ABSTRACT
Regulation of translation is essential for the diverse biological processes involved in development. Particularly, mammalian oocyte development requires the precisely controlled translation of maternal transcripts to coordinate meiotic and early embryo progression while transcription is silent. It has been recently reported that key components of mRNA translation control are short and long noncoding RNAs (ncRNAs). We found that the ncRNABrain cytoplasmic 1 (BC1) has a role in the fully grown germinal vesicle (GV) mouse oocyte, where is highly expressed in the cytoplasm associated with polysomes. Overexpression of BC1 in GV oocyte leads to a minute decrease in global translation with a significant reduction of specific mRNA translation via interaction with the Fragile X Mental Retardation Protein (FMRP). BC1 performs a repressive role in translation only in the GV stage oocyte without forming FMRP or Poly(A) granules. In conclusion, BC1 acts as the translational repressor of specific mRNAs in the GV stage via its binding to a subset of mRNAs and physical interaction with FMRP. The results reported herein contribute to the understanding of the molecular mechanisms of developmental events connected with maternal mRNA translation.
Graphical abstract
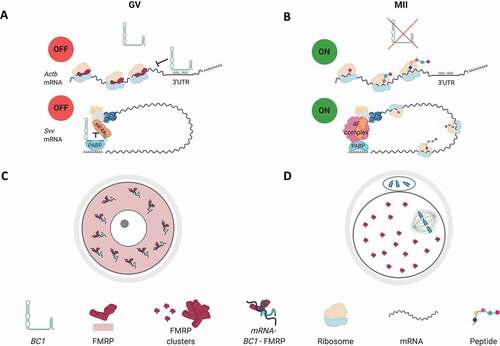
Introduction
Non-coding RNAs (ncRNAs) represent a major part of the transcriptome and exhibit a variety of structures and functions. Despite numerous ncRNAs having emerged and evolved rapidly, they are poorly conserved among mammalian species compared with protein-coding genes [Citation1,Citation2]. Also, whilst the number of reported ncRNAs is rapidly increasing [Citation3], we still only have little data about their cellular functions. Moreover, it is still puzzling as to which ncRNAs are truly functional and which of them represent only ‘transcriptional noise’.
A phylogenetically heterogeneous group of mammalian noncoding Brain Cytoplasmic (BC) RNAs was originally described as controlling translation in dendrites [Citation4]. While BC1 ncRNA is restricted to rodents and BC200 is found in primates, they independently gained translational repression function at a later stage of mammalian evolution when more stringent gene expression control mechanisms were acquired [Citation5]. BC1 ncRNA, which is a 154nt-long Pol III-derived transcript, is a phylogenetic descendant of tRNAAla [Citation6]. BC1 contains a 5ʹ-stem-loop domain followed by a single-stranded central homopolymeric A-rich region and a 3ʹ-stem-loop domain [Citation7]. BC1 was shown to obstruct the formation of the 48S preinitiation complex in cap-dependent translation. This is mediated by a 3ʹ-stem-loop and the adjacent A-rich region through which BC1 interacts with eIF4B, eIF4A and PABP [Citation8–11]. Thus, BC1 negatively modulates translation initiation.
Moreover, in 2003, Zalfa and colleagues reported BC1 acts together with RNA-binding Fragile X Mental Retardation Protein (FMRP) leading to the assembly of large messenger RNPs, in which translation repression of specific mRNA targets occurs [Citation12–16]. FMRP has been shown to orchestrate particular mRNAs by regulating their stability, localization and translation [Citation14]. However, BC1-FMRP interaction is still being described and discussed, as several groups suggest direct physical association of BC1 and FMRP in the rodent brain [Citation12,Citation17] while others propose a sequential model of action of these molecules in the same translational pathway [Citation9,Citation18–20]. BC1 is reported to bind mRNAs which bear U-rich regions through a one-stranded A22 central domain [Citation19]. Phenocopy detected in single BC1 and FMRP knock-outs (KOs), and more severe but still phenocopy observed in double KO animals indicate that the target mRNAs partially overlaps [Citation20–22].
In many mammalian cell types, ncRNA functions remain poorly understood. One significant example is the oocyte. In recent years, the expression of ncRNAs in oogenesis and oocyte maturation has become a focus of attention [Citation23–26]. Meiotic oocyte maturation is a complex process with unique mechanisms leading to the reduction of the genomic content to the haploid state. The fully grown oocyte (‘germinal vesicle (GV)’ oocyte) is transcriptionally silent and utilizes only transcripts synthesized in earlier developmental stages [Citation27]. Thus, reliance on translational control mechanisms throughout the maturation is a key feature of the oocyte [reviewed in Citation28], including the involvement of ncRNAs in mRNA stabilization and translation repression [Citation29].
Here, we analysed the expression and distribution of BC1 ncRNA in the mouse oocyte and its role in the modulation of the translation of the maternal mRNA pool. Our results demonstrate that BC1 plays a role in the regulation of the translation of specific maternal mRNAs in the GV stage without contributing to oocyte developmental competence.
Results
BC1 ncRNA is abundant in the GV oocyte with polysomal association
At first, we analysed the expression of BC1 ncRNA (BC1) in GV and MII oocytes and 2-cell embryos. We found that BC1 is highly expressed in the GV stage with a subsequent significant decrease in the MII stage and 2-cell embryo (). For comparison, we determined levels of Gapdh mRNA and Neat2 lncRNA. Both of them also declined from the GV to 2-cell stage, however, not as dramatically as BC1 (). Next, we focused on BC1 cellular localization in the GV-stage oocyte. We performed qPCR analysis of cDNA samples prepared from nuclear and cytoplasmic fractions and found out that BC1 exhibited exclusive cytoplasmic localization. Gapdh mRNA was present in both the nucleus and cytoplasm in sharp contrast to the strict and well-known nuclear localization of Neat2 lncRNA ( and Figure S1) [Citation30,Citation31].
Figure 1. BC1 ncRNA is abundant in the GV oocyte with polysomal association
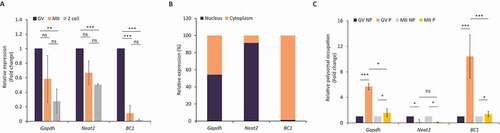
Several previous studies found that BC1 ncRNA acts as a suppressor of translation of specific mRNAs in the brain [Citation22,Citation32]. To test whether BC1 directly impacts translation in the mouse oocyte, we implemented a recently optimized approach for Scarce Sample Polysome profiling (SSP-profiling) – an established method to study active translation [Citation33]. In order to analyse the polysomal association of BC1, we performed qPCR of non-polysomal (NP) and polysomal fractions (P) of GV and MII oocytes. SSP-profiling was validated by qRT-PCR for association 18S and 28S rRNAs with each of profiling fraction (Figure S2) [Citation33]. To our surprise we detected a significant enrichment of BC1 ncRNA in the polysomal fraction of GV oocytes, meaning this RNA is bound to ribosomes despite not coding for a protein (). In MII oocytes BC1 demonstrated equal and very low polysomal association (). Polysomal occupancy of Gapdh mRNA was high in both oocyte stages () and nucleus abundant lncRNA Neat2 [Citation31] was absent in polysomal fractions ().
In conclusion, we found that BC1 is highly abundant in the cytoplasm of the GV oocyte with enrichment at polysomes indicating its involvement in the regulation of maternal RNAs metabolism.
BC1 overexpression leads to decrease of global translation and TOP RNA reporter in GV oocytes
We found BC1 ncRNA was localized in cytoplasm and associated with polysomes in the GV oocyte (, and Figure S1) indicating its possible involvement in translational control in the oocyte. As there was no effect of BC1 knock-down on female fertility [Citation34] we applied an overexpression (OE) approach using microinjection of BC1 RNA into the oocyte cytoplasm (Figure S3). Additionally, to detect global translation rate we performed 35S-Methionine labelling in both GV and MII stages. We found only a mild, but statistically significant, decrease of global translation in the GV stage with BC1 OE (). Nevertheless, there was no influence on total translation in the MII stage despite overexpressed BC1 transcript accumulation compared with the GV stage (Figure S3). Moreover, we analysed whether BC1 OE impedes translation by stress-activated phosphorylation of eIF2α [Citation35]. Using immunoblot we did not detect an increased level of phosphorylated eIF2α (Ser51) (Figure S4), therefore we conclude that BC1 OE does not cause stress-induced translation arrest.
Figure 2. BC1 overexpression leads to decrease of global translation and TOP RNA reporter in the GV oocytes
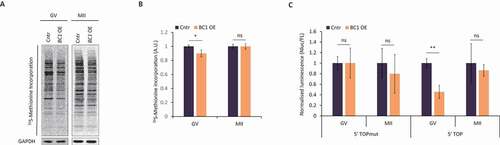
The diminutive decrease of global translation in the GV oocyte might be explained by BC1-mediated translational repression of just a subset of mRNAs. It was reported that BC1 binds PABP and eIF4 in dendrites leading to the repression of cap-dependent translation and therefore 5ʹ TOP mRNAs [Citation8,Citation32] could be the target of BC1-mediated regulation. In order to verify this phenomenon in oocytes, we applied luciferase reporters with 5ʹ TOP motif in the presence of BC1 [Citation36]. We microinjected two RNAs derived from NanoLuc reporter constructs to fully grown GV oocytes with BC1 OE. The first construct contained a mutated 5´TOP motif in 5ʹ UTR while the second one bore a strong canonical 5´TOP sequence. Subsequently, we measured the luminescence signal in both oocyte developmental stages. Obtained results demonstrated no influence of BC1 OE on the control 5ʹTOPmut reporter translation (). Contrarily, we detected a decrease of NanoLuc activity of the canonical 5ʹTOP reporter RNA in GV-stage oocyte, while a lack of influence of BC1 OE was observed in the MII stage ().
Taken together, obtained data might indicate that BC1 is able to influence the translation of a specific subset of mRNAs in the GV stage oocyte as demonstrated by the 5´TOP reporter construct.
BC1 ncRNA influences translation of specific targets in the GV mouse oocyte
Several studies associated BC1 ncRNA function with mRNAs coding for ACTIN B (ACTB) and DLG4 (PSD95) [Citation12,Citation19]. We tested whether BC1 OE influences the production of these proteins. Immunoblot analysis clearly showed that BC1 OE significantly decreased levels of ACTB and DLG4 proteins in the GV stage oocyte while had no effect in the MII stage (). This finding is consistent with the observed, GV-stage oocyte restricted decrease of 5ʹTOP NanoLuc reporter translation after BC1 OE (). Thus, we aimed to study the translation of endogenous 5´TOP mRNA coding for Survivin (SVV) protein [Citation37]. Similarly, the level of SVV protein declined exclusively in BC1 OE GV oocytes (); with no significant change in both control GV-stage oocytes, nor in the MII stage ().
Figure 3. BC1 ncRNA influences translation of specific targets in the GV mouse oocyte
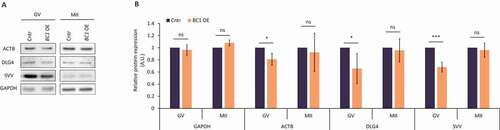
Our data clearly show that BC1 ncRNA negatively influences the translation of specific mRNAs only in the GV stage oocyte in agreement with the analysis of global translation and NanoLuc reporter ().
BC1 physically interacts with candidate mRNAs via protein interplay
In connection with the discovered translational repression of candidate mRNAs coding for ACTB, DLG4 and SVV, we examined potential molecular interactions of selected mRNAs () and BC1 in the mouse oocyte. Due to the fact that the 3ʹUTRs of mRNAs are considered to play major regulatory roles in mRNA fate [Citation38], we focused preferentially on the BC1 interactions with the 3ʹUTR of the candidate mRNAs. We performed in silico prediction of RNA–RNA interaction using open-source IntaRNA 2.0 software [Citation39]. We found that BC1 and 3ʹUTRs of Actb, Dlg4 and Svv mRNAs could form heteroduplexes with BC1 (displayed as different negative values of minimum free energy (MFE), which consist of hybridization energy subtracted by the energy needed to unfold BC1 and target mRNA; Figure S5A). Contrarily, Gapdh mRNA, which is not influenced by BC1 OE, does not contain any region able to interact with BC1 in its 3ʹUTR (Figure S5). Additionally, as a reference Anln mRNA was analysed. Anln mRNA, which interacts with lncRNA Uca1 [Citation40], displayed a high negative MFE (Figure S5A). Actb and Dlg4 mRNAs also exhibited relatively high negative MFE values (high binding affinity). In contrast to Gapdh 3ʹUTR, they both contain U-rich stretches, which were predicted to interact with the 22-nucleotide-long stretch of adenosines in BC1 ncRNA (Figure S5B). Analysis of binding efficiency of TOP mRNA SVV shows low MFE (Figure S5).
In silico analysis of the 3ʹUTR of candidate transcripts revealed their interaction with BC1 ncRNA. Next, we tested whether BC1 – target mRNA interactions are based only on direct RNA-RNA base pairing or are mediated/facilitated by some auxiliary protein/s. To answer this question, we performed RNA pull-down with BC1 anti-sense biotinylated oligonucleotide probes and streptavidin-coupled beads () [Citation41]. In relation to the known function of BC1 in the GV oocyte () we focused on this stage. Firstly, we pulled down BC1 with associated molecular partners from whole oocyte lysates. Following qRT-PCR analysis, we found significant enrichment of BC1 () indicating its efficient capture. Almost equal amounts of Gapdh mRNA were detected in samples without (Cntr) and those containing three (BC1) oligonucleotide probes excluding non-specific pull-down (). Importantly, Actb and Dlg4 candidate mRNAs were considerably enriched after BC1 pull-down (), however, without statistical significance in the case of Svv mRNA (). Additionally, we performed BC1 ncRNA pull-down from purified total RNA isolated from GV oocytes to test whether BC1 binds target mRNA through a protein intermediate partner. Similarly as in the qPCR analysis showed high enrichment of the input BC1 ncRNA () but the absence of the non-target Gapdh mRNA (). No significant enrichment was detected of target Actb, Dlg4 and Svv mRNAs in BC1 pull-down ().
Figure 4. BC1 physically interacts with candidate mRNAs via protein interplay
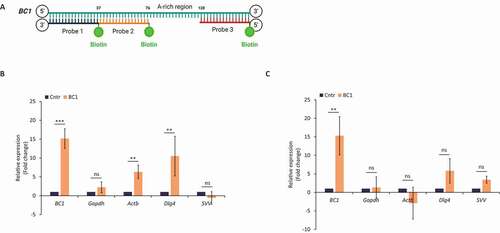
Comparison of BC1 pull-down results obtained from cell lysate and total RNA indicates that an unspecified protein partner/s mediates or at least facilitate (in contribution with the proposed base pairing) BC1 interaction with Actb and Dlg4 mRNAs in the mouse GV oocyte.
BC1 interacts with FMRP protein in the GV oocyte
As fragile X mental retardation protein (FMRP [Citation9,Citation15,Citation16,Citation19]; has previously been identified as partnering BC1 we were prompted to determine its role in the oocyte. Similarly to FMRP, BC1 ncRNA has been described as a translational repressor, but its exact mechanism of action has not yet been detailed [Citation14,Citation42]. Firstly, we analysed FMRP expression in the mouse oocyte and early embryo (Figure S6). FMRP was present in all stages of oocyte maturation with a significant decrease in the 2-cell embryo (Figure S6). To investigate physical BC1 interaction with FMRP we performed in situ RNA–proximity ligation assay (RNA-PLA) in the mouse oocyte [Citation43,Citation44] with the addition of a hybridization step to target RNA followed by proximity detection by anti-biotin antibody and specific antibodies for protein of interest. Using confocal microscopy we observed multiple interaction sites of BC1 ncRNA with FMRP in the GV-oocyte cytoplasm (Figure 5AB) with a significant reduction of their number in the MII stage (). As a positive control for PLA, we used known interaction of eEF2 mRNA with RPL24 in polysomes [Citation43] (Figure S7) and as a negative technical control used LMN A/C phosphorylated at Ser22 (does not interact with eEF2 mRNA) (Figure S7).
Figure 5. BC1 interacts with FMRP protein in the GV oocyte
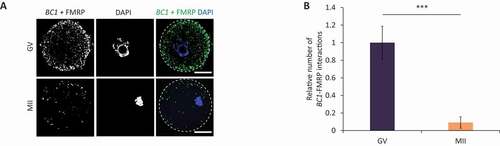
Here, we confirmed that BC1 ncRNA physically interacts with its protein partner FMRP in the GV oocyte.
BC1 overexpression does not lead to clustering of FMRP and RNA in GV oocyte
It is known that inhibition of mRNA translation is associated with the formation of cytoplasmic RNP [Citation45]. FMRP was identified to promote and regulate the assembly of RNP granules including RNP transport granules, P-bodies and stress granules in various cell types [Citation46,Citation47]. Accordingly, we expected that BC1 OE will also induce RNP clusters in the mouse oocyte. Thus, we performed immunocytochemistry (ICC) and RNA FISH in order to examine the localization of FMRP () and poly(A) mRNA () in oocytes with BC1 overexpression. BC1 OE did not induce the formation of either FMRP-containing or Poly(A)-containing RNP granules in the GV stage (). In contrast, MII-stage oocytes contained both FMRP and poly(A) in distinct cytoplasmic clusters (foci) regardless of BC1 overexpression (). Localization of the BC1-FMRP complex positively correlates with detected BC1 ncRNA localization in the cytoplasm ( and Figure S1). Quantification of FMRP-containing and poly(A)-containing foci in MII oocytes showed a significant increase in their number in the BC1 OE group suggesting a positive effect of BC1 on their formation (). Additionally, we performed RNA FISH of Actb mRNA and, intriguingly, we did not observe any clustering pattern in either oocyte group (Figure S8A). Moreover, quantification of the Actb mRNA signal showed a significant increase of the mRNA in the MII stage (Figure S8B); which was independently confirmed by qRT-PCR (Figure S8C).
Figure 6. BC1 overexpression does not lead to clustering of FMRP and RNA in GV oocyte
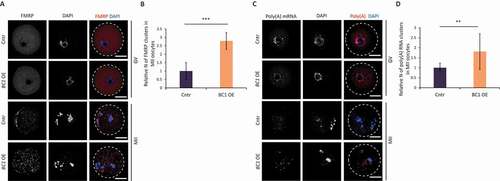
Further, we wanted to test whether the FMRP foci seen in GV oocytes can be related to stress granules (SGs), in other words, whether FMRP is a constituent of SG in GV-stage oocytes ( and Figure S8A). We induced translational stress [Citation48] by 0.5 mM sodium arsenite (arsenite; NaAsO2) treatment. Arsenite is a well-established SGs elicitor, which induces eIF2α phosphorylation at Serine51 and causes translation arrest and polysome disassembly [Citation49] (Figure S9A). Although arsenite treatment of GV oocytes revealed no formation of FMRP clusters, we did however observe a distinct pattern of FMRP localization in perinuclear area (Figure S9B). MII oocytes showed similar FMRP localization clustering pattern in both arsenite-treated and untreated groups (Figure S9B and S9C).
Altogether our results suggest that BC1 OE inhibits the translation of specific mRNAs in the GV stage by mechanisms that do not depend on the formation of protein or RNA foci.
Overexpression of BC1 does not alter oocyte or early embryo development
We observed that BC1 OE leads to the mild downregulation of the translation of specific mRNAs only in the GV oocyte ( and ). Thus, we asked whether BC1 OE might cause any developmental defects during mouse oocyte maturation. We did not find any effect on the oocyte physiology nor on meiotic progression after BC1 OE (). Moreover, we performed parthenogenic activation of MII oocytes matured in vitro from GV oocytes with BC1 OE to analyse developmental oocyte competence. Similarly to oocyte maturation, no abnormalities in the embryo development were observed ().
Figure 7. Overexpression of BC1 does not alter oocyte or early embryo development
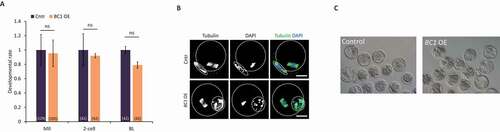
To sum up, we saw that the BC1 OE alone did not have an influence over mouse oocyte maturation nor early embryo development.
In conclusion, we propose an overall scheme how BC1 might contribute to the translational regulation of specific mRNAs in the GV and MII stages (Graphical abstract).
Discussion
In this study, we analysed the role of BC1, a maternal ncRNA which is involved in mRNA metabolism in the mammalian oocyte. Functional analysis of ncRNAs is challenging and roles have only been assigned to a small fraction of ncRNAs, while it is not clear what fraction of ncRNAs has a measurable effect beyond being transcribed and processed through the RNA metabolism. mRNA translation has been shown to be essential for regulating a number of cellular processes during development [Citation50]. This is especially true in the mammalian oocyte which, after a transcriptionally active growth period, resumes meiosis during a period of transcriptional quiescence with a store of maternally synthesized RNAs. Progression through meiosis and early embryo development is therefore regulated at the level of mRNA stabilization, translation and post-translational modification. The storage of ribosomal components and maternal mRNA is a prerequisite for the meiotic and developmental competence of the oocyte [Citation51].
BC1 ncRNA has been shown as a negative regulator of translation in neuronal synapses [Citation9]. We found that BC1 has high expression in the GV stage oocyte and almost disappears in the MII oocyte and post-fertilization. Here we studied the role of BC1 in oocyte protein synthesis via its overexpression. Importantly, overexpression of BC1 does not cause stress leading to global translation decrease as is the case with arsenite treatment. Cytoplasmic localization of BC1 and association with polysomes [our results and Citation4] lead us to the suggestion that BC1 is involved in translational regulation. Polysomal occupation in the oocyte shows the increased translation of specific mRNAs [Citation33,Citation52]; however, a certain portion of polysomes might be translationally inactive and belong to cytoplasmic ribosomal lattices; a storage form of ribosomes known in oocyte [Citation53,Citation54]. The presence of BC1 in polysomal fraction and BC1 physical interaction with FMRP indicate direct interplay with translational machinery of GV oocyte. Colocalisation of BC1 with FMRP in the cytoplasm is consistent with roles in mRNA translation/stabilization. FMRP had been shown to interact with ribosomal protein L5 in drosophila [Citation55,Citation56] but, on the other hand, it was associated with stress granules and P bodies [Citation57,Citation58]. Moreover, in neuronal cell FMRP is associated with polyribosomes [Citation59–61], stalled ribosomes and messenger ribonucleoproteins (mRNPs) which sediment in the same fractions [Citation62, Citation63] where functions as a translational repressor of subset mRNAs [Citation64]. Ribosome stalling is linked to translational repression mechanism where mRNA is repressed at elongation/termination awaiting translational reactivation upon appropriate signals [Citation60,Citation63]. However, to our knowledge, nothing is known about stalling polysomes in mammalian oocyte but endogenous decay of BC1 in the MII oocyte might lead to the release of stalled polysomes or mRNPs from translational inhibition. BC1 appears to promote the interaction between FMRP and other mRNAs that are known to interact with FMRP, possibly via base-pairing interactions, and thereby regulate the translation of a subset of mRNAs at synapses [Citation15,Citation16]. FMRP, involved in regulating the translation of mRNPs, combines with BC1 in the GV oocyte to form the BC1-FMRP complex. This complex can inhibit the translation of a certain subset of FMRP-targeted mRNAs. BC1-FMRP forms in the GV where BC1 is abundant which leads to a mild negative effect on translation only in fully grown GV oocytes. As an example of BC1 negative influence on translation in the GV oocyte is target Actb mRNA which moreover promotes its stability. Experimental expression of BC1 promoted stabilization of target mRNA in the matured oocyte which might indicate that i) translational repression leads to mRNA stabilization or ii) involvement of BC1 in the stabilization of mRNA during oocyte development. In connection, BC1 has possibly more a potent effect during oocyte growth with suppression of newly transcribed mRNAs to exclude them from translational machinery thus promoting their storage and stability for further cellular development.
Interestingly in the GV oocyte both BC1–FMRP and poly(A) RNA distribute evenly without clustering suggesting no formation of large RNP structures (foci) for translational suppression of candidate mRNAs in the GV oocyte. Despite translational stress by arsenite treatment this similarly does not promote the formation of FMRP foci but FMRP instead becomes localized to the perinuclear area where the ER is localized [Citation65–68] suggesting that the clustering or formation of granular structures in the GV oocyte is in some extent inhibited. Flemr et al. [Citation69] similarly show no formation of granular structures related to P-bodies in the fully grown mouse oocyte. P-bodies are distinctive foci in the cytoplasm of eukaryotic cells which have a functional role in mRNA decay and miRNA-mediated translational repression [Citation70–72]. Related to the non-functional miRNA pathway in the mouse oocyte [Citation73,Citation74] might suggest that BC1 could take a role as a translational repressor in the fully grown oocyte however substituted by another mechanism after the resumption of meiosis. Possibly BC1 ncRNA might substitute the miRNA pathway in transitional repression in the growing and fully grown oocyte where the target mRNAs are suppressed therefore promoting their translational dormancy and stability to form ribosomal lattices [Citation53,Citation54,Citation75]. Our results suggest the role of BC1-FMRP complex in the mechanism of promoting translational dormancy and stability of specific maternal mRNAs at cytoplasmic polysomal-mRNP structures in the GV oocyte.
In silico analysis of BC1 binding motifs showed to some extent an affinity to the target mRNAs containing a poly(U) stretch on 3ʹUTR. Direct mRNA-ncRNA binding in the 3ʹUTR region may disrupt PABP attachment and hence the initiation of translation at a single mRNA level. We found that binding efficiency is debilitated with the absence of protein/organelles-ribosomes, mRNPs suggesting a BC1-protein interplay in the translational inhibition. Additionally, our dual-luciferase reporter assay and candidate mRNA demonstrated that translational suppression of BC1 influences RNA belonging to the TOP motif class [Citation37].
As BC1 naturally disappears from the maturing oocyte and its experimental overexpression does not influence translatome globally or specifically (candidate mRNAs) we speculated on an increased formation of FMRP and RNA foci. However, we discovered that FMRP clusters form naturally in the MII oocyte when BC1 becomes naturally decreased. Despite ectopic overexpression BC1 in the mature oocyte increases the clustering of FMRP and RNA without any effect on translation of the candidate mRNA targets. We, therefore, propose that FMRP and polyA RNA clustering is independent of a BC1 role in translational repression in this stage. We suggest that FMRP and PolyA clustering in the MII oocyte lead to the attenuation of an ectopically overexpressed translational repressor that promotes translation.
Apart from a mild effect on the translation of candidate mRNAs, there were no abnormalities in meiotic progression and early embryo development. In summary, BC1 ncRNA is not necessary for terminal mouse oocyte development. Observed resultlinked with BC1 interaction with the 3ʹUTR of newly transcribed mRNAs during oocyte growth suggesting suppression their translation directly on the ribosome-mRNP structures to promote maternal mRNA storage and stability. Further research is needed to investigate the involvement of ncRNAs in the regulation of translation in the mammalian oocyte and early embryo.
Methods
Oocyte isolation, cultivation and treatment
Oocytes were acquired from ICR mice of a minimum of 6 weeks old. The females were stimulated 46 h prior to oocyte isolation using 5 IU of pregnant mare serum gonadotropin (PMSG; Folligon; Merck Animal Health) per mouse. All animal experiments were performed in accordance to guidelines and protocols approved by the Laboratory of Biochemistry and Molecular Biology of Germ Cells at the Institute of Animal Physiology and Genetics in Czech Republic. All animal work was conducted according to Act No. 246/1992 on the protection of animals against cruelty, issued by experimental project #215/2011, certificate #CZ02389, issued by the Ministry of Agriculture. Fully grown GV oocytes were isolated into transfer medium (TM) supplemented with 100 µM 3-isobutyl-1-methylxanthine (IBMX; Sigma Aldrich) for prevention of spontaneous meiotic resumption. Selected oocytes were denuded and cultivated in M16 medium (Millipore) without IBMX at 37°C, 5% CO2 for 0 (GV) or 12 h (MII). For induction of SGs oocytes were treated with 0.5 mM sodium arsenite in M16 medium (arsenite; NaAsO2). Parthenogenetic activation was carried out as previously described [Citation76]. In brief, in vitro matured MII-stage oocytes were cultured in calcium-free CZB medium with 10 mM strontium and supplemented with 5 μg/ml cytochalasin B for 5 hours. Embryos were then cultured in KSOM-AA medium at 37°C, 5%CO2 and 5%O2.
Nuclei isolation
The zona pellucida was removed using Tyrode acid solution (Sigma). The oocytes were disrupted by hand using a pulled glass pipette in PBS drop (cytoplasm) and nuclei were washed in three drops of PBS. Pipette wash (W) from oocyte disruption was used as a control of the isolation protocol.
PCR and RT-PCR
RNeasy Plus Micro kit (Qiagen) was used for RNA extraction following the manufacturer’s instructions. Reverse transcription was performed with qPCRBIO cDNA Synthesis Kit (PCR Biosystems). PCR was performed with PPP master mix (TOP-Bio). The following program was used: 94°C 1 min; 94°C 18 sec; 58°C 15 sec; 72°C for Gapdh; 94°C 1 min; 94°C 18 sec; 60°C 15 sec; 72°C for Neat2 and BC1. Products were separated on 1.5% agarose gel with GelRed (41,003, Biotinum) staining. RT-PCR was then carried out using the QuantStudio13 and the Luna® Universal qPCR Master Mix (New England BioLabs) according to manufacturer’s protocols with an annealing temperature of 60°C. Primers are listed in Table S2A.
Polysome fractionation
Polysome fractionation followed by RNA isolation was carried out according to Scarce Sample Polysome profiling (SSP-profiling) method by Masek et al. [Citation33]. Briefly, cycloheximide-treated oocytes (CHX, Sigma Aldrich) were lysed and resulting samples were loaded onto 10–50% sucrose gradients. Centrifugation was performed in SW55Ti rotor (Beckman Coulter) at 45,000 RPM (246,078 x g) for 65 min at 4°C (Optima L-90 ultracentrifuge, Beckman Coulter). Ten equal fractions were collected from each polysome profile and subjected to RNA isolation by Trizol reagent (Sigma-Aldrich). qRT-PCR-based quantification of 18S and 28S rRNAs in each fraction was applied to visualize individual polysome profiles [Citation33]. Then, non-polysomal (NP; fractions 1–5) and polysomal fractions (P; fractions 6–10) were pooled and subjected to qRT-PCR (QuantStudio 3 cycler, Applied Biosystems) using BC1, Neat2 and Gapdh gene-specific primers. Sequencing libraries were prepared using SMART-seq v4 ultra-low input RNA kit (Takara Bio). Sequencing was performed with HiSeq 2500 (Illumina) as 150-bp paired-ends. Reads were trimmed using Trim Galore v0.4.1 and mapped onto the mouse GRCm38 genome assembly using Hisat2 v2.0.5. Gene expression was quantified as fragments per kilobase per million (FPKM) values in Seqmonk v1.40.0.
Measurement of overall protein synthesis
To measure the overall protein synthesis, 50 mCi of 35S-methionine (Perkin Elmer) was added to methionine-free culture medium. An exact number of oocytes per sample (5–10) were labelled for 12 h, then lysed in SDS-buffer and subjected to SDS–polyacrylamide gel electrophoresis (PAGE). The labelled proteins were visualized by autoradiography on BasReader (Fuji) and quantified by Aida software (RayTest). Gapdh was used as a loading control.
In silico prediction
RNA–RNA interactions were predicted by using the IntaRNA tool with default settings (http://rna.informatik.uni-freiburg.de/IntaRNA/Input.jsp) [Citation39]. Accession numbers of analysed mRNA targets and 3ʹUTR coordinates are listed in Table S1. Visualization of interactions was created with BioRender.com.
Cell lysate and total RNA-lncRNA pull-down
RNA-lncRNA pull-down procedures were done according to Torres et al. [Citation41] with slight changes. Cell lysate pull-down included a cross-linking step: fixation of the cells in 1% PFA and quenching by 1/10 volume of glycine 1.25 M. After 2 × 5 min washes in PBS cells were frozen and stored at −80. In the case of total RNA pull-down cells were frozen without a cross-linking step with subsequent RNA isolation by Trizol reagent (Sigma-Aldrich). Using described lysis (cell lysate) and hybridization buffers, samples were lysed and subsequently incubated on RT for 6 hours with following BC1 anti-sense DNA 5ʹ-biotinylated oligonucleotide probes (GENERI BIOTECH) designed to bind three distinct regions of the BC1 (): probe 1 GGCAAGCGCTCTACCACTGAGCTAAATCCCCAACCCC; probe 2 CCAGAGCTGAGGACCGAACCCAGGGCCTTGCGCTTGCTA; probe 3 TCTTTGAAAATGAAAAAGGTTGTGTGTGCCAGTTACCTTG. Samples were incubated overnight with Dynabeads M-280 Streptavidin (Themofisher). The RNA-protein complex was washed and separated from the beads by Proteinase K. RNA was isolated by Trizol reagent with subsequent analysis by RT-PCR with specific primers.
RNA FISH
RNA FISH was performed according to Tetkova et al. [Citation31]. Briefly, fixed oocytes (15 min in 4% PFA) were pre-treated with protease III provided in RNAScope H2O2 and Protease Reagents kit (diluted 1:15 in nuclease-free water; Cat. No. 322,381, ACD) for 10 min. Each sample was then incubated with RNAScope probe (Table S2C) at 2 h in 40°C to detect Actb mRNA. RNA FISH protocol for amplification was followed using reagents in RNAScope Multiplex Fluorescent Detection Reagents v2 kit (Cat. No. 323,110, ACD), with extended washing: 2 × 10 min washing after probe hybridization (1x wash buffer diluted in nuclease-free water; RNAScope Wash Buffer Reagents, Cat. No. 310,091, ACD); v2 Amp1 30 min, 40°C, 2 × 5 min 1x wash buffer; v2 Amp2 30 min, 40°C, 2 × 5 min 1x wash buffer; v2 Amp3 15 min, 40°C, 2 × 5 min 1x wash buffer. After amplification, HRP-C1/C2/C3 was used on the corresponding channels of specific probe, for 15 min, 40°C. Oocytes were washed again 2 × 5 min in 1x wash buffer. TSA Cy5 dye (Perkin Elmer) diluted to 1:1500 in TSA buffer (ACD) was used for fluorescent labelling of the amplified signal. After washing and application of HRP blocker (30 min in 40°C), samples were washed a final time 2 × 5 min in 1x wash buffer and mounted in Prolong Gold Antifade with DAPI (Life Technologies) on epoxy coated slides (Thermo Scientific). PolyA RNA FISH was performed according to Citation43. Briefly, oocytes were fixed for 10 min in 4% paraformaldehyde and permeabilized in 0.1% Triton X-100 in PBS with 40 units/20 μL of RNAseOut (Invitrogen). Oocytes were washed in Wash Buffer A (Biosearch Technologies) and incubated overnight at 30°C in hybridization buffer (Biosearch Technologies) with 75 nM oligo-dT(22) labelled with Cy5 probe (Generi Biotech). Oocytes were then washed 3x in Wash Buffer A and 2x in SSC (Biosearch Technologies). Images were obtained using a confocal microscope (Leica SP5). Image quantification was performed using ImageJ software (http://rsbweb. nih.gov/ij/). Bacterial DapB RNA (Bacillus subtilis, str. SMY; EF191515.1) was used as a negative control.
RNA–protein in situ proximity ligation assay (RNA-PLA)
Oocytes were cultivated then fixed for 15 min in 4% PFA and pre-treated with protease III provided in RNAScope H2O2, washed in PBS and incubated with BC1 (mentioned above) or eEF2 (GGACTTAACACTTGAACTGCCGTGGGAGTG) anti-sense DNA 5ʹ-biotinylated oligonucleotide probes 2 h in 40°C. After washes in PBS (2 × 5 min), cells were then incubated with mouse anti-biotin antibody (1:150; 03–3700; Invitrogen) together with corresponding primary antibodies: rabbit anti-FMRP (1:150; cs4317; Cell signalling) or anti-RPL24 (1:150; PAS-62,450, Thermo Fisher) at 4°C overnight (primary antibodies are listed in Table S2B). For negative control mouse, anti-biotin antibody and anti-pLMN A/C (1:150; cs2026; Cell signalling) antibody were used. PLA was performed following instructions of PLA Duolink kits (DUO92006 and DUO92008, Sigma Aldrich) and a previously published protocol [Citation43]. The samples were washed using PBS and then in Wash Buffer A (Sigma Aldrich) and incubated with 40 μL of the probe reaction mix for 1 h at 37°C. Next, the samples were washed in 1x Wash Buffer A for 2 × 5 min and the following ligation reaction was performed for 30 min at 37°C. After washing (2 × 5 min) in Wash Buffer A, 40 μL of amplification reaction reagent was added to each sample and incubated for 100 min at 37°C. The samples were then washed for 2 × 10 min in 1x Wash Buffer B (Sigma Aldrich) and for 2 min in 0.01x Wash Buffer B and mounted on a slide using Prolong Mounting Medium with DAPI (H-1500, Vector Laboratories). Inverted confocal microscope (Leica SP5) was used for sample visualization. Image quantification of interactions was analysed in ImageJ software (http://rsbweb.nih.gov/ij/).
Immunocytochemistry
Fixed oocytes (15 min in 4% PFA; Sigma Aldrich) were permeabilized in 0.1% Triton X-100 for 10 min, washed in PBS supplemented with polyvinyl alcohol (PVA, Sigma Aldrich) and incubated with primary antibodies (Table S2B) diluted in PVA/PBS overnight at 4°C. Oocytes were then washed 2 × 15 min in PVA/PBS and primary antibodies were detected using relevant Alexa Fluor 488/594/647 conjugates (Invitrogen) diluted to 1:250 for 1 h at room temperature. Washed oocytes (2 × 15 min in PVA/PBS) were mounted onto slides using ProLong Mounting Medium with DAPI. Inverted confocal microscope (Leica SP5) was used for sample visualization. Image quantification and assembly were performed using ImageJ and Adobe Photoshop CS3. Experiments were repeated 3x with 20–30 oocytes per group/experiment.
Microinjection of oocytes and live-cell imaging
Isolated NE oocytes were microinjected in TM with IBMX using inverted microscope Leica DMI 6000B, TransferMan NK2 (Eppendorf) and FemtoJet (Eppendorf). Solution used for oocyte injection contained: 20 ng/µL of in vitro transcribed H2b:gfp RNA (mMessage, Ambion) from plasmid (provided by Dr. Martin Anger, Laboratory of Cell Division Control, IAPG CAS) in combination with 20 ng/µL of in vitro transcribed BC1 RNA (mMessage, Ambion) from Puc57 plasmid (Generi biotech). 1 h after microinjection oocytes were selected and either washed from IBMX and cultivated to MII stage or stored at GV stage overnight. Microinjected oocytes were placed into 4-well culture chamber (Sarstedt) in 10 µL of equilibrated M16 media (37.5°C, 5% CO2) covered with mineral oil (M8410; Sigma Aldrich). The cells were imaged using inverted microscope Leica DMI 6000B equipped with a controlled chamber system (Tempcontroller 2000–2 Pecon, and a CO2 controller, Pecon). Time-lapse movies (LAS X, Leica microsystems) of meiotic maturation of microinjected oocytes with chromatin marker (H2b:gfp) were used for phenotype evaluation (nuclear envelope breakdown, polar body extrusion).
Luciferase reporter assay
GV oocytes were microinjected with Nanoluc and Firefly reporter constructs using micromanipulator Leica DMI 6000B, TransferMan NK2 (Eppendorf) and FemtoJet (Eppendorf). 5ʹTOP and 5ʹTOPmut sequence were cloned into pNL1.2 vector (Promega). NheI-T7 over hanged forward primer and BglII reverse primers used to amplify 5ʹTOP and 5ʹTOPmut sequence from plasmids which contained conventional and mutated 5ʹUTR TOP sequence of Eef2 mRNA: pIS1-Eef25UTR-renilla (Addgene #38,235), pIS1-Eef25UTR-TOPmut-renilla (Addgene #38,236) [Citation77] vector and cloned into pNL1.2 vector. Combination with Firefly Luciferase (Addgene #18,964) was used as an injection control. Samples were analysed by Dual-Glo Luciferase Assay System (Promega). Luminescence was measured by Synergy HTX Plate Reader (BioTek Instruments).
Immunoblotting
An exact number of cells (15–30 oocytes) was washed in PVA/PBS and frozen to −80°C. Prepared samples were lysed in NuPAGE LDS Sample Buffer (NP0007, Thermo Fisher Scientific) and NuPAGE Sample Reducing Agent (NP0004, Thermo Fisher Scientific) and heated at 100°C for 5 min. Proteins were separated on precast gradient 4–12% SDS–PAGE gel (Thermo Fisher Scientific) and blotted to Immobilon P membrane (Millipore) in a semidry blotting system (Biometra GmbH) at 5 mA cm2 for 25 min. Membranes were then blocked in 5% skimmed milk dissolved in 0.05% Tween-Tris buffer saline (TTBS), pH 7.4 for 1 h. Membranes were incubated overnight at 4°C with the primary antibodies (Table S2B) diluted in 1% milk/TTBS. Secondary antibodies with Peroxidase were used (711–035-152 Anti-Rabbit Donkey or 715–035-151 Anti-Mouse Donkey, both Jackson Immunoresearch), diluted 1:7500 in 1% milk/TTBS for 1 h at room temperature. ECL (Amersham) was used for visualization of immunodetected proteins on X-ray films. The films were scanned by calibrated densitometer (GS-800, Bio-Rad Laboratories) and quantified in ImageJ. Presented images were cropped from membranes, contrast and brightness were adjusted using Adobe Photoshop CS3.
Ethical approval
All animal work was conducted according to Act No 246/1992 for the protection of animals against cruelty; from 25.09.2014 number CZ02389, issued by the Ministry of Agriculture.
Supplemental Material
Download PDF (2.3 MB)Acknowledgments
We thank Jaroslava Supolikova and Marketa Hancova for assistance with experiments.
Disclosure statement
No potential conflict of interest was reported by the authors.
Supplemental material
Supplemental data for this article can be accessed here.
Additional information
Funding
References
- Kutter C, Watt S, Stefflova K, et al. Rapid turnover of long noncoding RNAs and the evolution of gene expression. PLoS Genet. 2012;8:e1002841.
- Ponting CP, Oliver PL, Reik W. Evolution and functions of long noncoding RNAs. Cell. 2009;136:629–641.
- Frankish A, Diekhans M, Ferreira AM, et al. GENCODE reference annotation for the human and mouse genomes. Nucleic Acids Res. 2019;47:766–773.
- Tiedge H, Fremeau RT, Weinstock PH, et al. Dendritic location of neural BC1 RNA. Proc Natl Acad Sci U S A. 1991;88:2093–2097.
- Taft RJ, Pheasant M, Mattick JS. The relationship between non-protein-coding DNA and eukaryotic complexity. BioEssays. 2007;29:288–299.
- Rozhdestvensky TS, Kopylov AM, Brosius J, et al. Neuronal BC1 RNA structure: evolutionary conversion of a tRNAAla domain into an extended stem-loop structure. Rna. 2001;7:722–730.
- Lin D, Pestova TV, Hellen CUT, et al. Translational control by a small RNA: dendritic BC1 RNA targets the eukaryotic initiation factor 4A helicase mechanism. Mol Cell Biol. 2008;28:3008–3019.
- Eom T, Berardi V, Zhong J, et al. Dual nature of translational control by regulatory BC RNAs. Mol Cell Biol. 2011;31:4538–4549.
- Lee Y, Lee HS, Kim M, et al. Brain cytoplasmic RNAs in neurons: from biosynthesis to function. Biomolecules. 2020;10:11–15.
- Muddashetty RS, Khanam T, Kondrashov A, et al. Poly(A)-binding protein is associated with neuronal BC1 and BC200 ribonucleoprotein particles. J Mol Biol. 2002;321:433–445.
- Wang H, Iacoangeli A, Popp S, et al. Dendritic BC1 RNA: functional role in regulation of translation initiation. J Neurosci. 2002;22:10232–10241.
- Briz V, Restivo L, Pasciuto E, et al. The non-coding RNA BC1 regulates experience-dependent structural plasticity and learning. Nat Commun. 2017;8:1–15.
- Lacoux C, Di Marino D, Boyl PP, et al. BC1-FMRP interaction is modulated by 2′-O-methylation: RNA-binding activity of the tudor domain and translational regulation at synapses. Nucleic Acids Res. 2012;40:4086–4096.
- de Rubeis S, Bagni C. Regulation of molecular pathways in the Fragile X Syndrome: insights into Autism Spectrum Disorders. J Neurodev Disord. 2011;3:257–269.
- Zalfa F, Giorgi M, Primerano B, et al. The Fragile X syndrome protein FMRP associates with BC1 RNA and regulates the translation of specific mRNAs at synapses. Cell. 2003;112:317–327.
- Zalfa F, Adinolfi S, Napoli I, et al. FMRP binds specifically to the brain cytoplasmic RNAs BC1/BC200 via a novel RNA binding motif. J Biol Chem. 2005;280:33403–33410.
- Zhang T, Pang P, Fang Z, et al. Expression of BC1 impairs spatial learning and memory in alzheimer’s disease via APP translation. Mol Neurobiol. 2018;55:6007–6020.
- Eom T, Muslimov IA, Iacoangeli A, et al. Dendritic targeting and regulatory RNA control of local neuronal translation. Oxford Handb Neuronal Protein Synth. 2018;1–29.
- Iacoangeli A, Rozhdestvensky TS, Dolzhanskaya N, et al. On BC1 RNA and the fragile X mental retardation protein. Proc Natl Acad Sci U S A. 2008;105:734–739.
- Zhong J, Chuang SC, Bianchi R, et al. Regulatory BC1 RNA and the fragile X mental retardation protein: convergent functionality in brain. PLoS One. 2010;5:e15509.
- Darnell JC, Van Driesche SJ, Zhang C, et al. FMRP stalls ribosomal translocation on mRNAs linked to synaptic function and autism. Cell. 2011;146:247–261.
- Iacoangeli A, Tiedge H. Translational control at the synapse: role of RNA regulators. Trends Biochem Sci. 2013;38:47–55.
- Battaglia R, Vento ME, Borzì P, et al. Non-coding RNAs in the ovarian follicle. Front Genet. 2017;8:57.
- Ganesh S, Horvat F, Drutovic D, et al. The most abundant maternal lncRNA Sirena1 acts post-transcriptionally and impacts mitochondrial distribution. Nucleic Acids Res. 2020;48:3211–3227.
- Karlic R, Ganesh S, Franke V, et al. Long non-coding RNA exchange during the oocyte-to-embryo transition in mice. DNA Res. 2017;24:129–141.
- Kataruka S, Modrak M, Kinterova V, et al. MicroRNA dilution during oocyte growth disables the microRNA pathway in mammalian oocytes. Nucleic Acids Res. 2020;48:8050–8062.
- De La Fuente R, Viveiros MM, Burns KH, et al. Major chromatin remodeling in the germinal vesicle (GV) of mammalian oocytes is dispensable for global transcriptional silencing but required for centromeric heterochromatin function. Dev Biol. 2004;275:447–458.
- Susor A, Kubelka M. Translational regulation in the mammalian oocyte. In: Results and problems in cell differentiation. Results. 2017;63:257-295. Springer Verlag
- Carrieri C, Cimatti L, Biagioli M, et al. Long non-coding antisense RNA controls Uchl1 translation through an embedded SINEB2 repeat. Nature. 2012;491:454–457.
- Hutchinson JN, Ensminger AW, Clemson CM, et al. A screen for nuclear transcripts identifies two linked noncoding RNAs associated with SC35 splicing domains. BMC Genomics. 2007;8:1–16.
- Tetkova A, Jansova D, Susor A. Spatio-temporal expression of ANK2 promotes cytokinesis in oocytes. Sci Rep. 2019;9:1–13.
- Wang H, Iacoangeli A, Lin D, et al. Dendritic BC1 RNA in translational control mechanisms. J Cell Biol. 2005;171:811–821.
- Masek T, Del Llano E, Gahurova L, et al. Identifying the translatome of mouse NEBD-stage oocytes via SSP-profiling; a novel polysome fractionation method. Int J Mol Sci. 2020;21:1254.
- Skryabin BV, Sukonina V, Jordan U, et al. Neuronal untranslated BC1 RNA: targeted gene elimination in mice. Mol Cell Biol. 2003;23:6435–6441.
- Dever TE. Gene-specific regulation by general translation factors. Cell. 2002;108:545–556.
- England CG, Ehlerding EB, Cai W. NanoLuc: a small luciferase is brightening up the field of bioluminescence. Bioconjug Chem. 2016;27:1175–1187.
- Gandin V, Masvidal L, Hulea L, et al. NanoCAGE reveals 5ʹ UTR features that define specific modes of translation of functionally related MTOR-sensitive mRNAs. Genome Res. 2016;26:636–648.
- Mayr C. What are 3′ UTRs doing? Cold Spring Harb. Perspect Biol. 2019;11(10):a034728.
- Mann M, Wright PR, Backofen R. IntaRNA 2.0: enhanced and customizable prediction of RNA-RNA interactions. Nucleic Acids Res. 2017;45:435–439.
- Barbagallo C, Brex D, Caponnetto A, et al. LncRNA UCA1, upregulated in CRC biopsies and downregulated in serum exosomes, controls mRNA expression by RNA-RNA interactions. Mol Ther Nucleic Acids. 2018;12:229–241.
- Torres M, Becquet D, Guillen S, et al. RNA pull-down procedure to identify RNA targets of a long non-coding RNA. J Vis Exp. 2018;2018:e57379.
- Chen E, Joseph S. Fragile X mental retardation protein: a paradigm for translational control by RNA-binding proteins. Biochimie. 2015;114:147–154.
- Jansova D, Tetkova A, Koncicka M, et al. Localization of RNA and translation in the mammalian oocyte and embryo. PLoS One. 2018;13:1–25.
- Söderberg O, Leuchowius KJ, Gullberg M, et al. Characterizing proteins and their interactions in cells and tissues using the in situ proximity ligation assay. Methods. 2008;45:227–232.
- Erickson SL, Lykke-Andersen J. Cytoplasmic mRNP granules at a glance. J Cell Sci. 2011;124:293–297.
- Lai A, Valdez-Sinon AN, Bassell GJ. Regulation of RNA granules by FMRP and implications for neurological diseases. Traffic. 2020;21:454–462.
- Rosario R, Filis P, Tessyman V, et al. FMRP associates with cytoplasmic granules at the onset of meiosis in the human oocyte. PLoS One. 2016;11:1–14.
- Wheeler JR, Matheny T, Jain S, et al. Distinct stages in stress granule assembly and disassembly. Elife. 2016;5:e18413.
- Pakos‐Zebrucka K, Koryga I, Mnich K, et al. The integrated stress response. EMBO Rep. 2016;17:1374–1395.
- Shi Z, Barna M. Translating the genome in time and space: specialized ribosomes, RNA regulons, and RNA-binding proteins. Annu Rev Cell Dev Biol. 2015;31:31–54.
- Monti M, Zanoni M, Calligaro A, et al. Developmental arrest and mouse antral not-surrounded nucleolus oocytes. Biol Reprod. 2013;88:1–7.
- Del Llano E, Masek T, Gahurova L, et al. Age-related differences in the translational landscape of mammalian oocytes. Aging Cell. 2020;19:e13231.
- Burkholder GD, Comings DE, Okada TA. A storage form of ribosomes in mouse oocytes. Exp Cell Res. 1971;69:361–371.
- Yurttas P, Vitale AM, Fitzhenry RJ, et al. Role for PADI6 and the cytoplasmic lattices in ribosomal storage in oocytes and translational control in the early mouse embryo. Development. 2008;135:2627–2636.
- Chen E, Sharma MR, Shi X, et al. Fragile X mental retardation protein regulates translation by binding directly to the ribosome. Mol Cell. 2014;54:407–417.
- Ishizuka A, Siomi MC, Siomi H. A Drosophila fragile X protein interacts with components of RNAi and ribosomal proteins. Genes Dev. 2002;16:2497–2508.
- Didiot MC, Subramanian M, Flatter E, et al. Cells lacking the fragile X mental retardation protein (FMRP) have normal RISC activity but exhibit altered stress granule assembly. Mol Biol Cell. 2009;20:428–437.
- Lee EK, Kim HH, Kuwano Y, et al. HnRNP C promotes APP translation by competing with FMRP for APP mRNA recruitment to P bodies. Nat Struct Mol Biol. 2010;17:732–739.
- Feng Y, Absher D, Eberhart DE, et al. FMRP associates with polyribosomes as an mRNP, and the I304N mutation of severe fragile X syndrome abolishes this association. Mol Cell. 1997;1:109–118.
- Khandjian EW, Huot ME, Tremblay S, et al. Biochemical evidence for the association of fragile X mental retardation protein with brain polyribosomal ribonucleoparticles. Proc Natl Acad Sci U S A. 2004;101:13357–13362.
- Stefani G, Fraser CE, Darnell JC, et al. Fragile X mental retardation protein is associated with translating polyribosomes in neuronal cells. J Neurosci. 2004;24:7272–7276.
- Davidson EH. Gene Activity in Early Development. 3rd Edition. Academic Press, Inc; 1986. p. 158-159.
- Graber TE, Hébert-Seropian S, Khoutorsky A, et al. Reactivation of stalled polyribosomes in synaptic plasticity. Proc Natl Acad Sci U S A. 2013;110:16205–16210.
- Shah S, Molinaro G, Liu B, et al. FMRP control of ribosome translocation promotes chromatin modifications and alternative splicing of neuronal genes linked to autism. Cell Rep. 2020;30:4459–4472.e6.
- Dalton CM, Carroll J. Biased inheritance of mitochondria during asymmetric cell division in the mouse oocyte. J Cell Sci. 2013;126:2955–2964.
- FitzHarris G, Marangos P, Carroll J. Changes in endoplasmic reticulum structure during mouse oocyte maturation are controlled by the cytoskeleton and cytoplasmic dynein. Dev Biol. 2007;305:133–144.
- Schlaitz AL, Thompson J, Wong CCL, et al. REEP3/4 ensure endoplasmic reticulum clearance from metaphase chromatin and proper nuclear envelope architecture. Dev Cell. 2013;26:315–323.
- Susor A, Jansova D, Cerna R, et al. Temporal and spatial regulation of translation in the mammalian oocyte via the mTOR-eIF4F pathway. Nat Commun. 2015;6:1–12.
- Flemr M, Ma J, Schultz RM, et al. P-body loss is concomitant with formation of a messenger RNA storage domain in mouse oocytes. Biol Reprod. 2010;82:1008–1017.
- Chan SP, Slack FJ. microRNA-mediated silencing inside P-bodies. RNA Biol. 2006;3:97–100.
- Jakymiw A, Pauley KM, Li SL, et al. The role of GW/P-bodies in RNA processing and silencing. J Cell Sci. 2007;120:1702.
- Parker R, Sheth U. P bodies and the control of mRNA translation and degradation. Mol Cell. 2007;25:635–646.
- Ma J, Flemr M, Stein P, et al. MicroRNA activity is suppressed in mouse oocytes. Curr Biol. 2010;20:265–270.
- Suh N, Baehner L, Moltzahn F, et al. MicroRNA function is globally suppressed in mouse oocytes and early embryos. Curr Biol. 2010;20:271–277.
- Sternlicht AL, Schultz RM. Biochemical studies of mammalian oogenesis: kinetics of accumulation of total and poly(A)‐containing RNA during growth of the mouse oocyte. J Exp Zool. 1981;215:191–200.
- Lin CJ, Koh FM, Wong P, et al. Hira-mediated H3.3 incorporation is required for DNA replication and ribosomal RNA transcription in the mouse zygote. Dev Cell. 2014;30:268–279.
- Thoreen CC, Chantranupong L, Keys HR, et al. A unifying model for mTORC1-mediated regulation of mRNA translation. Nature. 2012;485:109–113.