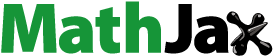
ABSTRACT
Sugar palm (Arenga Pinnata) fiber is abundant in Malaysia which necessitates its utilization as a source of agricultural waste for industrial development. This paper aims at characterizing the sugar palm fiber to explore its silica content as a potential renewable material. The sugar palm fiber was carbonized, and the ash obtained was characterized via X-ray diffraction (XRD), Fourier transform infrared spectroscopy (FTIR), thermogravimetric analysis (TGA), energy dispersive spectroscopy (EDS) and field emission scanning electron microscope (FESESM). The presence of amorphous silica (SiO2) with an average particle size of 41.25 nm was revealed by X-ray diffraction. Fourier transform infrared spectroscopy indicated the presence of functional groups such as silane and siloxane groups, and thermogravimetric analysis and differential scanning calorimetry identified the main degradation stages of the sugar palm fiber ash, and the decomposition of hemicellulose, lignin, cellulose, and carbonate to other oxides. The energy dispersive spectroscopy of the sugar palm fiber ash confirmed silica to be of the highest amount than other elements. Sugar palm fiber ash has been found as a possible silica substitute and a prospective industrial resource.
摘要
马来西亚盛产糖棕榈(Arenga Pinnata)纤维,因此必须将其作为农业废弃物来源用于工业发展. 本文旨在对糖棕榈纤维进行表征,以探索其二氧化硅含量作为一种潜在的可再生材料. 对糖棕榈纤维进行碳化,并通过X射线衍射(XRD)、傅里叶变换红外光谱(FTIR)、热重分析(TGA)、能量色散光谱(EDS)和场发射扫描电子显微镜(FEESM)对获得的灰分进行了表征. 通过X射线衍射揭示了平均粒径为41.25 nm的无定形二氧化硅(SiO2)的存在. 傅里叶变换红外光谱表明存在硅烷基和硅氧烷基等官能团,热重分析和差示扫描量热法确定了糖棕榈纤维灰分的主要降解阶段,以及半纤维素、木质素、纤维素和碳酸盐分解为其他氧化物. 糖棕榈纤维灰分的能量色散光谱证实二氧化硅的含量高于其他元素. 糖棕榈纤维灰已被发现是一种可能的二氧化硅替代品和一种潜在的工业资源.
Introduction
Sugar palm tree also called Arenga Pinnata (Wurmb.) Merr is a multipurpose tree that is native to tropical countries like Malaysia, India, Indonesia, and the Philippines. The sugar palm tree () is a natural fiber source having the braided fiber found on the sugar palm tree trunk (). The stem and fibers of the sugar palm tree contain a lot of carbohydrates and green fiber respectively (Ilyas, Sapuan, and Ishak Citation2018, Ilyas et al. Citation2018; Sanyang et al. Citation2016). Sugar palm fiber (SPF) is a promising source of natural fiber-reinforcing materials in Malaysia because it is inexpensive and readily available, and it does not necessitate any additional processes during preparation such as water retting or mechanical decortication. This is due to the fact that threads, which were originally weaved from the bottom to the top of the sugar palm trunk, are natural woven fibers (Ishak et al. Citation2013a; Sherwani et al. Citation2021).
Figure 1. (a) Sugar palm tree and (Ishak et al. Citation2013a) (b) Sugar palm fiber.
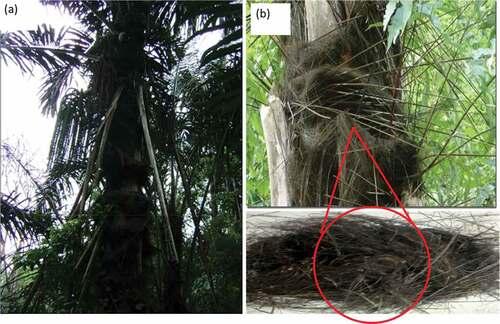
Natural fibers are regarded as the most readily available, environment-friendly, useful bio-material, and primary asset for renewable energy in today’s world. Natural fibers have sufficient qualities to replace fossil fuels, and their performance is determined by their chemical composition (Armynah et al. Citation2018; Bisht, Chandra Gope, and Rani Citation2020; Ishak et al. Citation2013a; Sanjay et al. Citation2016). Fiber is frequently employed for several reasons due to its renewable nature, such as direct heat generation and as a raw material for thermochemical and biological conversion in the production of fuels and chemicals. Fibrous materials are becoming a more popular source of silicon-based refractory compounds (Adeleke et al. Citation2020; Bardalai and Mahanta Citation2016; Ikubanni et al. Citation2020).
Fiber ash is obtained by the controlled burning of natural fiber to produce silica with high market value and several advantages, which include high volatile matter content, and low sulfur, ash, and nitrogen contents. Natural fiber ashes contain metals in oxide form such as SiO2, Fe2O3, Al2O3, CaO, MnO, and others. This ash is used in a variety of applications, including the manufacture of cement-based materials, composite materials, the electronic industry, fuel cell technology, nanomaterials, and bioenergy production. Natural fiber ash is the most recently discovered reinforcement material used in Metal Matrix Composites (MMCs) due to its low density, low cost of production, and availability. A few researchers studied groundnut shell ash and discovered that SiO2 is a major constituent in groundnut shell ash 18.61, 18.79, and 34.20%, which are used to fabricate Aluminum Matrix Composites (AMCs) (Alaneme, Bodunrin, and Awe Citation2018; Jadhav et al. Citation2019; Venkatesh, Arjunan, and Ravikumar Citation2019). The percentage of SiO2 in the fiber ash powder varies depending on the cultivation region, basic shell properties, soil chemistry, and ash powder production route (Terzioglu et al. Citation2013). The production of silica is dependent on its purity (acid treatment, demineralized water washing, and ion exchange treatment) and transformations experienced based on combustion conditions (time and temperature), supercritical drying process, sol-gel, alkaline fusion, organic alkoxides, vapor-phase reaction, and thermal breakdown. Another consideration is the source of the silica, which can be rice husk, wheat, Dracaena reflexa, corn cob, palm kernel shell, coffee husk, and so on (Ananthi, Geetha, and Ramesh Citation2016; Athinarayanan et al. Citation2015; Attol and Hamied Mihsen Citation2019; Channoy et al. Citation2018; Costa and Paranhos Citation2018; Imoisili, Ukoba, and Jen Citation2020; Manimaran et al. Citation2019; Salakhum et al. Citation2018; Usama, Subhani, and Wilayat Husain Citation2016).
Silica particles (both chemical and physical) have wide and unique applications in areas such as agricultural biotechnology, environmental adsorption, medical applications, insulation-based material, filler for building materials, catalysis, and the production of solar cells (Channoy et al. Citation2018; Gewaily Citation2019; Hernández-Martínez et al. Citation2020; Huseini and Fitriyano Citation2019; Narayan et al. Citation2018).
Silica gels are a three-dimensional connection of colloidal silica that is both hard and flexible. Amorphous mesoporous silica gels are classified as aqua-gel (pores packed with water), aero-gel (solvent extracted by supercritical extraction), and xerogel (aqueous phase in the pores is eliminated by evaporation) based on their synthesis procedure (Kumar et al. Citation2013).
In any fiber ash sample, Fourier transform infrared spectroscopy analysis has proven to be a highly effective method for identifying the chemical components and functional groups present in them (Liu, He, and Uchimiya Citation2015). While the thermogravimetric analysis gives a proximate analysis for example, coconut shell having a volatile matter, fixed carbon, ash, and moisture content of 57, 19, 13, and 10.03%, respectively (Ghafar, Halidi, and So’aib Citation2020). Lwin et al. (Citation2019) studied activated carbon made from rice husk biomass that had been carbonized for 1 h at 300°C. The carbonized material was then immersed in 1 M KOH in a 1:1 ratio for 24 h before being physically activated at 300°C for a duration of 2 h in a muffle furnace. The structure of activated carbon rice husk biochar (AC-RHB) was studied using the X-ray diffraction method. The presence of a variety of functional groups in AC-RHB was revealed using Fourier transformation infrared spectroscopy, while energy dispersive spectroscopy was used to confirm the existence of C, O, Si, and other elements.
Silica materials have recently caught the interest of researchers due to their important role in modern applications such as drug delivery, vulcanized rubber, etc (Tishkevich et al. Citation2019; Vorobjova et al. Citation2020; Zdorovets and Kozlovskiy Citation2020). However, there is a problem with silica production due to the use of complex processes such as alkaline and acid treatment, which incur additional costs and prolong reaction time (24–48 h) (Uda et al. Citation2021). The concept of synthesizing silica from natural fiber through a simple route at low temperatures increases the yield, profitability, and cost-effectiveness, whereas alkaline treatment causes fiber degradation (Hernández-Martínez et al. Citation2020; Mukhtar et al. Citation2018). There are numerous works reported on silica production from agricultural waste, and their content was high. However, according to the literature, and to the best of our knowledge, this is the first report on silica synthesis and detailed characterization from sugar palm fiber via carbonization. Considering the aforementioned matter, the objective of this study is to synthesize sugar palm fiber ash using a simple extraction technique to determine the amount of silica present, its detailed characterization, and its possible applications in various industries. The sugar palm fiber ash (SPFA) was thermally and morphologically analyzed using TGA, DSC, and FESEM, respectively, while the chemical constituents were revealed using EDS and FTIR.
Material and methods
Material
Sugar palm fibers (SPFs) were obtained from the sugar palm tree farm in Jempol, Negeri Sembilan, Malaysia.
Carbonization and calcination of SPF
The black SPFs () were washed with running tap water to remove dust before being air-dried in the laboratory for 48 h at room temperature. The dried SPFs were carbonized by burning them in the open air for complete combustion (). Then it was calcined at 700°C for 4 h in a muffle furnace () to remove the carbonaceous and volatile matter to obtain grayish color sugar palm fiber ash (SPFA), as depicted in . presents the stages of the carbonization and calcination processes for obtaining SPFA.
Morphological and chemical characterization
X-Ray diffraction (XRD) analysis
The diffraction patterns, particle size, and crystalline structures of the SPFA particles were determined using X-Ray Diffraction (XRD). The evaluation was performed using a Shimadzu XRD 6100 (30 kV, 30 mA) with a Cu-Ka1 wavelength of 1.5405 Å and a rate of 2 °/min in the angle range of 2θ = (4 ° − 90 °). CuKα radiation was used for the XRD characterization, which was done at room temperature. Using the Scherrer EquationEquation (1)(1)
(1) , particle size is determined from the XRD diffraction pattern and the obtained Full Width at Half Maximum (FWHM).
Where β represents the line broadening at half maximum intensity, K is the Scherrer constant (K = 0.9), D is crystal diameter, and X-ray wavelength (λ) is equivalent to 0.15406 nm.
Fourier transformation infrared (FTIR) spectroscopy analysis
Fourier transformation infrared (FTIR) spectroscopy analysis was used to determine the functional groups contained in SPFA. For this analysis, the SPFS sample was combined with KBr and formed into a pellet with the use of a hydraulic press. The FITR of the SPFS sample was analyzed in the wavenumber range 4000–650 cm−1 regions with 16 scans at 4 cm−1 resolution using an FTIR spectrometer (Model: Nexus Analytics-Isio 71,360, Malaysia) with altered total reflectance capabilities.
Field emission scanning electron microscope (FESEM) and energy dispersive spectroscopy (EDS)
Morphological characteristics analysis of the SPFA sample was carried out with the assistance of a Field emission scanning electron microscope (FESEM) (Model: Coxem-EM-30A×+) at a magnification of 100,000× in secondary mode. The FESEM apparatus was employed with an emission current of 58 A, a working distance of 14.7 mm, and an accelerating voltage of 20.0 kV in accordance with Atiqah, Mohammad Jawaid, and Ridzwan Ishak (Citation2018). The elemental components present in the SPFA sample are recorded by the Energy dispersive spectroscopy (EDS) detector attached to the FESEM apparatus.
Thermal properties
Thermogravimetric analysis (TGA) characterization of SPFA
The thermal stability and degradation pattern of SPFA were determined using TGA analysis based on weight loss by increasing temperature. The analysis was performed in a nitrogen atmosphere, using a TGA analyzer (Model: SDT Q600 V20.9 Build 20) at a heating capacity of 10°C/min. The degradation trend was investigated using an SPFA sample of approximately 9.8160 mg.
Differential scanning calorimetry (DSC)
A differential scanning calorimetry analyzer (Model: Universal V3-9A TA Tool, New Castle, PA, USA) was employed to determine the thermal stability of the SPFA sample after being conditioned at 52% for 48 h. In the machine’s configuration, indium was employed for the standard test. SPFA sample of 5 mg was weighed and placed on a hermetically sealed aluminum sample pan during the DSC experiment. The heating of the SPFA was carried out within a temperature range of 35–265°C at the rate of 10°C/min. To maintain an inert atmosphere, the DSC cell was flooded with nitrogen gas, and the thermogram data were determined using transfer temperatures.
Results and discussion
X-Ray diffraction (XRD) of SPFA
The XRD characterization was conducted to investigate the diffraction pattern, crystallographic structure, and particle size of the SPFA. In the XRD diffraction patterns of SPFA depicted in , there are three major strongest peaks observed at the corresponding angles of 29.4, 39.5, and 43.4 °. The quartz (Q) peaks were noticed to be the major constituent as shown in the XRD diffraction pattern () having a hexagonal crystal system with fewer traces of minor peaks of Albite (NaAlSi3O8) (A) and Wollastonite (CaSiO3) (W) both having triclinic crystal system. The broad diffraction peaks in the XRD patterns which corresponded to quartz (Q) confirmed the presence of silica (SiO2) which is amorphous in nature. Ikubanni et al. (Citation2020) and Salakhum et al. (Citation2018) revealed that the broad diffraction peaks confirmed the presence of amorphous silica while crystalline silica happened to be those with minor peaks. When the thermal treatment was conducted over and temperature range of 450 to 700°C, according to Ziegler et al. (Citation2021), the silica formed was predominantly amorphous. As seen from the XRD diffraction patterns (), the quartz (Q) (silica) peaks predominated which confirmed silica to be the most abundant constituent in SPFA. Aigbodion et al. (Citation2018) found SiO2 and NaAlSi3O8, as well as other chemicals including CaCO3 and Al4O4C, in the bean pod ash. From the peaks in the XRD diffraction patterns and employing the Scherrer equation, the calculated average grain size of the SPFA was found to be 41.25 nm.
Fourier transformation infrared (FTIR) of SPFA
FTIR spectra were used to examine the mineralogical composition of SPFA because each mineral has its individual absorption pattern in the infrared spectrum. The functional groups that were present in the SPFA after thermal treatment were represented in the FTIR spectra in in the form of broad bands. The broad peak at 3415.12 cm−1 in a range of 3500–3000 cm−1 indicates the stretching and bending of H2O molecules associated with physio-absorbed moisture on the surface of the sample. There were no C=C, C=O, C-H, and C-O-C bending and stretch vibrations experienced due to the burning of carbonaceous material during the carbonization process. The carbonization process is thought to be the cause of the decrease in organic components such as hemicelluloses and lignin linked with cellulose in sugar palm fiber, which created modest peaks in the 3415.12 to 1800.50 cm−1 range. Low-intensity stretch vibrations of the C-O bond occurred at 1800.50 cm−1 in the FTIR spectra of SPFA. The peak bond at 1647.05 cm−1 was caused as a result of O-H stretching and bending vibrations of Si-OH silanol groups, including HO-H vibrations of absorbed H2O molecules on the surface of the SPFA sample (Costa and Paranhos Citation2018; Imoisili, Ukoba, and Jen Citation2020; Santana). There was evidence of the formation of carbon-based linkages in SPFA that was caused by the degradation of cellulose and lignin into smaller molecules. In addition, there was also an asymmetric stretching and vibration bond of Si-O-Si known as the siloxane group at the strong broad peak around 1431.19 cm−1, and the prominent broad peak around 1056.20 cm−1 was assigned to symmetric stretching and vibration of Si-O bond. Santana Costa and Paranhos (Citation2018) reported similar asymmetric stretching vibration of the Si-O-Si bond around 1093 cm−1 while a symmetric stretch vibration of the Si-O bond was found around 807 cm−1. There was a partial C – O adsorption attributed to CO2 from the atmosphere during the FTIR procedure at the broad peak of 798.71 cm−1, in addition to the O-Si – O bond bending and vibration at the broad peak of 709.61 cm−1, confirming the existence of amorphous silica (Costa et al. Citation2015; Valencia-Saavedra, Mejie De Gutierrez, and Gordillo Citation2018).
Field emission scanning electron microscopy (FESEM) and energy dispersive spectroscopy (EDS) of SPFA
FESEM and EDS are employed to analyze the morphological and chemical constituents, respectively, of SPFA particles as shown in . The morphology of the SPFA () was observed to have particles of irregular shapes of varying sizes and with minor quantities of longitudinal-shaped particles. The SPFA particles, which appeared grayish and were thought to be silica compounds that agglomerated to each other, thereby giving rise to the features as shown in , and were held firmly in a dark void-like matrix with pores of around 1 µm diameter that were visible in some areas.
The presence of more minerals in SPFA other than C, O, and Si was revealed by energy dispersion spectroscopy (EDS) (). The EDS spectrum revealed that Si (silica) had the highest elemental intensity which confirmed its presence in SPFA, along with notable traces of contaminants. In the EDS test, the presence of oxygen suggested a close interaction with Si, similar to silica (SiO2). The elemental breakdown of SPFA is depicted in in terms of percentage. The most abundant element in SPFA is silica (SiO2, 66.03%), which is similar to other siliceous pozzolans such as rice husk ash. This confirmed that silica (SiO2) is the most abundant element found in the SPFA with different amounts of Ca, C, Mg, Al, and other inorganic contaminants.
Table 1. The chemical makeup of sugar palm fiber ash (SPFA).
Thermogravimetric (TGA) and diffraction scanning calorimetry (DSC) of SPFA
Thermogravimetric is a common method for determining weight loss in relation to time or temperature. The TGA curve shown in depicts the thermal stability and degradation pattern of SPFA in the temperature range of 25–450°C at a rate of 10°C/min. The weight loss is categorized into three stages as depicted in . The first stage corresponded to a maximum weight loss of 0.3010 mg (3.0664%) which occurred at a temperature below 221°C and was attributed to physically adsorbed water. The physically adsorbed water from the SPFA evaporated completely at 221°C. Then, at temperatures ranging from 221 to 317.73°C, the second stage of speedy mass deterioration occurred at a shorter time of approximately 10 min. A weight loss of 0.1387 mg (1.4132%) was experienced in the second stage and this could be a result of the breakdown of organic compounds that were associated with the decomposition of lignin, cellulose, and hemicellulose (Anuar et al. Citation2020). The breakdown of lignocellulose materials happened between 300 and 350°C, according to a certain researcher who investigated the combustion kinetics of natural resource materials (Saba et al. Citation2015). Finally, at the temperature range of 430.13 to 453.69°C, the third stage exhibited a slight weight loss of 30.9461 х 10−3 mg (0.3153%), which could be attributed to chemically bonded water. At temperatures above 453.69°C, the volatile components were completely transformed into char without any significant weight loss (Alias et al. Citation2014; Emdadi et al. Citation2015). The remaining weight of 9.3480 mg (95.23%) was accounted for by the ash product, commonly known as the residue remnant.
Differential scanning calorimetry was employed to determine the rate of endothermic or exothermic heat production. The DSC curve for the SPFA (), shows a distinctive broad band around 82.70°C, which corresponded to water evaporation. This validates the presence of water molecules in the SPFA sample under investigation from the FTIR analysis. The temperature in the range of 317.73 to 430.36°C, having a mass loss of 0.27 mg, resulted in an endothermic peak at a temperature of 376.53°C which could be attributed to the disintegration of carbonate, especially calcium carbonate, the reactivity of calcium oxide, and other oxides formed with other elements such as silicon to produce silicate at higher temperatures (Febrero et al. Citation2014). Ma et al. (Citation2017) observed a similar endothermic peak when performing a thermal investigation on a biomass ash sample. There are changes in endothermic temperature based on the biomass ash investigated. Rice husk ash has an endothermic peak at a temperature of 100°C, which corresponded to the decomposition of K-struvite in the temperature range of 50–200°C (Liu et al. Citation2020).
A comparison of the current work to recently published studies employing various silica extraction routes from natural fiber and SiO2 yield is shown in . After carbonization and subsequent treatment with NaOH and H2SO4, the silica content of bamboo leaf ash was found to be high (Sapawe Citation2018). In another study, a significant amount of silica was obtained using only a carbonization treatment at 800°C (Usman et al. Citation2020). Finally, Okoronkwo, Imoisili, and Olusunle (Citation2013) found an adequate amount of silica in corn cob ash after carbonization at 700°C and treated with NaOH, whereas Oladele and Moses Okoro (Citation2016) found a low amount of silica even after the ash was treated with NaOH after being fired in the furnace. In this study, a high amount of silica (66.03%) was recorded with the carbonization and calcination process without the use of any chemical.
Table 2. Comparison of recently reported studies with the current work on the amount of silica obtained from natural fibers.
Characteristics possessed of silica for industrial application
Silica possessed significant characteristics such as chemical inertness, high melting point, biocompatibility, strong surface absorption force, large specific surface area, large surface energy, excellent dispersion performance, thermal conductivity, etc. that enable its use in different industrial applications (Nan et al. Citation2018; Xu et al. Citation2019).
Advantages of silica produced from SPFA
The silica produced from SPFA with its unique characteristics distinguishes it as a material for varieties of industrial applications such as electronic packaging, fillers for plastic composite, glass manufacturing, abrasive for toothpaste, water filtration, and purification, tiles manufacturing, drug delivery, raw material for fertilizer, pesticide against insects, additive in paint, refractory material, fire extinguisher, etc. (Anggria, Husnain, and Masunaga Citation2021; Bakar, Yahya, and Neon Gan Citation2016; Pode Citation2016; Shamsutdinov et al. Citation2021; Thabet et al. Citation2021).
Weaknesses of silica produced from SPFA
Amorphous mesoporous silica can be obtained from agrarian waste such as sugar palm fiber ash, rice husk ash, palm kernel shell ash, etc. Silica comprises fewer weaknesses in terms of its application in areas such as rubber production. The mechanical properties of vulcanizates strengthened with silica were found to be inferior as compared to those strengthened with carbon black (Song, Song, and Zhang Citation2020). This was due to the fact that silica is highly polar and hydrophilic in nature causing it to be incompatible with natural rubber thereby making it a weak filler material (Surya, Ismail, and Azura Citation2013). Consequently, silica has strong interaction between its particles forming large aggregates without uniformly dispersing throughout the rubber matrix. This might have been caused by the hydrogen bonding of the silanol groups on the silica particle surfaces. These weaknesses of silica associated with incompatibility and agglomerate formed can be minimized by the use of silane-coupling reagents such as stearyl alcohol, mercaptopropyltriethoxysilane, bis[3-(triethoxysilyl)propyl]tetrasulfide, etc. (Surya, Ginting, and Purwandari Citation2019; Zhong et al. Citation2019).
Conclusion
The sugar palm fiber was successfully carbonized, calcined, and characterized using XRD, FTIR, SEM, EDX, TGA, and DCS. The XRD patterns of the sugar palm fiber ash revealed the presence of silica (SiO2) as a major constituent and other trace compounds such as NaAlSi3O8 and CaSiO3. The degradation pattern of SPFA confirmed it to exhibit an endothermic system. The SPFA morphology revealed irregular particle shapes of varying sizes with few pores, and EDS confirmed the presence of hard particles such as SiO2, Fe2O3, and Al2O3, which can be used as particulate reinforcement in MMCs in the fabrication of AMC. The FTIR analysis revealed functional groups such as Si-O-Si, C-O, O-H, and Si-O present in SPFA. Due to the high substantial amount of silica, sugar palm fiber ash could be an economically viable material for the production of silica material and the characteristics of silica enable its wide applications in industries.
Supplemental Material
Download Zip (144.6 KB)Supplementary material
Supplemental data for this article can be accessed online at https://doi.org/10.1080/15440478.2023.2170943
Disclosure statement
No potential conflict of interest was reported by the authors.
Additional information
Funding
References
- Adeleke, A. A., J. K. Odusote, P. P. Ikubanni, O. A. Lasode, M. Malathi, and D. Paswan. 2020. The ignitability, fuel ratio and ash fusion temperatures of torrefied woody biomass. Heliyon 6 (3):e03582. doi:10.1016/j.heliyon.2020.e03582.
- Aigbodion, V. S., O. J. Agunsoye, R. O. Edokpia, and I. C. Ezema. 2018. Performance analysis of a connecting rod produced with Al-Cu-Mg/Bean pod ash nanoparticles. Silicon 10 (1):107–13. doi:10.1007/s12633-015-9382-8.
- Alaneme, K. K., M. O. Bodunrin, and A. A. Awe. 2018. Microstructure, mechanical and fracture properties of groundnut shell ash and silicon carbide dispersion strengthened aluminium matrix composites. Journal of King Saud University - Engineering Sciences 30 (1):96–103. doi:10.1016/j.jksues.2016.01.001.
- Alias, N., N. Ibrahim, M. K. Abd Hamid, H. Hasbullah, R. R. Ali, A. N. Sadikin, and U. A. Asli. 2014. Thermogravimetric analysis of rice husk and coconut pulp for potential biofuel production by flash pyrolysis. Malaysian Journal of Analytical Sciences 18 (3):705–10.
- Ananthi, A., D. Geetha, and P. S. Ramesh. 2016. Preparation and characterization of silica material from rice husk ash – an economically viable method. Chemistry and Material Research 8 (6):1–7.
- Anggria, L., Husnain, and T. Masunaga. 2021. A method for production of pure silica as fertilizer from industrial waste material. IOP Conference Series: Earth and Environmental Science 648 (1):012213. doi:10.1088/1755-1315/648/1/012213.
- Anuar, M. F., Y. Wing Fen, M. Hafiz Mohd Zaid, K. Amin Matori, and R. Emilia Mohamed Khaidir. 2020. The physical and optical studies of crystalline silica derived from the green synthesis of coconut husk ash. Applied Sciences 10 (6):2128. doi:10.3390/app10062128.
- Armynah, B., Z. D. Atika, W. H. Piarah, and D. Tahir. 2018. Analysis of chemical and physical properties of biochar from rice husk biomass. Journal of Physics Conference Series 979 (1):012038. doi:10.1088/1742-6596/979/1/012038.
- Athinarayanan, J., V. Subbarayan Periasamy, M. Alhazmi, K. A. Alatiah, and A. A. Alshatwi. 2015. Synthesis of biogenic silica nanoparticles from rice husks for biomedical applications. Ceramics International 41 (1):275–81. doi:10.1016/j.ceramint.2014.08.069.
- Atiqah, A., S. M. S. Mohammad Jawaid, and M. Ridzwan Ishak. 2018. Effect of surface treatment on the mechanical properties of sugar palm/glass fiber-reinforced thermoplastic polyurethane hybrid composites. BioResources 13 (1):1174–88. doi:10.15376/biores.13.1.1174-1188.
- Attol, D. H., and H. Hamied Mihsen. 2019. SYnthesis of silica-salen derivative from rice husk ash and its use for extraction of divalent metal ions co(ii), ni(ii) and cu(ii). Indonesian Journal of Chemistry 20 (1):16. doi:10.22146/ijc.38558.
- Bakar, R. A., R. Yahya, and S. Neon Gan. 2016. Production of high purity amorphous silica from rice husk. Procedia Chemistry 19:189–95. doi:10.1016/j.proche.2016.03.092.
- Bardalai, M., and K. D. Mahanta. 2016. Characterization of rice husk through x- ray diffraction, scanning electron microscope and Fourier transform infrared analysis. International Journal of Innovative Research in Science and Engineering 2 (5):472–79.
- Bisht, N., P. Chandra Gope, and N. Rani. 2020. Rice husk as a fibre in composites: A review. Journal of the Mechanical Behavior of Materials 29 (1):147–62. doi:10.1515/jmbm-2020-0015.
- Channoy, C., S. Maneewan, C. Punlek, and S. Chirarattananon. 2018. Preparation and characterization of silica gel from bagasse ash. Advanced Materials Research 1145:44–48. doi:10.4028/scientific.net/amr.1145.44.
- Costa, J. A. S., A. C. F. S. Garcia, D. O. Santos, V. H. V. Sarmento, M. E. de Mesquita, and L. P. C. Romão. 2015. APplications of inorganic–organic mesoporous materials constructed by self-assembly processes for removal of benzo[k]fluoranthene and benzo[b]fluoranthene. Journal of Sol-Gel Science and Technology 75 (3):495–507. doi:10.1007/s10971-015-3720-6.
- Costa, S. J. A., and C. M. Paranhos. 2018. Systematic evaluation of amorphous silica production from rice husk ashes. Journal of Cleaner Production 192:688–97. doi:10.1016/j.jclepro.2018.05.028.
- Emdadi, Z., N. Asim, M. A. Yarmo, and K. Sopian. 2015. Effect of chemical treatments on rice husk (RH) water absorption property. International Journal of Chemical Engineering and Applications 6 (4):273–76. doi:10.7763/ijcea.2015.v6.495.
- Febrero, L., E. Granada, C. Pérez, D. Patiño, and E. Arce. 2014. Characterisation and comparison of biomass ashes with different thermal histories using TG-DSC. Journal of Thermal Analysis and Calorimetry 118 (2):669–80. doi:10.1007/s10973-014-3717-3.
- Gewaily, E. 2019. Impact of compost rice straw and rice straw as organic fertilizer with potassium treatments on yield and some grain quality of Giza 179 rice variety. Journal Plant Production, Mansoura Univ 10 (2):143–51. doi:10.21608/jpp.2019.36244.
- Ghafar, H., S. N. A. M. Halidi, and M. S. So’aib. 2020. Coconut shell: thermogravimetric analysis and gross calorific value. Proceedings of Mechanical Engineering Research, Kampus Teknologi UteM, Melaka, Malaysia. December: 206–07.
- Hernández-Martínez, D., A. A. Leyva-Verduzco, M.A.E. Francisco Rodríguez-Félix, and F. J. Wong-Corral. 2020. Obtaining and characterization of silicon(Si) from wheat husk ash for its possible application in solar cells. Journal of Cleaner Production 271 (October):122698. doi:10.1016/j.jclepro.2020.122698.
- Huseini, M. R., and G. Fitriyano. 2019. The isotherm studies of adsorbent development from pulogadung primary sewage sludge (PS) with rice straw addition by hydrothermal. Journal of Physics Conference Series 1295 (1):012070. doi:10.1088/1742-6596/1295/1/012070.
- Ikubanni, P. P., M. Oki, A. A. Adeleke, A. A. Adediran, and O. S. Adesina. 2020. Influence of temperature on the chemical compositions and microstructural changes of ash formed from palm kernel shell. Results in Engineering 8 (August):100173. doi:10.1016/j.rineng.2020.100173.
- Ilyas, R. A., S. M. Sapuan, and M. R. Ishak. 2018. Isolation and characterization of nanocrystalline cellulose from sugar palm fibres (arenga pinnata). Carbohydrate Polymers 181 (June 2017):1038–51. doi:10.1016/j.carbpol.2017.11.045.
- Ilyas, R. A., S. M. Sapuan, M. R. Ishak, and E. S. Zainudin. 2018. Development and characterization of sugar palm nanocrystalline cellulose reinforced sugar palm starch bionanocomposites. Carbohydrate Polymers 202 (September):186–202. doi:10.1016/j.carbpol.2018.09.002.
- Imoisili, P. E., K. O. Ukoba, and T.C. Jen. 2020. Synthesis and characterization of amorphous mesoporous silica from palm kernel shell ash. Boletín de La Sociedad Española de Cerámica y Vidrio 59 (4):159–64. doi:10.1016/j.bsecv.2019.09.006.
- Ishak, M. R., Z. Leman, S. M. Sapuan, M. Z. A. Rahman, and U. M. K. Anwar. 2013a. Chemical composition and ft-ir spectra of sugar palm (arenga pinnata) fibers obtained from different heights. Journal of Natural Fibers 10 (2):83–97. doi:10.1080/15440478.2012.733517.
- Ishak, M. R., Z. Leman, S. M. Sapuan, M. Z. A. Rahman, and U. M. K. Anwar. 2013b. Impregnation modification of sugar palm fibres with phenol formaldehyde and unsaturated polyester. Fibers and Polymers 14 (2):250–57. doi:10.1007/s12221-013-0250-0.
- Jadhav, S., A. Aradhye, S. Kulkarni, Y. Shinde, and V. Vaishampayan. 2019. Effect of hybrid ash reinforcement on microstructure of a356 alloy matrix composite. AIP Conference Proceedings 2105 (May). 10.1063/1.5100695.
- Kanthasamy, S., T. S. Ravikumar, and T. Tamilanban. 2020. Withdrawn: Mechanical and corrosion behavior of Groundnut Shell ash particle (GSAp) reinforced AZ31 magnesium composite. Materials Today: Proceedings xxxx:1–5. doi:10.1016/j.matpr.2020.11.834.
- Kumar, S., P. Sangwan, R. Mor, V. Dhankhar, and S. Bidra. 2013. Utilization of rice husk and their ash: A review. Journal of Chemical and Environmental Sciences 1 (5):126–29.
- Liu, Y., Z. He, and M. Uchimiya. 2015. Comparison of biochar formation from various agricultural by-products using FTIR spectroscopy. Modern Applied Science 9 (4):246–53. doi:10.5539/mas.v9n4p246.
- Liu, R., B. Pang, X. Zhao, and Y. Yang. 2020. Effect of rice husk ash on early hydration behavior of magnesium phosphate cement. Construction and Building Materials 263:120180. doi:10.1016/j.conbuildmat.2020.120180.
- Lwin, T., T. T. Win, M. L. Wai, and Y. M. Maung. 2019. Preparation and characterization of activated carbon derived from rice husk biomass. Journal Myanmar Academy of Arts and Science XVII (3):195–207.
- Ma, X., L. Fenghai, M. Mingjie, and Y. Fang. 2017. Investigation on blended ash fusibility characteristics of biomass and coal with high silica-alumina. Energy & Fuels 31 (8):7941–51. doi:10.1021/acs.energyfuels.7b01070.
- Manimaran, P., S. P. Saravanan, M. R. Sanjay, S. Siengchin, M. Jawaid, and A. Khan. 2019. Characterization of new cellulosic fiber: dracaena reflexa as a reinforcement for polymer composite structures. Journal of Materials Research and Technology 8 (2):1952–63. doi:10.1016/j.jmrt.2018.12.015.
- Mukhtar, I., Z. Leman, M. R. Ishak, and E. S. Zainudin. 2018. Thermal and physicochemical properties of sugar palm fibre treated with borax. IOP Conference Series: Materials Science and Engineering 368 (June):012038. doi:10.1088/1757-899X/368/1/012038.
- Nan, J., R. Shang, S. G. J. Heijman, and L. C. Rietveld. 2018. High-Silica Zeolites for Adsorption of Organic Micro-Pollutants in Water Treatment: A Review. Water Research 144 (November):145–61. doi:10.1016/j.watres.2018.07.017.
- Narayan, R., U. Nayak, A. Raichur, and S. Garg. 2018. Mesoporous silica nanoparticles: a comprehensive review on synthesis and recent advances. Pharmaceutics 10 (3):118. doi:10.3390/pharmaceutics10030118.
- Ochuokpa, E. O., D. S. Yawas, P. U. Okorie, and M. Sumaila. 2021. Evaluation of Mechanical and Metallurgical Properties Al- Si-Mg/Mangiferaindica Seed Shell Ash (MSSA) Particulate Composite for Production of Motorcycle Hub. ATBU Journal of Science, Technology and Education 9 (1):221–38.
- Okoronkwo, E. A., P. E. Imoisili, and S. O. O. Olusunle. 2013. Extraction and characterization of amorphous silica from corn cob ash by sol-gel method related papers. Chemistry and Materials Research 3 (4):6.
- Oladele, I. O., and A. Moses Okoro. 2016. The effect of palm kernel shell ash on the mechanical properties of as-cast aluminium alloy matrix composites. 5 (28): 15–30. doi:10.7726/ajmst.2016.1002.
- Pode, R. 2016. Potential applications of rice husk ash waste from rice husk biomass power plant. Renewable and Sustainable Energy Reviews 53:1468–85. doi:10.1016/j.rser.2015.09.051.
- Raju, S. S., and G. S. Rao. 2017. Assessments of Desirability Wear Behaviour on Al-Coconut Shell Ash - Metal Matrix Composite using Grey - Fuzzy Reasoning Grade. Indian Journal of Science and Technology 10 (15):1–11. doi:10.17485/ijst/2017/v10i15/113826.
- Saba, N., M. Paridah, K. A. Tahir, and N. Azowa Ibrahim. 2015. Preparation and characterization of fire retardant nano-filler from oil palm empty fruit bunch fibers. BioResources 10 (3):4530–43. doi:10.15376/biores.10.3.4530-4543.
- Salakhum, S., T. Yutthalekha, M. Chareonpanich, J. Limtrakul, and C. Wattanakit. 2018. Synthesis of hierarchical faujasite nanosheets from corn cob ash-derived nanosilica as efficient catalysts for hydrogenation of lignin-derived alkylphenols. Microporous and Mesoporous Materials 258:141–50. doi:10.1016/j.micromeso.2017.09.009.
- Sanjay, M. R., G. R. Arpitha, L. Laxmana Naik, K. Gopalakrishna, and B. Yogesha. 2016. Applications of natural fibers and its composites: an overview. Natural Resources 07 (03):108–14. doi:10.4236/nr.2016.73011.
- Sanyang, M. L., S. M. Sapuan, M. Jawaid, M. Ridzwan Ishak, and J. Sahari. 2016. Effect of sugar palm-derived cellulose reinforcement on the mechanical and water barrier properties of sugar palm starch biocomposite films. BioResources 11 (2):4134–45. doi:10.15376/biores.11.2.4134-4145.
- Sapawe, N. 2018. Production of silica from agricultural waste. Archives of Organic and Inorganic Chemical Sciences 3 (2):342–43. doi:10.32474/AOICS.2018.03.000160.
- Shamsutdinov, A. S., N. B. Kondrashova, I. V. Valtsifer, Y. H. Edward Bormashenko, E. V. Saenko, A. V. Pyankova, and V. A. Valtsifer. 2021. Manufacturing, properties, and application of nanosized superhydrophobic spherical silicon dioxide particles as a functional additive to fire extinguishing powders. Industrial & Engineering Chemistry Research 60 (32):11905–14. doi:10.1021/acs.iecr.1c01999.
- Sherwani, S. F. K., S. M. Sapuan, Z. Leman, E. S. Zainudin, and A. Khalina. 2021. Physical, mechanical and morphological properties of sugar palm fiber reinforced polylactic acid composites. Fibers and Polymers 0 (0):1–11. doi:10.1007/s12221-021-0407-1.
- Song, P., J. Song, and Y. Zhang. 2020. Stretchable conductor based on carbon nanotube/carbon black silicone rubber nanocomposites with highly mechanical, electrical properties and strain sensitivity. Composites Part B: Engineering 191 (March):107979. doi:10.1016/j.compositesb.2020.107979.
- Surya, I., M. Ginting, and V. Purwandari. 2019. Mechanical properties improvement in silica-filled natural rubber composites using stearyl alcohol. IOP Conference Series: Materials Science and Engineering 509 (1):012054. doi:10.1088/1757-899X/509/1/012054.
- Surya, I., H. Ismail, and A. R. Azura. 2013. Alkanolamide as an accelerator, filler-dispersant and a plasticizer in silica-filled natural rubber compounds. Polymer Testing 32 (8):1313–21. doi:10.1016/j.polymertesting.2013.07.015.
- Terzioglu, P., S. Yucel, T. M. Rababah, and D. Ozcimen. 2013. Characterization of wheat hull and wheat hull ash as a potential source of SiO2. BioResources 8 (3):4406–20. doi:10.15376/biores.8.3.4406-4420.
- Thabet, A. F., H. A. Boraei, O. A. Galal, M. F. M. El-Samahy, K. M. Mousa, Y. Z. Zhang, M. Tuda, E. A. Helmy, J. Wen, and T. Nozaki. 2021. Silica nanoparticles as pesticide against insects of different feeding types and their non-target attraction of predators. Scientific reports 11 (1):14484. doi:10.1038/s41598-021-93518-9.
- Tishkevich, D. I., I. V. Korolkov, A. L. Kozlovskiy, M. Anisovich, D. A. Vinnik, A. E. Ermekova, A. I. Vorobjova, E. E. Shumskaya, T. I. Zubar, S. V. Trukhanov, et al. 2019. Immobilization of boron-rich compound on fe3o4 nanoparticles: stability and cytotoxicity. Journal of Alloys and Compounds 797 (August):573–81. doi:10.1016/j.jallcom.2019.05.075.
- Uda, M. N. A., C. B. G. Subash, N. H. H. Uda Hashim, N. A. Parmin, M. N. Afnan Uda, and P. Anbu. 2021. Production and characterization of silica nanoparticles from fly ash: Conversion of agro-waste into resource. Preparative Biochemistry & Biotechnology 51 (1):86–95. doi:10.1080/10826068.2020.1793174.
- Usama, Z., T. Subhani, and S. Wilayat Husain. 2016. Synthesis and characterization of silica nanoparticles from clay. Journal of Asian Ceramic Societies 4 (1):91–96. doi:10.1016/j.jascer.2015.12.001.
- Usman, Y., E. T. Dauda, M. Abdulwahab, and R. M. Dodo. 2020. Effect of mechanical properties and wear behavior on locust bean waste ash (lbwa) particle reinforced aluminium alloy (A356 alloy) composites. FUDMA Journal of Science 4 (200):416–21.
- Valencia-Saavedra, W., R. Mejía De Gutierrez, and M. Gordillo. 2018. Geopolymeric concretes based on fly ash with high unburned content. Construction and Building Materials 165:697–706. doi:10.1016/j.conbuildmat.2018.01.071.
- Venkatesh, L., T. V. Arjunan, and K. Ravikumar. 2019. Microstructural CHaracteristics and mechanical behaviour of aluminium hybrid composites reinforced with groundnut shell ash and B4C. Journal of the Brazilian Society of Mechanical Sciences and Engineering 41 (7):1–13. doi:10.1007/s40430-019-1800-1.
- Vorobjova, A., D. Tishkevich, D. Shimanovich, M. Zdorovets, A. Kozlovskiy, T. Zubar, D. Vinnik, M. Dong, S. Trukhanov, A. Trukhanov, et al. 2020. Electrochemical behaviour of Ti/Al 2 O 3/Ni nanocomposite material in artificial physiological solution: Prospects for biomedical application. Nanamaterials. 10(173):1–20. doi:10.3390/nano10010173.
- Xu, X., S. Niu, X. Wang, L. Xin, L. Hui, S. Xinghua, and S. Luo. 2019. Fabrication and casting simulation of composite ceramic cores with silica nanopowders. Ceramics International 45 (15):19283–88. doi:10.1016/j.ceramint.2019.06.178.
- Yao, X., K. Xu, and Y. Liang. 2017. Comparative Analysis of the Physical and Chemical Properties of Different Biomass Ashes Produced from. BioResources 12 (2):3222–35.
- Zdorovets, M. V., and A. L. Kozlovskiy. 2020. Surface & coatings technology study of the stability of the structural properties of CeO 2 microparticles to helium irradiation. Surface & Coatings Technology 383 (November 2019):125286. doi:10.1016/j.surfcoat.2019.125286.
- Zhong, B., X. Zeng, W. Chen, Q. Luo, H. Dechao, Z. Jia, and D. Jia. 2019. Nonsolvent-assisted surface modification of silica by silane and antioxidant for rubber reinforcement. Polymer Testing 78 (March):105949. doi:10.1016/j.polymertesting.2019.105949.
- Ziegler, D., F. Boschetto, E. Marin, P. Palmero, G. Pezzotti, and J. Marc Tulliani. 2021. Rice husk ash as a new humidity sensing material and its aging behavior. Sensors and Actuators B, Chemical 328 (June 2020):129049. doi:10.1016/j.snb.2020.129049.