiu et al. (Citation2019) developed an endothelium-mimetic coating via surface modification with heparin and selenocysteine, improving the antithrombosis, re-endothelialization, and anti-restenosis capacities in vivo. However, vulnerable plaques and lesions are still exposed to blood flow after DES implantation, readily causing stent thrombosis, in-stent restenosis, and bleeding complications over time (Ben-Yehuda Citation2020).
Third-generation stent-grafts, which consist of metal stents and flexible grafts, have shown satisfactory clinical efficacy for treating aneurysms, sealing vessel perforations, and ruptures to reduce the risk of internal hemorrhage. Lequoy et al. (Citation2016) used a bioactive coating with chondroitin sulfate and epidermal growth factor to modify expanded PTFE stent-grafts, and the modified stent-grafts could accelerate healing of canine aneurysms after implanting. Giol et al. (Citation2018) designed a PET graft covalently modified with gelatin, which promoted reendothelialization and anti-coagulation properties. However, these two materials are prone to endoleak or malapposition due to their poor compliance and non-degradation, leading to repeated episodes of thrombosis and inflammation (Gupta et al. Citation2020; Verzini et al. Citation2021). A recent clinical analysis report indicated that expanded PTFE stent-grafts and PET stent-grafts suffered worse long-term results in thoracic endovascular aneurysm repair, compared with surgical bypass (Mezzetto et al. Citation2021). By comparison, polyurethane grafts have excellent compliance, and they are conducive to apposing adequately and firmly with the vessel walls and supporting endothelialization (Lattuca et al. Citation2015). However, the long-term patency of such materials remains unsatisfactory (Adipurnama et al. Citation2017).
Natural biomacromolecules such as fibrin and collagen are ideal vascular graft materials that can prevent further thrombus formation and reduce the occurrence of restenosis (Janke et al. Citation2017; Safiullin et al. Citation2015). Similarly, silk fibroin (SF) derived from insects is another natural biomacromolecule with incredible potential for application in tissue engineering, based on 30 years of research (Gupta and Mandal Citation2021; Zahedi et al. Citation2021). Studies have confirmed that SF has excellent blood compatibility and cytocompatibility. Our previous work developed an SF small-caliber artificial blood vessel that achieved rapid endothelialization and maintained long-term patency without intimal hyperplasia (Li et al. Citation2019, Citation2020).
Unlike collagen and fibrin, silk is a natural fiber with excellent mechanical properties and rich sources, hence it has been applied industrially to a variety of textiles. Moreover, SF accounts for up to 75% of silk, is easily extracted in high purity, and can be simply prepared into aqueous solution and regenerated SF (RSF) materials with various morphologies. Herein, we developed a novel vascular stent graft, combining two distinct material forms of SF. Silk fabrics were designed as a reinforcing material and embedded with RSF films, which were prepared using layer-by-layer (LBL) self-assembly via a rotary drying device. The morphology and water permeability of grafts were investigated, and the cytocompatibility of grafts was evaluated by assessing the growth of L929 fibroblasts.
Materials and methods
Preparation of SF aqueous solution and silk tubular fabric
Bombyx mori SF aqueous solution was prepared as described previously (Shi et al. Citation2019). In brief, raw silk was degummed by Na2CO3, then dissolved in 9.3 M LiBr solution at 65°C until dissolved completely. The SF aqueous solution was dialyzed against deionized water, then concentrated to 80 mg/mL.
Silk tubular fabric was braided with a 10 mm inner diameter by two types of degummed 1 × 2 or 3 × 2 silk yarns (). The 1 × 2 silk yarn was a thrown silk composed of two strands of 20/22 denier (D) raw silk, and the 3 × 2 silk yarn consisted of two-ply yarns composed of two strands of thrown silk, each with three strands of 20/22 D raw silk. The structural parameters of the silk tubular fabrics were designed by adjusting braiding angles, number of axial yarns, and silk yarn sizes (). Samples were named as shown in .
Figure 1. Schematic diagram of SF tubular grafts preparation and performance test. (a) Preparation of silk tubular fabric, (b) Parameter of the silk tubular fabric, (c) Preparation of SF tubular grafts by LBL self-assembly, (d) Water permeability test.
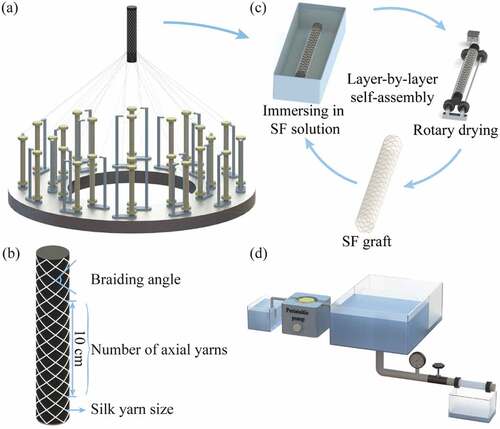
Table 1. The design of silk tubular fabrics.
Preparation of SF tubular grafts by LBL self-assembly
RSF aqueous solution and polyethylene glycol diglycidyl ether (PEG-DE; MW500 Da; Sigma, St. Louis, MO, USA) were mixed together at a weight ratio of 1.0:0.8 and stirred for 30 min to cross-link adequately. Rhodamine B-labeled SF was prepared by adding rhodamine B to the cross-linked SF solution at 1 mg/mL.
The silk tubular fabric was completely immersed in cross-linked SF solution, then dried on a rotary drying device (50 rpm, 60°C) (). Finally, an SF tubular graft was obtained after x (1–7) immersion-drying cycles, and named as SFx. A Rhodamine B-labeled SF tubular graft was prepared by alternately immersing in the rhodamine B-labeled SF aqueous solution and cross-linked SF solution in a dark room.
Morphology assay
The surface morphology of the SF tubular grafts was observed using a CLS100 stereoscopic microscopy (Leica, Wetzlar, Germany). The surface micrography was assessed by scanning electron microscope (SEM) using an S-4800 instrument (Hitachi, Tokyo, Japan). The rhodamine B-labeled tubular SF graft was visualized at 555 nm using an FV1000 confocal laser scanning microscope (Olympus, Tokyo, Japan).
Water permeability test
Water permeability tests were performed in accordance with ISO 7198: 2016. In brief, one end of the SF tubular graft was connected to a custom-made water permeability device, which maintained a 120 mmHg water pressure, and the other end was blocked (). Each sample was tested for 1 min at an invariable pressure and the leakage volume of water was measured. Water permeability of samples was calculated using the following equation:
where Q is the volume of water leaking through the sample (mL), d is the inner diameter of the sample (cm), l is the length of the sample (cm), and t is the testing time (min). Five replicate samples were analyzed for each SF graft.
Cell culture
L929 fibroblasts (Cell Bank of the Chinese Academy of Sciences, Shanghai, China) were cultured in Dulbecco’s modified Eagles medium (DMEM; Gibco, Carlsbad, CA, USA) containing 10% (v/v) fetal bovine serum (FBS; Gibco) and 1% (v/v) penicillin-streptomycin (Thermo Fisher, Waltham, MA, USA) at 37°C in a 5% CO2 incubator. During the logarithmic growth phase, cells were trypsinized using 0.25% trypsin (Sigma) and re-suspended at 5.0 × 104 cells/mL in fresh DMEM with FBS and antibiotics.
CCK-8 assay
Grafts were immersed in deionized water using LBL immersing methods or final immersing methods to remove unreacted substances. The LBL immersing group was immersed for 10 min after the end of each immersion-drying cycle. The final immersing group was immersed for 3 h after the end of all immersion-drying cycles. Both group grafts were cut into small pieces, sterilized, then immersed in DMEM medium (9 cm2/mL) for 24 h to obtain extracts. A 200 μL of the L929 cell suspension was added to a 96-well plate and pre-incubated for 24 h. After removing the medium, equal volumes (100 μL each) of extract and complete DMEM were added to each well and incubated. A well with 200 μL of complete DMEM served as a negative control. Complete DMEM containing 5% dimethyl sulfoxide (DMSO; Sinopharm, Shanghai, China) and 10% PEG-ED served as positive controls. Cell morphology was observed using an IX51 inverted microscope (Olympus). After culturing for 3 days, the medium in each well was replaced with 180 μL of fresh medium and 20 μL of CCK-8 solution (Bimake, Houston, TX, USA), and incubated for 3 h. The absorbance (A) of the mixed solution was measured using a Synergy HT microplate reader (BIO-TEK, Winooski, VT, USA) at 450 nm. The relative growth rate (RGR) of L929 cells was calculated using the following equation:
where a and b refer to samples and negative control, respectively. Six replicate samples were analyzed for each material.
Statistical analysis
All the quantitative results are presented in mean±standard deviation (SD). Statistical significance was determined by one-way analysis of variance using SPSS software (Version 26, IBM, Chicago, IL, USA). Significance was set at *p < .05 and **p < .01.
Results and discussion
Assumption of graft preparation and the mechanism of film formation
Fabrics, as reinforced materials, have been extensively studied and employed in clinical settings for a long time (Kale, Gorade, and Parmaj Citation2020; Li et al. Citation2019). In this work, we developed a novel SF tubular graft via LBL self-assembly for fabricating vascular stents (). The RSF films embedded with silk braiding fabrics function as a mechanical reinforcement, and the dense films should meet the requirements of ultrathin and even thickness to isolate blood flow and diseased sites. In our experiments, we found that a large number of defects (such as white clots or bubbles) appeared between the layers following static drying after LBL immersion (). Therefore, we developed a rotary drying method () in which the SF solution can flow regularly and uniformly, eliminating the defects and smoothing the surface of grafts ().
Figure 2. Drying methods of SF solution in LBL self-assembly process. (a) Grafts by static drying, (b) Grafts by rotary drying. The scale bar is 5 mm.
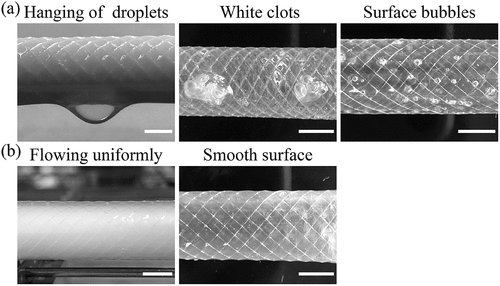
As shown in , grafts were slightly thicker at the interlacing points of braid fabrics than the RSF films without silk threads, while a tight bond between the silk yarns and RSF films was formed without cracks. In the process of LBL self-assembly, SF macromolecules were closely bound to the surface of silk yarns and infiltrated into the inside of interlacing points through polar interactions between functional groups (-OH, -COOH, -NH2, -CONH-), wrapping the silk yarns and interlacing points firmly, or binding tightly to the surface of cured films.
Figure 3. The cross-sectional morphology of grafts (1 × 2/90°/60/SF6). (a) SEM observation, (b) Rhodamine B fluorescence staining observation. 1–6 represent 1–6 layers with SF self-assembly.
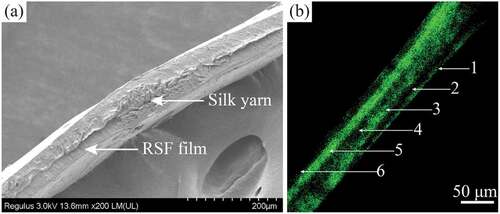
shows a fluorescence image of a graft cross-section in the area without interlacing points. Green fluorescence was observed at the first, third and fifth layers, interspersed with black strips between the fluorescent strips (second and fourth layers). The average thickness of each SF layer was ~11 μm, but the thickness between layers was uneven. When self-assembling the first layer, the SF layer was slightly thin but formed a continuous film, and the thickness of SF deposited in the third layer increased, which was uniform in the area without the interlacing points of silk yarns.
Adjusting graft morphology by varying fabric structural parameters
The surface morphologies of grafts prepared by varying fabric structures are shown in . Compared with grafts of the same number of axial yarns (100 threads/10 cm as an example), the radial threads of 3 × 2/25°/100/SF6 were sparse, while those of 3 × 2/90°/100/SF6 were denser. The radial pitch length of 3 × 2/25°/100/SF6 was about three times that of 3 × 2/90°/100/SF6. The distribution of threads was uniform in the radial and axial directions at a 90° braiding angle, implying that the mechanical reinforcement in different directions may be more homogeneous. Compared with grafts at the same braiding angle (90° as an example), the fabric network became denser with an increasing number of axial yarns, which is also beneficial to the mechanical reinforcement of grafts.
Graft morphology is affected by different self-assembly layers
The thickness of grafts determines their ability to resist blood flow shear and blood pressure. With an increase in SF self-assembly layers, silk yarns and interlacing points were gradually wrapped by RSF films, the braid fabric structures became blurred or disappeared, and the surfaces of grafts became flat (). When self-assembling the first layer, a small amount of SF was deposited on the tube surface, and the turns of silk yarns were clearly visible and completely bulged, especially in the 3 × 2 group. When self-assembling three layers, silk yarns in the 1 × 2 group were closely embedded in the RSF films, the visibility of fabric structures was significantly reduced, and the surface of grafts became flat. However, the silk yarns in the 3 × 2 group were still visible, and the surface was uneven. When self-assembling six layers, the surfaces of the 1 × 2 group grafts were flat, and the braiding fabrics were completely covered by the RSF films with a thickness of about 70–80 μm. Although the surfaces of the 3 × 2 group grafts were still uneven, the interlacing points of the silk yarns were not exposed, and its thickness containing the interlacing points was about 160–170 μm.
Water permeability
Water permeability related to different fabric structural parameters
The main role of stent grafts is to seal vessel perforations, ruptures or aneurysms for remodeling vascular access. Therefore, the graft should be resistant to blood leakage. Compared with grafts possessing the same number of axial yarns, water permeability tended to decrease with increasing braiding angle (). The water permeability of 3 × 2/25°/100/SF6 grafts was 17.7 times that of 3 × 2/90°/100/SF6 grafts, and that of 1 × 2/25°/100/SF6 grafts was 5.7 times that of 1 × 2/90°/100/SF6 grafts. A higher braiding angle resulted in the deposition of more radial yarns, which provided more bonding sites between silk yarns and RSF films, resulting in a decrease in water permeability.
Figure 6. The water permeability of grafts with varying fabric structural parameters (6 layers via self-assembly; a, b) and different SF self-assembly layers (c). (a) Grafts of 3 × 2 group, (b) Grafts of 1 × 2 group.
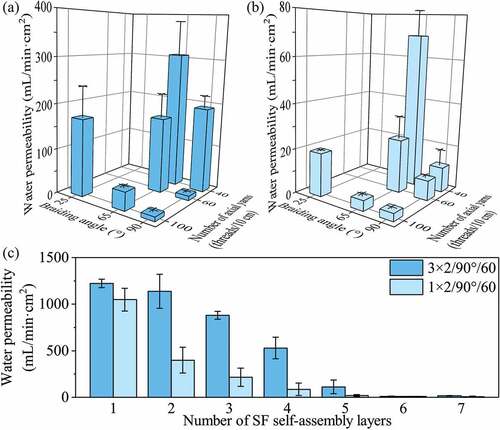
Regarding the number of axial yarns, the water permeability of grafts decreased significantly with an increase in the number of axial yarns (). When the number of axial yarns was changed from 40 threads/10 cm to 60 threads/10 cm, the water permeability of 3 × 2/90°/SF6 decreased significantly from 191.4 mL/(min·cm2) to 10.0 mL/(min·cm2). A higher number of axial yarns also provided more sites for bonding between silk yarns and RSF films, resulting in lower water permeability. However, when the number of axial yarns was increased further from 60 threads/10 cm to 100 threads/10 cm, water permeability decreased insignificantly.
The silk yarn size also influenced the water permeability of grafts significantly. For the same braiding angle and number of axial yarns, the water permeability of grafts prepared from 3 × 2 silk yarn was higher than that prepared from 1 × 2 silk yarn. There was a significant height difference between interleaving points and RSF films in the 3 × 2 group, and it formed a type of pennation angle structure in the interface, leading to the higher water permeability.
Water permeability related to different self-assembly layers
On the basis of the above investigation, we further analyzed the water permeability of 3 × 2/90°/60 and 1 × 2/90°/60 grafts with different self-assembly layers (). With increasing SF self-assembly layers, the water permeability of the two grafts decreased significantly, owing to an increase in film thickness and tighter binding between RSF films and silk yarns at higher self-assembly layers. When self-assembling three layers, the water permeability of the 1 × 2 group decreased significantly from 1048.6 mL/(min·cm2) to 215.0 mL/(min·cm2) compared with grafts self-assembling one layer. By contrast, when self-assembling four layers, the water permeability of the 3 × 2 group (529.1 mL/min·cm2) remained significantly higher than that of the 1 × 2 group self-assembling two layers (399.1 mL/min·cm2). When self-assembling 6–7 layers, the water permeability of both groups was similar (less than 10.0 mL/min·cm2) because the SF macromolecules self-assembled into a thicker film, and firmly wrapped the silk yarns and interlacing points. The results show that the developed grafts displayed lower water permeability than commercially available woven PET grafts (78.0 mL/min·cm2) and met the implantation requirements in vivo (Takeuchi et al. Citation2014).
Cytotoxicity
The RSF films in our grafts were prepared via LBL self-assembly, which formed a strong interlayer bond, leading to a dense film structure. However, this could retain residues of unreacted PEG-DE and SF molecules in grafts. We tested two different methods, LBL immersing and final immersing, to remove unreacted substances, then evaluated the cytocompatibility of these grafts. As shown in , after 1 day of culture, cells had spread well in fusiform and triangular shapes, but not in the 10% PEG-DE positive control. After 3 days of culture, there was no significant difference in the cell proliferative activity of samples compared with the negative control. Cells in the two positive controls spread very poorly and showed no proliferative activity. The highest cell density was observed in the LBL immersing group. Quantitative analysis results of cell proliferation for 3 days are shown in . The RGR of the LBL immersing group was 111%, compared with 91% for the final immersing group, and <50% for the 10% PEG-DE and 5% DMSO positive control groups. The results showed that grafts subjected by the two immersing methods had no significant cytotoxicity.
Figure 7. Growth of L929 cells in medium containing extract of grafts (a – e) and cell proliferation activity for 3 days (f). (a) LBL immersing, (b) Final immersing, (c) 10% PEG-DE positive control, (d) 5% DMSO positive control, (e) Negative control. The scale bar is 200 μm. “*” represents p <.05, “**” represents p <.01.
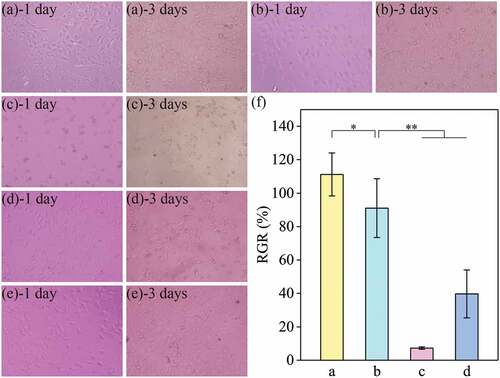
Conclusions
In the work, we developed a novel vascular graft via LBL self-assembly, which was fabricated by compounding RSF films with silk braiding fabric. The morphology results showed that silk yarns and interlacing points were effectively and tightly covered RSF films with increasing SF self-assembly layers, and the surface of grafts was smooth and flat. The water permeability of grafts could be controlled by adjusting the braiding angle, the number of axial yarns, and the number of SF self-assembly layers. When the braiding angle was 90° and the number of axial yarns was up to 60 threads/10 cm, the water permeability of grafts was satisfactory, and that of 1 × 2/90°/60 and 3 × 2/90°/60 was less than 10 mL/(min·cm2), which is promising for construction of vascular stents. In addition, the grafts exhibited no significant cytotoxicity. The preliminary results suggest that these novel grafts developed for vascular stents have promising application prospects.
Highlights
We design and develop a series ofstent grafts for application in vascular tissue engineering.
The composite grafts were prepared using braiding technology and layer-by-layer self-assembly.
Silk fabrics were covered effectively and tightly by flexible regenerated silk fibroin films.
Grafts had a thinner and uniform thickness (70–80 μm) with no cytotoxicity.
Grafts exhibited lowerwater permeability (less than 10 mL/min·cm2), meeting the requirements for implantation in vivo.
Acknowledgements
We thank International Science Editing (http://www. International- scienceediting.com) for editing this manuscript.
Disclosure statement
The authors declare that they have no known competing financial interests or personal relationships that could have appeared to influence the work reported in this paper.
Data availability statement
The data that support the findings of this study are available from the corresponding author[JNW] upon reasonable request.
Additional information
Funding
References
- Adipurnama, I., M. C. Yang, T. Ciach, and B. Butruk-Raszeja. 2017. Surface modification and endothelialization of polyurethane for vascular tissue engineering applications: A review. Biomaterials Science 5 (1):22–13. doi:10.1039/c6bm00618c.
- Ben-Yehuda, O. 2020. Long-term outcomes with drug-eluting stents: Beyond stent choice. Journal of the American College of Cardiology 76 (2):159–61. doi:10.1016/j.jacc.2020.05.050.
- Cassese, S., R. A. Byrne, T. Tada, S. Pinieck, M. Joner, T. Ibrahim, L. A. King, M. Fusaro, K. L. Laugwitz, and A. Kastrati. 2014. Incidence and predictors of restenosis after coronary stenting in 10 004 patients with surveillance angiography. Heart 100 (2):153–59. doi:10.1136/heartjnl-2013-304933.
- Giol, E. D., S. Van Vlierberghe, R. E. Unger, D. Schaubroeck, H. Ottevaere, H. Thienpont, C. J. Kirkpatrick, and P. Dubruel. 2018. Endothelialization and anticoagulation potential of surface-modified PET intended for vascular applications. Macromolecular Bioscienc 18 (7):1800125. doi:10.1002/mabi.201800125.
- Grover, S. P., and N. Mackman. 2020. Tissue factor in atherosclerosis and atherothrombosis. Atherosclerosis 307:80–86. doi:10.1016/j.atherosclerosis.2020.06.003.
- Gupta, N., K. L. Hynes, O. Mahrouyan, C. S. Briggs, and A. Azzizadeh. 2020. polymer leak with the ovation abdominal stent graft system: Early recognition and treatment. Vascular 28 (2):159–64. doi:10.1177/1708538119862923.
- Gupta, P., and B. B. Mandal. 2021. Silk biomaterials for vascular tissue engineering applications. Acta biomaterialia 134:79–106. doi:10.1016/j.actbio.2021.08.004.
- Janke, H. P., J. Bohlin, R. Lomme, S. M. Mihaila, J. Hilborn, W. F. J. Feitz, and E. Oosterwijk. 2017. Bioinspired coupled helical coils for soft tissue engineering of tubular structures-improved mechanical behavior of tubular collagen type I templates. Acta biomaterialia 59:234–42. doi:10.1016/j.actbio.2017.06.038.
- Kale, R. D., V. G. Gorade, and O. Parmaj. 2020. Development and characterization study of silk filament reinforced chitosan biocomposite. Journal of Natural Fibers 17 (1):66–74. doi:10.1080/15440478.2018.1465878.
- Lattuca, B., L. Schmutz, L. Cornillet, B. Ledermann, V. Fernandez, P. Messner, F. Leclercq, and G. Cayla. 2015. New polyurethane covered stent with low profile for treatment of a large aneurysm after left anterior descending artery stenting: First experience. International Journal of Cardiology 201:208–09. doi:10.1016/j.ijcard.2015.08.036.
- Lequoy, P., H. Savoji, B. Saoudi, A. Bertrand-Grenier, M. R. Wertheimer, G. De Crescenzo, G. Soulez, and S. Lerouge. 2016. In vitro and pilot in vivo evaluation of a bioactive coating for stent grafts based on chondroitin sulfate and epidermal growth factor. Journal of Vascular and Interventional Radiology 27 (5):753–60. doi:10.1016/j.jvir.2016.02.004.
- Li, H. L., G. Z. Song, W. Tian, M. Y. Ding, X. L. Sun, J. M. Xu, F. L. Dong, A. Q. Wang, P. Ning, Y. Yin et al, 2020. Motility and function of smooth muscle cells in a silk small-caliber tubular scaffold after replacement of rabbit common carotid artery. Materials Science & Engineering C-Materials for Biological Applications 114:110977. doi:10.1016/j.msec.2020.110977.
- Li, H. L., Y. N. Wang, X. L. Sun, W. Tian, J. J. Xu, and J. N. Wang. 2019. Steady-state behavior and endothelialization of a silk-based small-caliber scaffold in vivo transplantation. Polymers 11 (8):1303. doi:10.3390/polym11081303.
- Mezzetto, L., L. Scorsone, R. Silingardi, S. Gennai, N. Leone, G. Piffaretti, and G. F. Veraldi. 2021. early and long-term results of ePTFE (gore tag(r)) versus dacron (relay plus(r) Bolton) grafts in thoracic endovascular aneurysm repair. Annals of Vascular Surgery 71:419–27. doi:10.1016/j.avsg.2020.07.054.
- Qiu, H., P. K. Qi, J. X. Liu, Y. Yang, X. Tan, Y. Xiao, M. F. Maitz, N. Huang, and Z. L. Yang. 2019. Biomimetic engineering endothelium-like coating on cardiovascular stent through heparin and nitric oxide-generating compound synergistic modification strategy. Biomaterials 207:10–22. doi:10.1016/j.biomaterials.2019.03.033.
- Roopmani, P., S. Satheesh, D. C. Raj, and U. M. Krishnan. 2019. Development of dual drug eluting cardiovascular stent with ultrathin flexible poly(l-lactide-co-caprolactone) coating. ACS Biomaterials Science & Engineering 5 (6):2899–915. doi:10.1021/acsbiomaterials.9b00303.
- Safiullin, R., W. Christenson, H. Owaynat, I. S. Yermolenko, M. K. Kadirov, R. Ros, and T. P. Ugarova. 2015. Fibrinogen matrix deposited on the surface of biomaterials acts as a natural anti-adhesive coating. Biomaterials 67:151–59. doi:10.1016/j.biomaterials.2015.07.007.
- Shi, P. G., L. Zhang, W. Tian, H. L. Li, Q. Y. Wang, H. G. Yi, Y. Yin, A. Q. Wang, P. Ning, F. L. Dong, and J. N. Wang. 2019. Preparation and anticoagulant activity of functionalised silk fibroin. Chemical Engineering Science 199:240–48. doi:10.1016/j.ces.2019.01.022.
- Takeuchi, M., T. Kuratani, S. Miyagawa, Y. Shirakawa, K. Shimamura, K. Kin, T. Yoshida, Y. Arai, T. Hoashi, and N. Teramoto. 2014. Tissue-engineered stent-graft integrates with aortic wall by recruiting host tissue into graft scaffold. The Journal of Thoracic and Cardiovascular Surgery 148 (4):1719–25. doi:10.1016/j.jtcvs.2014.04.003.
- Tammareddi, S., G. Y. Sun, and Q. Li. 2016. Multiobjective robust optimization of coronary stents. Materials & Design 90:682–92. doi:10.1016/j.matdes.2015.10.153.
- Verzini, F., E. Cieri, A. Kahlberg, Y. Sternbach, R. Heijmen, K. Ouriel, S. Robaina, and A. Azizzadeh. 2021. A preliminary analysis of late structural failures of the Navion stent graft in the treatment of descending thoracic aortic aneurysms. Journal of Vascular Surgery 74 (4):1125–34. doi:10.1016/j.jvs.2021.04.018.
- Zahedi, P., N. H. Besheli, M. Farokhi, F. Mottaghitalab, A. Sohrabi, and S. A. Ghorbanian. 2021. Silk fibroin nanoparticles functionalized with fibronectin for release of vascular endothelial growth factor to enhance angiogenesis. Journal of Natural Fibers. Advance Online Publication. doi:10.1080/15440478.2021.1982814.