ABSTRACT
Natural cellulosic materials are attractive biopolymers for their naturally abundant source, mechanical toughness, bioactivity, etc. However, porosity and flexibility remain challenges for the preparation of aerogels from natural cellulose. To maintain the characteristics of cellulose aerogels possessing the toughness of fibers and high porosity of aerogels, cellulose aerogels with three-dimensional void structures and high compressive resilience were prepared by mixing cellulose fiber suspension and polyvinyl alcohol (PVA). The PM 2.5 removal rate and thermal conductivity of cellulose aerogel reached 92.4% and 0.028 W/mK, respectively, when a PVA content of 0.7% was added, and the radiation cooling temperature was about 10.0°C lower than that of PE foam. The three-dimensional void structure and surface morphology of the cellulose aerogels were investigated by scanning electron microscopy (SEM). The cellulose aerogel porosity and pore size distribution were evaluated by a mercury intrusion meter. The crystal structure of cellulose was analyzed by X-ray diffraction (XRD). UV-Vis-NIR spectroscopy was used to investigate the infrared reflectance of cellulose aerogels. Then the air purification efficiency and radiation cooling effect of porous cellulose aerogels were evaluated in a homemade device, respectively.
摘要
天然纤维素材料由于其天然丰富的来源、机械韧性、生物活性等,是极具吸引力的生物聚合物. 然而,孔隙率和柔韧性仍然是天然纤维素制备气凝胶的挑战. 为了保持纤维素气凝胶具有纤维韧性和高孔隙率的特性,通过混合纤维素纤维悬浮液和聚乙烯醇(PVA)制备了具有三维孔隙结构和高压缩弹性的纤维素气凝胶. 当PVA含量为0.7%时,纤维素气凝胶的PM 2.5去除率和热导率分别达到92.4%和0.028W/mK,辐射冷却温度比PE泡沫低约10.0°C. 用扫描电子显微镜(SEM)研究了纤维素气凝胶的三维孔隙结构和表面形貌. 纤维素气凝胶的孔隙率和孔径分布通过水银侵入仪进行评估. 通过X射线衍射(XRD)分析了纤维素的晶体结构。采用UV-Vis-NIR光谱法研究了纤维素气凝胶的红外反射率. 然后在自制装置中分别评价了多孔纤维素气凝胶的空气净化效率和辐射冷却效果.
Introduction
Aerogels are a class of ultra-lightweight materials with continuous three-dimensional porous networks, which have attracted much attention for their unique properties in the fields of adsorption and purification (Li et al. Citation2022), thermal insulation (Boukind et al. Citation2021) and energy storage (Zhao, Tang, and Zhang Citation2021). However, aerogels composed of inorganic compounds exhibit high porosity but have strong brittleness as a result of the weak interconnection between the nanoclusters constituting the aerogel (Toivonen et al. Citation2015). In contrast, the three-dimensional void structure of the nano-cellulose aerogel is composed of overlaying and entanglement of saw-toothed nanofibers, which exhibit good flexibility and high toughness (Chen et al. Citation2021b). Therefore, high performance aerogels prepared based on nanofibers cellulose (Song et al. Citation2018), chitin (Zhu et al., Citation2019) have a wider application prospect.
Cellulose, poly-β-(1→4)-D-glucose, is an important component of natural plant fibers. Cellulose nanofibers can exhibit satisfactory mechanical properties and low thermal conductivity for the preparation of aerogels because of their high aspect ratio, abundant surface active groups and unique structure (De France, Hoare, and Cranston Citation2017). However, the natural properties of fibers are greatly disrupted by dissolution when using cellulose materials of natural origin to prepare high-performance aerogels (Wan et al. Citation2019); the further application of aerogels is also limited by their low porosity, weak toughness and high brittleness. Usually, chemical cross-linking improves the mechanical strength of cellulose aerogels through forming strong covalent bonds, but it is difficult to obtain strong entanglement and mechanical stability of such cellulose aerogels in aqueous solutions (Kim, Youn, and Lee Citation2015). Therefore, the porous structure and mechanical properties are limited when preparing aerogels from cellulose solutions.
Cellulose fibers not only have the advantageous abundance and mechanical toughness of natural fibers, but also have biodegradability and good biocompatibility. Although there are many literature reports on the preparation and functionalization of cellulose aerogels, their practical applications are still hindered by the dense stacked 2D structure; meanwhile, freeze-drying may cause mechanical strength deficiencies because it is difficult to obtain strong entanglement and stable cross-linking between the cellulose (Chen et al. Citation2019). Compared to cellulose solutions, the natural toughness and fibrous structure of cellulose fibers are used to prepare functional materials such as films, porous scaffolds and filaments, which can exhibit high porosity and mechanical toughness (Gamage et al. Citation2021). Also, inorganic nanoparticles with high reflectivity are an important way to achieve radiative cooling, while the high emissivity of C-O-C and C-O bonds in cellulose in the infrared region is often neglected (Gamage et al. Citation2020). To obtain high-performance nano-fibrous aerogels from natural cellulose, in this study, cellulose fibers were obtained from natural fiber materials based on an aqueous solution system. Then, a small amount of PVA binder was added to the suspension of cellulose fibers to enhance the stability of the nanofiber aerogels by curing the connections between the fibers with abundant hydroxyl groups. The resulting three-dimensional nano-fibrous aerogels not only maintain the unique properties of fibers, but also have the high porosity and ultra-lightweight properties of aerogels; meanwhile, cellulose aerogels in the infrared region also exhibit unique radiative cooling properties. Therefore, cellulose aerogels are prepared by cellulose fibers to impart high resilience, filtration, and radiation cooling properties.
Experimental
Materials
Cotton linter pulp fibers (origin fibers) was supplied from Shanghai Hengxin Chemical Reagent Co., Ltd. Sodium hydroxide (NaOH), urea, hydrochloric acid, and Poly (vinyl alcohol) (PVA, 1750 Da) was purchased from Sinopharm Chemical Reagent Co.,Ltd. The deionized water with resistivity of 10–16 MΩ·cm (25°C) used in the experiment was taken from the Milli-Q Plus 185 water purification system (Millipore, Bedford, MA).
Preparation of regenerated cellulose fiber
The origin fibers were added to the aqueous urea/NaOH mixed solution, where the urea, NaOH and water were 6.0 g, 9.0 g and 43.0 g, respectively. The mixed solution was kept at −20°C for 6 h. Subsequently, the frozen mixture was vigorously stirred at 20,000 rpm using a homogenizer (S10, Scientz company, Ningbo, China) until the origin fibers were completely dissolved. 0.5 M (12 mL) hydrochloric acid solution was added dropwise to the cellulose solution until neutral. The regenerated cellulose was collected by filtration, washed and soaked three times with deionized water, and then dried at 40°C for 8 h.
Preparation of the natural regenerated cellulose fiber aerogels
The suspension of purified regenerated cellulose fibers was mixed with the PVA solution by stirring. The mass ratios of PVA and cellulose fibers in the mixed solution were controlled at 0, 0.3, 0.5, 0.7, and 1.0 wt.%, respectively. The mixed solution was rapidly poured into metal container and frozen at −80°C for 24 hours. Subsequently, the cellulose aerogels were obtained by freeze-drying for 48 hours. The preparation process of natural cellulose aerogel as revealed in . The above mixed solution is named CR-n %-PVA, where n represents the concentration of PVA from 0 to 1.0. Thereafter, the mixed solutions were freeze-dried to obtain CR-n %-PVA aerogels.
Characterization
The surface topography of cellulose aerogels was investigated using a scanning electron microscope (SEM, JSM-6510LV, JEOL Co. Ltd., Japan).
Fourier transform infrared (FTIR, NEXUS 670, Nicolet Co. Ltd., USA) spectroscopy was used to analyze the composition of cellulose aerogels with 64 scans in the scan range from 4000 to 400 cm−1.
X-ray diffractometer (XRD, D8 Advance, Bruker GmbH, Germany) was used to investigate the crystal structure of cellulose and regenerated cellulose. Diffraction peaks were recorded in the range of 10–80° with a scanning speed of 5 °/min under a Cu-Kα radiation source.
The pore size distribution and pore area of the regenerated cellulose membranes were recorded using a high-performance automatic mercury porosimeter (Autopore IV 9500, Micrometrics, USA).
The compression properties of the cellulose aerogel samples were investigated on a universal testing machine (Instron 5965, Norwood, MA, USA) equipped with two metal plates and a 500 N sensor. All samples used for compression tests were cylindrical 40 mm × 20 mm with a compression rate of 5 mm·min−1(Compression deformation of 10–60%). Three compression tests were performed for each set of samples. The CR-0.0%-PVA and CR-0.7%-PVA samples were subjected to tensile tests at 5 mm·min−1 with a chuck sensitivity and chuck distance of 500 N and 2 cm, respectively. Tensile tests were performed at least three times for each set of samples.
Thermal conductivity of cellulose aerogels was evaluated at room temperature with a thermal constant analyzer (TPS 2500S, Hot Disk, Sweden).
The UV-Vis-NIR spectrophotometer equipped with an integrating sphere (Lambda 35, PerkinElmer, USA) was used to measure the reflectance of cellulose aerogels.
A self-made closed device was constructed to examine the cooling properties of cellulose aerogels. A film with a thickness of 0.5 cm is placed in polystyrene foam wrapped with aluminum foil, and then the upper space of the device is sealed using a transparent polyethylene film. A xenon lamp (PL-XQ500W, Princes Technology Co., Ltd. China) was used as a radiation source to simulate sunlight. The real-time temperature of the aerogel films was collected using a thermocouple-connected digital thermometer (PM6501, Huayi Instruments, China). Infrared images of the aerogels were acquired by an infrared thermal camera (TiS65, Fluke, US).
The self-made equipment for the purification test of particulate matter (PM) by cellulose aerogels. Cigarettes (Yellow Crane Tower, China) are used to simulate a harmful smoke particle environment. The PM values before and after filter filtration were recorded by an air quality monitor (BOHU-A1, China) with a nitrogen flow rate of 0.1 Mpa. Repeat the measurement for all filter elements at least 5 times.
Simulations of cellulose aerogel filtered PM 2.5 was performed using the fluid dynamics (CFD) module in COMSOL Multiphysics 6.4 software. Among them, the gas flow rate was set to 0.53 m/s and the airflow field around the filter was calculated as a single-phase laminar flow.
Results and discussion
Surface morphology of cellulose aerogels
The surface of the origin fibers presents an intricate fiber structure, and the fiber diameter was about 13 μm (). The cellulose aerogel does not have a fibrous structure, and its surface has many random three-dimensional void structures. Notably, the concentration of PVA affected the void size and structure distribution of the cellulose aerogel. The surface structure of CR-0.0%-PVA has the phenomenon of large cavity spacing and mutual entanglement, while CR-0.7%-PVA distributes a more three-dimensional cavity structure. As the PVA concentration reaches 1.0 wt. %, the surface of CR-1.0%-PVA is coated with a layered structure of thin films. The above results indicate that the three-dimensional network structure of cellulose aerogels could be adjusted by the PVA concentration.
XRD analysis
The origin fiber revealed characteristic diffraction peaks at 14.6°, 16.5°, 22.6° and 34.4° (), which were assigned to the (1–10), (110), (200), and (004) lattice planes of cellulose I, respectively (Sun et al. Citation2016). While CR-n %-PVA presented characteristic peaks at 12.3°, 20.1° and 22.0°Corresponding to the (1–10), (110) and (020) lattice planes of cellulose II, respectively (Huang et al. Citation2020). The above results confirmed that the crystal structure of natural fibers before and after regeneration was converted from cellulose I to cellulose II.
FTIR analysis
shown the FTIR spectra of origin fibers and CR-n %-PVA, respectively. The enhancement of the absorption peak of CR-0.7%-PVA at 3341 cm−1 may be due to the hydroxyl stretching vibration of PVA. Compared with the original fiber, the new characteristic peaks at 1660 cm−1 for CR-0.7%-PVA can be attributed to the C-O stretching vibration of urea, indicating that urea plays an important role in cellulose dissolution (Fauziyah et al. Citation2019). In addition, the weak peak at 1099 cm−1 may be attributed to the carbon-oxygen bond stretching vibration of PVA (Huang, Hu, and Wei Citation2016).
Porosity analysis
The pore size distribution of CR-0.7%-PVA ranges from 1 to 100 μm, and the median pore size is about 79.6 μm (). The porosity, bulk density, and BET surface area of CR-0.7%-PVA were 92.5%, 0.0273 g/ml, and 230 m2/g, respectively. These results indicate that cellulose aerogels have abundant porous structures, which not only endow them with excellent softness and toughness, but also available the possibility of adsorption. CR-0.7%-PVA on dandelion and azalea confirmed the lightweight properties of cellulose aerogels, as shown in .
Mechanical property of cellulose aerogel
shows the tensile properties of CR-0.0%-PVA and CR-0.7%-PVA. CR-0.7%-PVA exhibits tensile fracture when the strain reaches about 10.0%, while CR-0.0%-PVA tensile fracture occurs near 5.0%. Meanwhile, the tensile rupture strength of CR-0.7%-PVA is 1.5 times higher than that of CR-0.0%-PVA. These results demonstrate that the addition of PVA effectively improves the mechanical strength of cellulose aerogels. In addition, the compressive strength of the cellulose aerogel at 50% strain increased from 0.019 to 0.138 MPa as the PVA concentration increased from 0 to 0.7 wt% (). reveals the relationship between the three-dimensional void structure and compression resilience of the aerogel. In the initial stage of aerogel strain, the major pores are strongly deformed. Second, as the compressive strength continues to increase, the minor pores begin to deform.
Figure 3. A) Tensile stress of CR-0.0%-PVA and CR-0.7%-PVA; b) Compressive stress of CR-n %-PVA; c) Schematic illustration of aerogel deforming during compression; d) Compression-rebound curves of CR-0.7%-PVA at 60% strain for 50 cycles.
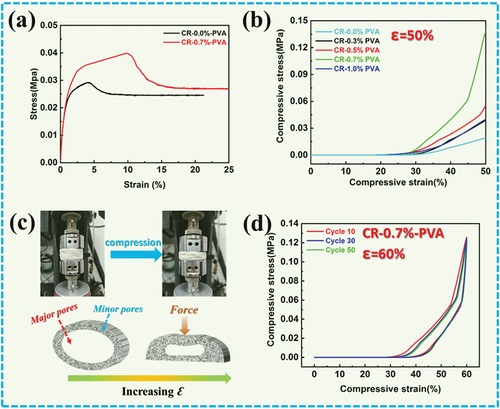
CR-0.7%-PVA still displayed good resilience after 50 compression cycles at 60% strain (). Among them, the compressive stress of CR-0.7%-PVA remains above 84.0% after 50 compression cycles. Therefore, CR-0.7%-PVA exhibited good durable resilience may be ascribed to the three-dimensional void structure providing deformation recovery.
Radiative cooling of aerogels
The thermal conductivity of the cellulose aerogel gradually decreased as the PVA concentration increased from 0.0% to 0.7% as revealed in . This is mainly attributed to the formation of three-dimensional void structures in cellulose aerogels by the addition of PVA. Moreover, the micro-pores of the three-dimensional void structure are close to the mean free path of air molecules, which helps to reduce the thermal conductivity of the aerogel (Beluns et al. Citation2021). Therefore, the thermal conductivity of CR-0.7%-PVA (0.028 W·m−1·K) at room temperature is similar to that of air (0.023 W·m−1·K.
Figure 4. A) Thermal conductivity of CR-n %-PVA; b) Reflectance spectra of CR-0.0%-PVA and CR-0.7%-PVA in the UV-Vis-NIR region; c) Real-time temperature changes of origin fibers, CR-0.0%-PVA, CR-0.7%-PVA and PE aerogel within 60 min; d) Schematic of radiation cooling device; d) Infrared images of origin fibers, CR-0.0%-PVA, CR-0.7%-PVA and PE aerogel after 60 min irradiation.
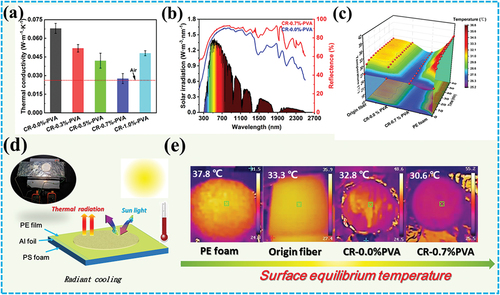
displays the reflectance spectra of CR-0.0%-PVA and CR-0.7%-PVA in the UV-Vis-NIR region. Cellulose aerogels with and without PVA have a good light reflection in the solar spectral range (0.3–2.5 μm) (). This is mainly due to the strong reflection formed by molecular vibration and stretching of cellulose (Chen et al. Citation2021a). However, the reflectivity of CR-0.7%-PVA is higher than that of CR-0.0%-PVA in the near-infrared region, because the close-packed pores can improve the reflective interface and scattering ability inside the aerogel, thereby decreasing the thermal radiation absorption ability (Q. Cui et al. Citation2018; Li et al. Citation2022) Therefore, the three-dimensional void structure of cellulose aerogels endows them with radiative cooling properties.
To verify the radiative cooling performance of cellulose aerogel, real-time thermal monitoring was performed in a homemade thermal insulation device (). The equilibrium temperature of the original fiber after 60 min of radiation is 31.5°C, significantly lower than the air temperature (39.8°C) under PE shielding (Figure S1). Compared to the equilibrium temperature (60 min) of origin fiber, CR-0.0%-PVA and CR-0.7%-PVA were 30.8 and 26.7°C (), respectively. This indicates that cellulose aerogels with a three-dimensional void structure exhibit stronger radiative cooling properties. It is worth noting that the equilibrium temperature (36.7°C) of commercialized PE foam is significantly higher than that of origin fiber, which further confirms the high reflectivity of cellulose to sunlight. After 60 min irradiation, the surface temperatures of commercialized PE foam and origin fibers reached 37.8 and 33.3°C, respectively, while the surface temperature of CR-0.7%-PVA is only 30.6°C as revealed in . These results confirm that cellulose molecules and the three-dimensional void structure jointly determine the radiative cooling effect.
Filtration performance of cellulose aerogel
A home-made particulate purification device to evaluate the filtration performance of cellulose aerogels as displayed in . During the test, the airtight container on the right was filled with high-concentration particulate matter and then filtered through the connected cellulose aerogel filter, and the PM values in the two containers were observed. The three-dimensional void structure and active groups of cellulose aerogels can effectively adsorb and filter particles of different sizes and properties () (Zhu et al. Citation2019).
Figure 5. A) Homemade device for particulate pollutant filtration test; b) Schematic of cellulose aerogel filtering particulate pollutants; c) Filtration efficiency of CR-n %-PVA for particles pollutant with different particle diameter; d-e) the micromorphology before and after filtration of CR-0.7%-PVA, respectively; f) Simulation of cellulose aerogel filtrated PM 2.5 (filtering for 5 min).
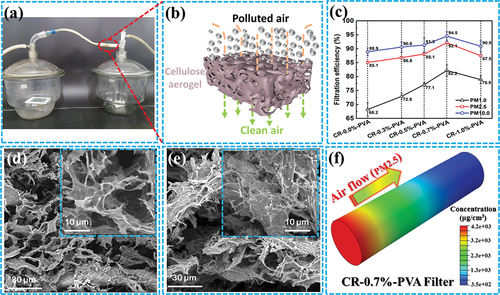
The diameter of particulate pollutants is a key factor affecting the filtration efficiency of CR-n %-PVA. It can be seen that the removal efficiency of all filters to PM 10.0 is higher than that of PM 1.0 and PM 2.5. This suggests that larger particulate pollutants are more easily blocked by cellulose aerogels. PM 10.0, PM 2.5 and PM 1.0 removal rates of CR-0.7%-PVA were 94.5%, 92.4% and 82.2%, respectively. Compared to the reported filter materials, CR-0.7%-PVA has a significant advantage in terms of particle removal efficiency (Table S1). In addition, the pressure drop of CR-0.7%-PVA filter was monitored at 28.3 Pa (filtered for 30.3%), indicating that the CR-0.7%-PVA can filter particulate pollutants with a low pressure-drop under the air flow rate of 32 L/min.
displays that the surface of CR-0.7%-PVA before filtration exhibits many pore structures formed by closed regions, which effectively improve the contact area between the cellulose aerogels and particulate sediment. In contrast, collapsed closed areas and covered deposits were observed on the filtered CR-0.7%-PVA surface (), which proved that cellulose aerogel has an obvious filtering effect on particulate pollutants.
Based on the initial concentration of PM 2.5 and the structure and airflow parameters of CR-0.7%-PVA, the PM 2.5 capture and concentration distribution of the filter within 5 min were simulated, as displayed in and Figure S2. Obviously, the CR-0.7%-PVA filter gradually decreases the PM 2.5 concentration distribution from left to right, and the high concentration area in the filter gradually increased with the prolongation of filtration time (Figure S2). These results indicate that the particulate pollutants are blocked and adsorbed in the three-dimensional void structure of the cellulose aerogel.
Conclusion
In this research, we demonstrate the natural cellulose-based fibers to form cellulose aerogels with reliable three-dimensional void structures and compression resilience. The high adhesion of PVA between nanofibers could endow the aerogel with a stronger binding force and facilitate the formation of three-dimensional void structures. Controlled by the internal three-dimensional void structure and the properties of cellulose, cellulose aerogels can be prepared as thermal insulation materials and filter media with high efficiency for filtering particulate pollutants. Among them, the removal efficiencies of CR-0.7%-PVA to PM 10, PM 2.5, and PM 1.0 were 94.5%, 92.4%, and 82.2%, respectively. Meanwhile, the radiation cooling temperature of CR-0.7%-PVA is about 10.0°C lower than that of PE foam. Therefore, these properties would urge natural cellulose aerogels becomes to potential materials for air purifiers and radiative cooling in the future.
Author contributions statement
Sun J: contributed to the theoretical analysis and investigation. Zhou Y and Zhou J: participated in the discussion. Yang H: contributed to the methodology and investigation, and wrote the paper.
Highlights
Preparation of high porosity aerogels by cellulose fiber suspensions
A small amount of PVA linked to the abundant hydroxyl groups on the surface of cellulose fibers can improve the resilience of aerogels.
When PVA was added at a concentration of 0.7 wt.%, the porosity of cellulose aerogel reached 74.5%, the PM 2.5 removal rate reached 92.4%
CR-0.7% -PVA surface radiation cooling temperature decreased by about 13°C compared with the ambient temperature.
Supplemental Material
Download MS Word (15.5 KB)Disclosure statement
No potential conflict of interest was reported by the authors.
Supplemental data
Supplemental data for this article can be accessed online at https://doi.org/10.1080/15440478.2023.2181276
Additional information
Funding
References
- Beluns, S., S. Gaidukovs, O. Platnieks, G. Gaidukova, I. Mierina, L. Grase, O. Starkova, P. Brazdausks, and V. Thakur. 2021. From wood and hemp biomass wastes to sustainable nanocellulose foams. Industrial Crops and Products 170:113780. doi:10.1016/j.indcrop.2021.113780.
- Boukind, S., S. Sair, H. Ousaleh, S. Mansouri, M. Zahouily, Y. Abboudc, and A. Bouari. 2021. Ambient pressure drying as an advanced approach to the synthesis of silica aerogel composite for building thermal insulation. Journal of Natural Fibers 19 (15):1–10. doi:10.1080/15440478.2021.1993486.
- Chen, Y., B. Dang, J. Fu, C. Wang, C. Li, Q. Sun, and H. Li. 2021a. Cellulose-based hybrid structural material for radiative cooling. Nano Letters 21:397–404. doi:10.1021/acs.nanolett.0c03738.
- Chen, Y., L. Zhang, Y. Yang, B. Pang, W. Xu, G. Duan, S. Jiang, and K. Zhang. 2021b. Recent progress on nanocellulose aerogels: Preparation, modification, composite fabrication, applications. Advanced Materials 33 (11):2005569. doi:10.1002/adma.202005569.
- Chen, Y., L. Zhou, L. Chen, G. Duan, C. Mei, C. Huang, J. Han, and S. Shao. 2019. Anisotropic nanocellulose aerogels with ordered structures fabricated by directional freeze-drying for fast liquid transport. Cellulose 26 (11):6653–67. doi:10.1007/s10570-019-02557-z.
- Cui, Y., H. Gong, Y. Wang, D. Li, and H. Bai. 2018. A thermally insulating textile inspired by polar bear hair. Advanced Materials 30:1706807–15. doi:10.1002/adma.201706807.
- De France, K. J., T. Hoare, and E. D. Cranston. 2017. Review of hydrogels and aerogels containing nanocellulose. Chemistry of Materials 29:4609–31. doi:10.1021/acs.chemmater.7b00531.
- Fauziyah, M., W. Widiyastuti, R. Balgis, and H. Setyawan. 2019. Production of cellulose aerogels from coir fibers via an alkali–urea method for sorption applications. Cellulose 26 (18):9583–98. doi:10.1007/s10570-019-02753-x.
- Gamage, S., D. Banerjee, M. Alam, T. Hallberg, C. Åkerlind, A. Sultana, R. Shanker, M. Berggren, X. Crispin, H. Kariis, et al. 2021. Reflective and transparent cellulose-based passive radiative coolers. Cellulose 28:9383–93. doi:10.1007/s10570-021-04112-1.
- Gamage, S., E. S. H. Kang, C. Åkerlind, S. Sardar, J. Edberg, H. Kariis, T. Ederth, M. Berggren, and M. Jonsson. 2020. Transparent nanocellulose metamaterial enables controlled optical diffusion and radiative cooling. Journal of Materials Chemistry C 8 (34):11687–94. doi:10.1039/D0TC01226B.
- Huang, C., K. Hu, and Z. Wei. 2016. Comparison of cell behavior on pva/pva-gelatin electrospun nanofibers with random and aligned configuration. Scientific Reports 6:37960–68. doi:10.1038/srep37960.
- Huang, Z., C. Liu, X. Feng, M. Wu, Y. Tang, and B. Li. 2020. Effect of regeneration solvent on the characteristics of regenerated cellulose from lithium bromide trihydrate molten salt. Cellulose 27:9243–56. doi:10.1007/s10570-020-03440-y.
- Kim, C., H. Youn, and H. Lee. 2015. Preparation of cross-linked cellulose nanofibril aerogel with water absorbency and shape recovery. Cellulose 22 (6):3715–24. doi:10.1007/s10570-015-0745-5.
- Li, J., X. Tang, H. Zhang, X. Gao, S. Zhang, and T. Tan. 2022. Adsorption behavior of three-dimensional bio-adsorbent from maize stalk pith for methylene blue. Industrial Crops and Products 188:115717. doi:10.1016/j.indcrop.2022.115717.
- Song, J., C. Chen, Z. Yang, Y. Kuang, T. Li, Y. Li, H. Huang, L. Kierzewski, B. Liu, S. He, et al. 2018. Highly compressible, anisotropic aerogel with aligned cellulose nanofibers. ACS Nano 12 (1):140–47. doi:10.1021/acsnano.7b04246.
- Sun, B., M. Zhang, Q. Hou, R. Liu, T. Wu, and C. Si. 2016. Further characterization of cellulose nanocrystal (CNC) preparation from sulfuric acid hydrolysis of cotton fibers. Cellulose 23:439–50. doi:10.1007/s10570-015-0803-z.
- Toivonen, M., A. Kaskela, O. Rojas, E. Kauppinen, and O. Ikkala. 2015. Ambient-dried cellulose nanofibril aerogel membranes with high tensile strength and their use for aerosol collection and templates for transparent, flexible devices. Advanced Functional Materials 25:6618–26. doi:10.1002/adfm.201502566.
- Wan, C., Y. Jiao, S. Wei, L. Zhang, Y. Wu, and J. Li. 2019. Functional nanocomposites from sustainable regenerated cellulose aerogels: A review. Chemical Engineering Journal 359:459–75. doi:10.1016/j.cej.2018.11.115.
- Zhao, Z., Z. Tang, and Y. Zhang. 2021. Discarded dates as a sustainable source to prepare porous carbon-aerogel with multiple energy storage functions. Industrial Crops and Products 170:113772–83. doi:10.1016/j.indcrop.2021.113772.
- Zhu, G., Z. Chen, B. Wu, and N. Lin. 2019. Dual-enhancement effect of electrostatic adsorption and chemical crosslinking for nanocellulose-based aerogels. Industrial Crops and Products 139:111580–89. doi:10.1016/j.indcrop.2019.111580.