ABSTRACT
B cells constitute a main branch adaptive immune system. They mediate host defence through the production of high-affinity antibodies against an enormous diversity of foreign antigens. Remarkably, B cells undergo multiple types of somatic DNA mutation to achieve this effector function, including class switch recombination (CSR) and somatic hypermutation (SHM). These processes occur in response to antigen recognition and inflammatory signals, and require strict biological control at multiple levels. Transcription within the locus that encodes antibodies plays direct roles in CSR. Additional non-coding RNAs (ncRNAs), including both microRNAs (miRNAs) and long ncRNAs (lncRNAs), also play pivotal roles in B cell activation and terminal effector function through post-transcriptional gene regulation and chromatin remodelling, respectively.
Intent
The purpose of this review is to convey a concise resource outlining recent work in the field of ncRNAs as it pertains to non-malignant B cell biology. Extensive reviews exist for individual aspects of miRNA mediated B cell development and lymphomagenesis [Citation1,Citation2], miRNA Cross-linking and immunoprecipitation (CLIP) experimental design and analysis [Citation3–5], B cell responses [Citation6], and the molecular regulation of class switch recombination (CSR) [Citation7,Citation8]. We refer the reader to these in-depth reviews for further detail on these individual topics, and reference them and recent primary literature to explore these topics in this review.
B cells
B cells form the humoral branch of the adaptive immune system and are key drivers in defending the host through immunological memory and antibody production. The complex life span of B cells requires distinct developmental checkpoints beginning with early development and lineage commitment in the bone marrow, followed by migration to and final establishment of maturity in secondary lymphoid organs like the spleen and peripheral lymph nodes [Citation9]. These mature B cells are poised to respond directly to pathogens through engagement of their antigen B cell receptor (BCR), and to a broad range of pathogenic stimuli through pattern recognition receptors (PRRs) such as the Toll-like receptors (TLRs). Antigen receptor-stimulated responses can occur in a T-independent (TI) fashion, where B cells expand rapidly and generate low-affinity antigen-specific antibodies mostly of the IgM isotype [Citation6]. The responses can also be T-dependent (TD), whereby activated cells enter the microanatomical space of the germinal centre (GC), and iteratively cycle between antigen receptor mutating and proliferating centroblasts in the dark zone (DZ) that then migrate to the light zone (LZ) as centrocytes where they are selected through pro-survival cues from T follicular helper (Tfh) cells [Citation6,Citation10]. Through this process, long-term immunological memory is established, and high-affinity antibodies are produced against a specific antigen. To generate high-affinity antibodies, B cells induce a DNA mutation process known as somatic hypermutation (SHM) in which Igv regions that encode antigen-binding domains of the BCR are selectively mutated by the activation-induced cytidine deaminase (AID) enzyme. B cell clones with BCRs of increased affinity gain a selection advantage [Citation10]. B cells can also undergo another type of somatic DNA mutation, known as class switch recombination (CSR), in which B cells coordinately rearrange genomic regions in the IgH locus to produce antibodies of different isotypes (IgG, IgA, IgE) in response to pathogen and cytokine stimulation. This process occurs outside of the GC in extrafollicular regions [Citation11]. These DNA mutation events are tightly regulated and ncRNAs play a large role in the process of CSR, as discussed later.
MicroRNAs
Initially discovered in C. elegans, miRNAs are small 18–22nt RNAs that destabilize protein coding mRNAs [Citation12]. Canonical miRNAs are transcribed as long pri-miRNA transcripts that require processing by the Drosha/Dgcr8 microprocessor complex in the nucleus to yield short hairpin pre-miRNAs that are exported to the cytoplasm. There, they are further processed by Dicer to form short duplexes, one strand of which is loaded onto Argonaute (Ago) proteins as mature miRNAs [Citation13]. MiRNAs guide the Ago protein-containing miRNA-induced silencing complex (RISC) to the 3ʹUTR of target mRNAs via complementary base pairing [Citation14]. Families of miRNAs with shared target repertoires are defined by their ‘seed sequence’ (nucleotides 2–8 from the 5ʹ end of the mature miRNA), which is the major determinant of target recognition, while 3ʹ ends of the miRNAs may contribute partially to target recognition [Citation14,Citation15]. The miRISC mediates translational inhibition and destabilization of bound mRNAs [Citation16]. Individual miRNAs and their family members can target hundreds of unique transcripts and thereby regulate large gene networks in a manner specific to cellular context and gene expression programme.
The techniques utilized to match miRNAs to their targets include bioinformatic prediction based on sequence complementarity and other features, differential gene expression analyses, and biochemical experiments that capture Ago bound miRNAs and their physically associated target RNAs. A variety of methods to capture and catalogue protein-associated RNAs have been described [Citation4,Citation5,Citation17]. For miRNA and miRISC target identification, Ago2 high-throughput sequencing of RNA isolated by crosslinking immunoprecipitation (HITS-CLIP) [Citation18], photoactivatable ribonucleoside-enhanced CLIP (PAR-CLIP) [Citation19], and individual-nucleotide resolution CLIP (iCLIP) [Citation20,Citation21] have been instrumental. Each technique relies on UV crosslinking of ribonucleoprotein complexes, immunoprecipitation of Ago2 and then downstream RNA sequencing library preparation to elucidate both miRNA and target RNA sequences bound to Ago2.
Biochemical identification of miRNA target sites in immune cells was pioneered with Ago2-HITS-CLIP in T cells [Citation22] and PAR-CLIP in immortalized B cells [Citation23], and more recently extended with iCLIP libraries from B cells, T cells DCs, and macrophages [Citation20]. Ago-CLIP experiments provide a global snapshot of miRNA and target RNA interactions, but the stoichiometry of miRNA and target mRNA expression limit the targets that can be elucidated by these techniques, as highly expressed miRNAs and targets can dominate sequencing libraries [Citation24]. Comparison of CLIP libraries from miRNA sufficient and deficient cells can improve Ago binding site assignment to a specific miRNA of interest. Open-source analysis tools for CLIP and comparative CLIP include dCLIP [Citation25], Piranha [Citation26] and PARma [Citation27], among others [Citation3]. As bioinformatic tools and CLIP technologies progress, global and context-specific miRNA:target maps will become more clear.
MiRNAs in B cell development
MiRNAs are indispensable for early B cell programming from the pro-B to pre-B stage, as ablation of miRNA processing machinery leads to complete block at this developmental stage [Citation1,Citation28,Citation29]. During this stage of development, B cells undergo VDJ recombination, a form of combinatorial DNA mutation to produce a unique antigen receptor in each developing B cell. Dysregulation of miRNA biogenesis at this stage leads to increased apoptosis, and an inability to form functional BCRs. Further studies demonstrated that individual miRNAs are necessary for key developmental checkpoints in the B cell lineage commitment, and their dysregulation can lead to transformation and leukaemia/lymphomagenesis phenotypes [Citation1,Citation2,Citation30].
MiRNA biogenesis is also important for B cell maturation. CD19-cre mediated Dicer ablation leads to dysfunctional B cell selection and the generation of autoimmunity with lupus-like disease and a skewing of the Ig repertoire towards a multitude of self-antigens [Citation31]. A propensity in mature B cells to commit to the marginal zone (MZ) lineage occurs in the absence of miR-142, and this miRNA is key to haematopoietic development of many immune cell lineages [Citation32]. The developmental fate decision for mature B cells to commit to the marginal zone compartment over the follicular compartment is also mediated by control of miR-146a [Citation33], and the B1 lineage is regulated by miR-150 [Citation34].
MiRNAs in B cell activation, GC and PC development
In the context of acute activation, naive B cells undergo profound metabolic restructuring [Citation35,Citation36], poising these cells to rapidly proliferate, and undergo transcriptional changes. The miRNA landscape of activated B cells also drastically changes, as miRNAs are dynamically regulated to modulate the gene expression changes necessary for such rapid cellular responses [Citation37,Citation38]. Deletion of Dicer at the GC/PC commitment stage (utilizing Aicda-Cre) leads to a profound defect in humoral immunity through decreased GC cells and PCs and isotype switched antibodies in response to immunization [Citation39].
Multiple miRNAs regulate the activation of B cells, with miR-155 as a keystone regulator of B cell activation and terminal cell differentiation and transformation (). Originally discovered as B cell integration cluster or (bic), the primary transcript from which miR-155 is processed is one of the most highly upregulated transcripts upon B cell activation [Citation40]. This miRNA has been shown to directly regulate GC and PC differentiation [Citation41–43], including promoting cell cycle [Citation44], regulating apoptosis [Citation45], and directly targeting AID and DNA mutation associated with CSR [Citation46,Citation47]. Notably, several of these studies showed that mutating binding sites in individual target mRNAs conferred partial or intermediate phenotypes related to those seen in miR-155 deficient B cells. Mutations in miR-155 seed match sites in the mRNAs that encode AID [Citation46,Citation47], PU.1 [Citation48], and SOCS1 [Citation49] each partially phenocopied miR-155 deficiency. These studies demonstrate that single miRNA:target interactions have measurable biological effects in a cell and context-dependent fashion. Taken together, they also demonstrate the promiscuity with which a single miRNA can target multiple genes simultaneously, and provide direct evidence that miRNAs operate through the regulation of networks of consequential gene targets.
Recent studies have demonstrated a novel interplay between miRNA regulation of metabolism [Citation50], and metabolite regulation of miRNA expression [Citation51]. MiRNAs of the let-7 family repress T-independent B cell responses through metabolic regulation [Citation50]. These miRNAs directly target Hexokinase-2 (Hk2) and the glutamine transporter Slc1a5 to limit glycolysis and glutamine uptake, ultimately repressing c-Myc protein expression in response to both antigen and lipopolysaccharide stimulation of B cells. Mice with a B cell-specific deficiency in the let-7adf miRNA cluster show increased antibody responses to T-independent immunizations, linking this metabolic control to the T-independent PC fate decision. Further evidence has directly linked metabolites as regulators of miRNA expression in B cells [Citation51]. Epigenetic changes elicited by the short-chain fatty acid metabolites butyrate and propionate in B cells upregulate specific miRNAs, which in turn modulate Aicda and Prdm1 and affect PC fate decisions and autoantibody production. B cell activation induces rapid metabolic changes [Citation36] and GC B cells selectively utilize fatty acid oxidation over glycolysis [Citation52]. The dynamic inter-regulation of miRNAs and metabolism in B cells in the context of cell fate decisions requires further study.
The miR-17 ~ 92 miRNA cluster plays a key role in regulating PC development, migration to the bone marrow and isotype-specific antibody production [Citation53]. Overexpression of miR-17 ~ 92 causes lymphoproliferation [Citation54] and lymphomagenesis [Citation55]. Mice with germline deficiency in this miRNA cluster have a profound pro-B to pre-B development block [Citation29,Citation56]. However, utilizing CD19-cre to ablate this cluster later in development leads to dysregulated B cell selection, central tolerance and autoimmunity [Citation57]. Deletion at this stage also leads to defects in IgG2c antibody titres and enhanced PC migration to the bone marrow, related to targeting of Ikaros and S1pr1, respectively [Citation53]. Further studies also implicated this miRNA cluster in graft vs host disease in both T and B cell-intrinsic fashions [Citation58].
Many of the biological effects of these miRNAs are mediated at least in part by tuning antigen and cytokine receptor signalling. MicroRNAs of the miR-17 ~ 92 cluster repress several mRNAs that encode negative regulators of intracellular signalling including Pten, Socs1, Tnfaip2, Phlpp2 [Citation57,Citation59,Citation60], as well as Cd22 and Fcgr2b, which encode cell surface proteins whose signalling inhibit B cell activation [Citation61]. miR-29 also targets Pten, and ablating one copy of this target gene ameliorated signalling, survival and differentiation defects observed in miR-29-deficient B cells [Citation62]. In addition to Socs1, miR-155 also targets Inpp5d, which encodes the phosphatase SHIP1 [Citation63].
Intercellular miRNA transfer was recently implicated as positively regulating the GC B cell response [Citation64]. Rab27, which is required for the production of extracellular vesicles (EV), was required in T cells for optimal GC B cell responses in vivo. T cell-derived EVs containing miR-20a, miR-25, and miR-155 can be transferred to GC B cells through the immune synapse formed between activated GC B cells and Tfh cells in the LZ of the GC. Activated T cells release EVs with diverse cargos, including other miRNAs and other ncRNAs [Citation65,Citation66]. Further study is needed to probe whether transfer of other cargos also influences cellular interactions and GC dynamics.
ncRNAs in CSR
miRNAs
The process of CSR, whereby B cells rearrange the immunoglobulin heavy chain locus (Igh), confers isotype-specific biological activity to the BCR and corresponding antibodies. This process requires active transcription in the Igh locus and the deaminase AID [Citation7,Citation8]. Several miRNAs directly regulate AID, including miR-181b in mouse [Citation67], miR-29b in human [Citation68], and miR-155 in both species [Citation43,Citation46,Citation47] (). Mouse miR-29 also limits class switch recombination in mouse, though this appears to be related to regulation of cell signalling rather than direct repression of AID [Citation62]. Other miRNAs that alter B cell signalling and transcriptional programmes also affect CSR indirectly in this manner.
Germline Igh transcripts
Beyond miRNAs that directly regulate cellular functions necessary for CSR, ncRNA from active germline transcription (GLT) at switch (S) regions in the Igh locus have been shown to form stable RNA:DNA hybrids known as R loops and further DNA and RNA G quadraplexes (G4) [Citation7,Citation8,Citation69,Citation70]. R loops have been implicated as a mode of structural conformation stability that allows targeting of AID to single-stranded DNA exposed during transcription [Citation8]. It had been postulated that R loops were necessary for AID recruitment, as inversion of the Sγ1 region in mice, which profoundly reduced R loop formation, concordantly reduced CSR to IgG1 [Citation71]. However, a recent study demonstrated that R loops are not required for AID-mediated mutagenesis, as a transgenic mouse where the Ig variable (Igv) region was replaced with a core S region in either physiological or reverse orientation showed equivalent AID mutation rates in both cases [Citation72]. These two studies together indicate that the frequency of R loop formation in Igh is correlated with CSR, but not necessarily with AID recruitment, hinting that R loops could be a transient structural phenomenon. These studies were complemented by the demonstration that RNAse H overexpression in B cells, leading to the rapid digestion of R loops, showed an increase in AID-induced mutation in the Igh locus and minimally decreased the frequency of CSR [Citation73]. It is possible that collapse of R-loops allows access of AID to both strands of DNA, as postulated previously [Citation74]. Furthermore, the RNA exosome, an RNA processing and degradation complex, binds to AID during active transcription at Igv and S regions, linking ncRNA surveillance and degradation to AID activity for both SHM and CSR DNA mutation events [Citation75]. From these studies emerges a model wherein the physiological orientation of transcription in the Igh locus is necessary for R loop initiation, while prolonged R loop formation in this region is not, and RNA exosomes serve as an AID binding partner ()
Figure 2. Schematic of R-loop mediated mechanisms of Switch region chromatin remodelling and AID recruitment. A. Model by which active transcription complex guides AID directly to G4 DNA duplexes created from R-loop stabilization/collapse of the Switch region. B. Model by which the germline transcript (GLT) of the Switch region is processed to form RNA G4 complexes, which bind AID and provide sequence homology to Switch region sites whereby AID can bind G4 DNA duplexes. C. Model where in addition to GLT processing from B, DDX1 helicase activity is necessary for converting RNA G4 substrates into R-loops that releases AID to bind to DNA G4 substrates D. Model where RNA exosome surveillance of GLT leads to the recruitment of AID to both DNA strands. All of these models could be occurring simultaneously for efficient DNA targeting and CSR
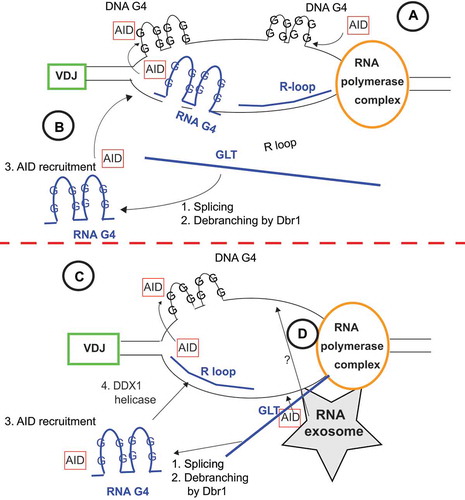
Recent protein crystallization studies have shown that AID binds directly to DNA G4 structures generated at the G-rich non-template ssDNA during transcription at the Igh S regions [Citation76]. From these data, it appears that DNA G4 structures influence AID recruitment, and R loops are only necessary in that they facilitate the formation of DNA G4 structures on non-template DNA strands, and their collapse could facilitate guiding of AID to both strands [Citation74]. RNA G4 structures arising from GLT processing and intronic lariat debranching can bind AID as well, and limiting the production of these RNA G4 substrates by ablating the enzyme Dbr1 reduced CSR [Citation77] (). Furthermore, it has recently been speculated that these RNA G4 AID substrates may be processed by RNA helicase DDX1 to facilitate AID localization [Ribeiro Citation78] (). While genetic ablation of mediators of these processing events diminished CSR frequency, they did not completely abolish it, indicating redundancy with other mechanisms of AID recruitment like those from interactions with RNA polymerase II, other transcriptional machinery and RNA exosome complexes [Citation79–81]. DNA G4 structures arising from R loop formation serve as replication origin binding sites, plausibly facilitating CSR beyond an AID directing mechanism and through mitigation of DNA replication events [Citation82]. Without physiologically oriented GLT, CSR is fundamentally impaired. However, the full extent to which these noncoding transcripts are processed and the mechanistic action they have on recruiting AID and other proteins to S regions to facilitate DNA breaks requires further study. While R-loops and G4 structures have been implicated in the targeting of AID to the Igh S regions during CSR, the mechanism that recruits AID to Igv segments during SHM is clearly different, as there is no evidence of R-loop formation or G4 structures being formed at these regions.
Long noncoding RNAs
Additional lncRNAs have also been implicated in controlling isotype-specific CSR [Citation83–85]. LncRNAs can act as miRNA sponges, inhibiting their effects on coding mRNA targets [Citation86]. It is also well established that lncRNAs can modulate chromatin and act as enhancers for other genes [Citation87]. In a recent study, a newfound lncRNA-CSRIgA and 2 other RNA expressing elements were elucidated megabases downstream of the Igh coding region [Citation85]. B cells isolated from a mouse lacking transcription of lncRNA-CSRIgA fail to undergo normal levels of CSR to IgA and IgG2b in vitro and to IgA in gut-associated lymphoid organs called Peyer’s patches in vivo. lncRNA-CSRIgA is necessary for coordination of regulatory proteins to nearby CTCF sites that orchestrate long-range chromatin changes and interactions with the 3ʹ region of the Igh locus. Chromatin loop extrusion by a CTCF and cohesin-dependent mechanism is necessary for the physical orientation and organization of S sites and efficient CSR [Citation88]. It could be that regulation of CTCF/cohesin binding in the lncRNA locus also modulates chromatin loop extrusion in the neighbouring Igh locus as well. The epigenetic regulation of multiple topologically associated domains of chromatin interacting through active transcription of a lncRNA is an interesting mechanism of gene regulation, and the influence of this process on other sites of the genome will require further study.
Concluding remarks
Diverse species of ncRNA influence B cell responses and CSR through distinct modes of action. MiRNAs regulate gene expression networks through direct control of limiting mRNA targets in a cell state-dependent fashion during B cell maturation and activation. GLT in the Igh locus leads to R-loop mediated regulation of CSR. And more distally transcribed lncRNAs mediate topological associated domain regulation and chromatin dynamics necessary for efficient CSR. While miRNAs have been extensively studied, there is more to glean from studying cell type and state-specific miRNA:target networks as a means of discovering novel genes and pathways that control their unique biology. This work will be facilitated by improvement in CLIP techniques that provide global maps of miRNA binding. The diverse mechanisms by which lncRNAs operate presents a broad new horizon for discovery of ncRNAs and the modes by which they dictate cellular function.
Acknowledgments
We thank Dr. Jayanta Chaudhuri for critical reading of the manuscript.
Disclosure statement
The authors have no conflicting interests to report.
Additional information
Funding
References
- Coffre M, Koralov SB. miRNAs in B cell development and lymphomagenesis. Trends Mol Med. 2017;23:721–736.
- Zheng B, Xi Z, Liu R, et al. The function of MicroRNAs in B-Cell development, lymphoma, and their potential in clinical practice. Front Immunol. 2018;9:936.
- Bottini S, Pratella D, Grandjean V, et al. Recent computational developments on CLIP-seq data analysis and microRNA targeting implications. Brief Bioinform. 2018;19:1290–1301.
- Lee FCY, Ule J. Advances in CLIP technologies for studies of protein-RNA interactions. Mol Cell. 2018;69:354–369.
- Ule J, Hwang HW, Darnell RB. The future of cross-linking and immunoprecipitation (CLIP). Cold Spring Harb Perspect Biol. 2018;10. DOI:10.1101/cshperspect.a032243
- Cyster JG, Allen CDC. B cell responses: cell interaction dynamics and decisions. Cell. 2019;177:524–540.
- Yewdell WT, Chaudhuri J. A transcriptional serenAID: the role of noncoding RNAs in class switch recombination. Int Immunol. 2017;29:183–196.
- Yu K, Lieber MR. Current insights into the mechanism of mammalian immunoglobulin class switch recombination. Crit Rev Biochem Mol Biol. 2019;54:333–351.
- Ramirez J, Lukin K, Hagman J. From hematopoietic progenitors to B cells: mechanisms of lineage restriction and commitment. Curr Opin Immunol. 2010;22:177–184.
- Mesin L, Ersching J, Victora GD. Germinal center B cell dynamics. Immunity. 2016;45:471–482.
- Roco JA, Mesin L, Binder SC, et al. Class-Switch recombination occurs infrequently in germinal centers. Immunity. 2019;51:337–350 e337.
- Lee RC, Feinbaum RL, Ambros V. The C. elegans heterochronic gene lin-4 encodes small RNAs with antisense complementarity to lin-14. Cell. 1993;75:843–854.
- O’Brien J, Hayder H, Zayed Y, et al. Overview of MicroRNA biogenesis, mechanisms of actions, and circulation. Front Endocrinol (Lausanne). 2018;9:402.
- Bartel DP. MicroRNAs: genomics, biogenesis, mechanism, and function. Cell. 2004;116:281–297.
- Broughton JP, Lovci MT, Huang JL, et al. Pairing beyond the seed supports MicroRNA targeting specificity. Mol Cell. 2016;64:320–333.
- Jonas S, Izaurralde E. Towards a molecular understanding of microRNA-mediated gene silencing. Nat Rev Genet. 2015;16:421–433.
- Wang T, Xiao G, Chu Y, et al. Design and bioinformatics analysis of genome-wide CLIP experiments. Nucleic Acids Res. 2015;43:5263–5274.
- Chi SW, Zang JB, Mele A, et al. Argonaute HITS-CLIP decodes microRNA-mRNA interaction maps. Nature. 2009;460:479–486.
- Hafner M, Landthaler M, Burger L, et al. Transcriptome-wide identification of RNA-binding protein and microRNA target sites by PAR-CLIP. Cell. 2010;141:129–141.
- Hsin JP, Lu Y, Loeb GB, et al. The effect of cellular context on miR-155-mediated gene regulation in four major immune cell types. Nat Immunol. 2018;19:1137–1145.
- Huppertz I, Attig J, D’Ambrogio A, et al. iCLIP: protein-RNA interactions at nucleotide resolution. Methods. 2014;65:274–287.
- Loeb GB, Khan AA, Canner D, et al. Transcriptome-wide miR-155 binding map reveals widespread noncanonical microRNA targeting. Mol Cell. 2012;48:760–770.
- Jin HY, Oda H, Lai M, et al. MicroRNA-17~92 plays a causative role in lymphomagenesis by coordinating multiple oncogenic pathways. Embo J. 2013;32:2377–2391.
- Gagnon JD, Kageyama R, Shehata HM, et al. miR-15/16 restrain memory T cell differentiation, cell cycle, and survival. Cell Rep. 2019;28:2169–2181 e2164.
- Wang T, Xie Y, Xiao G. dCLIP: a computational approach for comparative CLIP-seq analyses. Genome Biol. 2014;15:R11.
- Uren PJ, Bahrami-Samani E, Burns SC, et al. Site identification in high-throughput RNA-protein interaction data. Bioinformatics. 2012;28:3013–3020.
- Erhard F, Dolken L, Jaskiewicz L, et al. PARma: identification of microRNA target sites in AGO-PAR-CLIP data. Genome Biol. 2013;14:R79.
- Brandl A, Daum P, Brenner S, et al. The microprocessor component, DGCR8, is essential for early B-cell development in mice. Eur J Immunol. 2016;46:2710–2718.
- Koralov SB, Muljo SA, Galler GR, et al. Dicer ablation affects antibody diversity and cell survival in the B lymphocyte lineage. Cell. 2008;132:860–874.
- de Yebenes VG, Bartolome-Izquierdo N, Ramiro AR. Regulation of B-cell development and function by microRNAs. Immunol Rev. 2013;253:25–39.
- Belver L, de Yebenes VG, Ramiro AR. MicroRNAs prevent the generation of autoreactive antibodies. Immunity. 2010;33:713–722.
- Kramer NJ, Wang WL, Reyes EY, et al. Altered lymphopoiesis and immunodeficiency in miR-142 null mice. Blood. 2015;125:3720–3730.
- King JK, Ung NM, Paing MH, et al. Regulation of marginal zone B-Cell differentiation by MicroRNA-146a. Front Immunol. 2016;7:670.
- Xiao C, Calado DP, Galler G, et al. MiR-150 controls B cell differentiation by targeting the transcription factor c-Myb. Cell. 2007;131:146–159.
- Akkaya M, Traba J, Roesler AS, et al. Second signals rescue B cells from activation-induced mitochondrial dysfunction and death. Nat Immunol. 2018;19:871–884.
- Waters LR, Ahsan FM, Wolf DM, et al. Initial B cell activation induces metabolic reprogramming and mitochondrial remodeling. iScience. 2018;5:99–109.
- Fowler T, Garruss AS, Ghosh A, et al. Divergence of transcriptional landscape occurs early in B cell activation. Epigenetics Chromatin. 2015;8:20.
- Kuchen S, Resch W, Yamane A, et al. Regulation of microRNA expression and abundance during lymphopoiesis. Immunity. 2010;32:828–839.
- Xu S, Guo K, Zeng Q, et al. The RNase III enzyme Dicer is essential for germinal center B-cell formation. Blood. 2012;119:767–776.
- Clurman BE, Hayward WS. Multiple proto-oncogene activations in avian leukosis virus-induced lymphomas: evidence for stage-specific events. Mol Cell Biol. 1989;9:2657–2664.
- Rodriguez A, Vigorito E, Clare S, et al. Requirement of bic/microRNA-155 for normal immune function. Science. 2007;316:608–611.
- Thai TH, Calado DP, Casola S, et al. Regulation of the germinal center response by microRNA-155. Science. 2007;316:604–608.
- Vigorito E, Perks KL, Abreu-Goodger C, et al. microRNA-155 regulates the generation of immunoglobulin class-switched plasma cells. Immunity. 2007;27:847–859.
- Arbore G, Henley T, Biggins L, et al. MicroRNA-155 is essential for the optimal proliferation and survival of plasmablast B cells. Life Sci Alliance. 2019;2. DOI:10.26508/lsa.201800244
- Nakagawa R, Leyland R, Meyer-Hermann M, et al. MicroRNA-155 controls affinity-based selection by protecting c-MYC+ B cells from apoptosis. J Clin Invest. 2016;126:377–388.
- Dorsett Y, McBride KM, Jankovic M, et al. MicroRNA-155 suppresses activation-induced cytidine deaminase-mediated Myc-Igh translocation. Immunity. 2008;28:630–638.
- Teng G, Hakimpour P, Landgraf P, et al. MicroRNA-155 is a negative regulator of activation-induced cytidine deaminase. Immunity. 2008;28:621–629.
- Lu D, Nakagawa R, Lazzaro S, et al. The miR-155-PU.1 axis acts on Pax5 to enable efficient terminal B cell differentiation. J Exp Med. 2014;211:2183–2198.
- Lu LF, Gasteiger G, Yu IS, et al. A single miRNA-mRNA interaction affects the immune response in a context- and cell-type-specific manner. Immunity. 2015;43:52–64.
- Jiang S, Yan W, Wang SE, et al. Let-7 suppresses B cell activation through restricting the availability of necessary nutrients. Cell Metab. 2018;27:393–403 e394.
- Sanchez HN, Moroney JB, Gan H, et al. B cell-intrinsic epigenetic modulation of antibody responses by dietary fiber-derived short-chain fatty acids. Nat Commun. 2020;11:60.
- Weisel FJ, Mullett SJ, Elsner RA, et al. Germinal center B cells selectively oxidize fatty acids for energy while conducting minimal glycolysis. Nat Immunol. 2020;21:331–342.
- Xu S, Ou X, Huo J, et al. Mir-17-92 regulates bone marrow homing of plasma cells and production of immunoglobulin G2c. Nat Commun. 2015;6:6764.
- Xiao C, Srinivasan L, Calado DP, et al. Lymphoproliferative disease and autoimmunity in mice with increased miR-17-92 expression in lymphocytes. Nat Immunol. 2008;9:405–414.
- He L, Thomson JM, Hemann MT, et al. A microRNA polycistron as a potential human oncogene. Nature. 2005;435:828–833.
- Ventura A, Young AG, Winslow MM, et al. Targeted deletion reveals essential and overlapping functions of the miR-17 through 92 family of miRNA clusters. Cell. 2008;132:875–886.
- Lai M, Gonzalez-Martin A, Cooper AB, et al. Regulation of B-cell development and tolerance by different members of the miR-17 approximately 92 family microRNAs. Nat Commun. 2016;7:12207.
- Wu Y, Schutt S, Paz K, et al. MicroRNA-17-92 is required for T-cell and B-cell pathogenicity in chronic graft-versus-host disease in mice. Blood. 2018;131:1974–1986.
- Olive V, Bennett MJ, Walker JC, et al. miR-19 is a key oncogenic component of mir-17-92. Genes Dev. 2009;23:2839–2849.
- Simpson LJ, Patel S, Bhakta NR, et al. A microRNA upregulated in asthma airway T cells promotes TH2 cytokine production. Nat Immunol. 2014;15:1162–1170.
- Psathas JN, Doonan PJ, Raman P, et al. The Myc-miR-17-92 axis amplifies B-cell receptor signaling via inhibition of ITIM proteins: a novel lymphomagenic feed-forward loop. Blood. 2013;122:4220–4229.
- Hines MJ, Coffre M, Mudianto T, et al. miR-29 sustains B cell survival and controls terminal differentiation via regulation of PI3K signaling. Cell Rep. 2020;33:108436.
- O’Connell RM, Chaudhuri AA, Rao DS, et al. Inositol phosphatase SHIP1 is a primary target of miR-155. Proc Natl Acad Sci U S A. 2009;106:7113–7118.
- Fernandez-Messina L, Rodriguez-Galan A, de Yebenes VG, et al. Transfer of extracellular vesicle-microRNA controls germinal center reaction and antibody production. EMBO Rep. 2020;21:e48925.
- Chiou NT, Kageyama R, Ansel KM. Selective export into extracellular vesicles and function of tRNA fragments during T cell activation. Cell Rep. 2018;25:3356–3370 e3354.
- de Candia P, Torri A, Gorletta T, et al. Intracellular modulation, extracellular disposal and serum increase of MiR-150 mark lymphocyte activation. PLoS One. 2013;8:e75348.
- de Yebenes VG, Belver L, Pisano DG, et al. miR-181b negatively regulates activation-induced cytidine deaminase in B cells. J Exp Med. 2008;205:2199–2206.
- Recaldin T, Hobson PS, Mann EH, et al. miR-29b directly targets activation-induced cytidine deaminase in human B cells and can limit its inappropriate expression in naive B cells. Mol Immunol. 2018;101:419–428.
- Pavri R. R loops in the regulation of antibody gene diversification. Genes (Basel). 2017;8. DOI:10.3390/genes8060154
- Roy D, Yu K, Lieber MR. Mechanism of R-loop formation at immunoglobulin class switch sequences. Mol Cell Biol. 2008;28:50–60.
- Shinkura R, Tian M, Smith M, et al. The influence of transcriptional orientation on endogenous switch region function. Nat Immunol. 2003;4:435–441.
- Yeap LS, Hwang JK, Du Z, et al. Sequence-intrinsic mechanisms that target AID mutational outcomes on antibody genes. Cell. 2015;163:1124–1137.
- Maul RW, Chon H, Sakhuja K, et al. R-loop depletion by over-expressed RNase H1 in mouse B cells increases activation-induced deaminase access to the transcribed strand without altering frequency of isotype switching. J Mol Biol. 2017;429:3255–3263.
- Yu K, Chedin F, Hsieh CL, et al. R-loops at immunoglobulin class switch regions in the chromosomes of stimulated B cells. Nat Immunol. 2003;4:442–451.
- Basu U, Meng FL, Keim C, et al. The RNA exosome targets the AID cytidine deaminase to both strands of transcribed duplex DNA substrates. Cell. 2011;144:353–363.
- Qiao Q, Wang L, Meng FL, et al. AID Recognizes structured DNA for class switch recombination. Mol Cell. 2017;67:361–373 e364.
- Zheng S, Vuong BQ, Vaidyanathan B, et al. Non-coding RNA generated following lariat debranching mediates targeting of AID to DNA. Cell. 2015;161:762–773.
- de Almeida R, Dhir CS, Dhir A, et al. RNA helicase DDX1 converts RNA G-quadruplex structures into R-loops to promote IgH class switch recombination. Mol Cell. 2018;70:650–662 e658.
- Laffleur B, Basu U, Lim J. RNA exosome and non-coding RNA-coupled mechanisms in AID-mediated genomic alterations. J Mol Biol. 2017;429:3230–3241.
- Nambu Y, Sugai M, Gonda H, et al. Transcription-coupled events associating with immunoglobulin switch region chromatin. Science. 2003;302:2137–2140.
- Pavri R, Gazumyan A, Jankovic M, et al. Activation-induced cytidine deaminase targets DNA at sites of RNA polymerase II stalling by interaction with Spt5. Cell. 2010;143:122–133.
- Wiedemann EM, Peycheva M, Pavri R. DNA replication origins in immunoglobulin switch regions regulate class switch recombination in an R-loop-dependent manner. Cell Rep. 2016;17:2927–2942.
- Pefanis E, Wang J, Rothschild G, et al. Noncoding RNA transcription targets AID to divergently transcribed loci in B cells. Nature. 2014;514:389–393.
- Pefanis E, Wang J, Rothschild G, et al. RNA exosome-regulated long non-coding RNA transcription controls super-enhancer activity. Cell. 2015;161:774–789.
- Rothschild G, Zhang W, Lim J, et al. Noncoding RNA transcription alters chromosomal topology to promote isotype-specific class switch recombination. Sci Immunol. 2020;5. DOI:10.1126/sciimmunol.aay5864
- Karreth FA, Reschke M, Ruocco A, et al. The BRAF pseudogene functions as a competitive endogenous RNA and induces lymphoma in vivo. Cell. 2015;161:319–332.
- Nair L, Chung H, Basu U. Regulation of long non-coding RNAs and genome dynamics by the RNA surveillance machinery. Nat Rev Mol Cell Biol. 2020;21:123–136.
- Zhang X, Zhang Y, Ba Z, et al. Fundamental roles of chromatin loop extrusion in antibody class switching. Nature. 2019;575:385–389.