ABSTRACT
RNA modifications play a vital role in multiple pathways of mRNA metabolism, and translational regulation is essential for immune cells to promptly respond to stimuli and adapt to the microenvironment. N6-methyladenosine (m6A) methylation, which is the most abundant mRNA modification in eukaryotes, primarily functions in the regulation of RNA splicing and degradation. However, the role of m6Amethylation in translational control and its underlying mechanism remain controversial. The role of m6A methylation in translation regulation in immune cells has received relatively limited attention. In this review, we aim to provide a comprehensive summary of current studies on the translational regulation of m6A modifications and recent advances in understanding the translational control regulated by RNA modifications during the immune response. Furthermore, we envision the possible pathways through which m6A modifications may be involved in the regulation of immune cell function via translational control.
Introduction
Gene expression undergoes post-transcriptional regulation by multiple steps via mRNA splicing, mRNA export from the nucleus to the cytosol, mRNA stability, and mRNA translation to produce proteins [Citation1,Citation2]. N6-methyladenosine (m6A) methylation, initially discovered in the 1970s, is the most abundant modification of mRNA in eukaryotes [Citation3]. It is widely involved in the regulatory processes of gene expression, including transcript splicing, export from the nucleus, stability, decay, translation efficiency, and RNA–protein interactions [Citation4–6]. Numerous mRNAs containing multiple m6A modification sites are associated with pathways that control cell fate, differentiation and morphogenesis [Citation7], and thus m6A modification play radical roles in development, organism homoeostasis and disease. We also summarized and discussed the functional relevance of m6A modification in different cells under normal or disease conditions in our previous publications [Citation8–11]. For instance, m6A methyltransferase METTL3 or METTL14 was found to promote homoeostatic expansion of naïve CD4+ T cell in vivo during the adoptive transfer colitis model. Mechanistically, m6A-modified mRNAs of the suppressor of cytokine signalling (SOCS) family genes underwent rapid degradation in response to interleukin (IL)-7 stimulation, thereby initiating the homoeostasis and differentiation of naïve T cells by sparing the IL-7-signal transducers and activators of transcription 5 (STAT5) pathway [Citation10]. Ito-Kureha et al. found that deficiency of Wtap (an essential scaffold protein of the m6A methyltransferase complex) impaired the functionality of RORγt+ iTreg cells in the gut and caused colitis, a phenotype shared with genetic inactivation of Mettl3 or Mettl14. Conditional deletion of Wtap with CD4-Cre downregulated CD8 co-receptor and T cell antigen-receptor (TCR)-β expression in single-positive thymocytes. Moreover, Wtap-deficient T cells die upon TCR-induced activation. In activation-induced T cell death, m6A modification and Wtap inhibition functioned by influencing mRNA decay of the Ca2+ channel Orai1 and the programmed cell death-inducible kinase Ripk1 [Citation12]. Differences in deletion efficiency, timepoint of deletion or compensatory changes may explain the different phenotypes produced by different mouse models of different components of the methyltransferase complex. While the regulation of RNA splicing and degradation by m6A has been well documented and abundantly reviewed, the regulation of translation by m6A and its mechanisms remain highly controversial [Citation13,Citation14].
Biological processes associated with the immune response are under precise and selective control of gene expression, including cytokine production, immune cell activation, differentiation homoeostatic expansion and innate antiviral immunity [Citation15]. mRNA translation, which enables rapid changes in the proteome without de novo transcription, plays a crucial role in facilitating immediate responses to changing environmental conditions. This capability allows organisms to mobilize host defence and coordinate immune responses to infections efficiently [Citation16]. It was found that naïve T cells maintain a large number of idle ribosomes, as well as multiple repressed mRNAs and a reservoir of glycolytic enzymes. These components engage rapidly upon stimulation, facilitating immediate translational and glycolytic conversion to accelerate the T cell activation program [Citation17]. Our recent study has also identified a novel mechanism by which N1-methyladenosine (m1A)-modified tRNAs control T cell homoeostasis and signalling-dependent translational control of specific key proteins, further highlighting the importance of the translational control in immune cells [Citation18]. Evidence suggests crosstalk between different RNA modifications. HRSP12 as an RNA-binding protein that recognized m1A and Boo et al. found that the binding of HRSP12 to m1A promoted efficient interaction of YTHDF2 with m6A, consequently promoting endoribonucleolytic cleavage via the RNase P/MRP complex. In addition, m1A and m6A modifications synergistically promote rapid mRNA degradation [Citation19]. The cooperative role of m1A and m6A modifications in translation regulation warrants further exploration, as understanding their synergistic effects can enhance our comprehension of the regulatory mechanisms involving m6A. In this review, we will summarize the recent progress of translational control regulated by RNA modifications during the immune response, with a particular focus on m6A modifications, and explore the limitations of current studies.
Introduction to m6A RNA modification
The m6A methyltransferase complex consists of METTL3, METTL14, and WTAP, each playing a distinct role in catalysing m6A modification. METTL3 is the first protein identified as an S-adenosylmethionine-binding subunit complex with the function of catalytic m6A modification [Citation20,Citation21]. METTL14 and METTL3 form a heterodimer [Citation22], and as METTL14 is primarily responsible for recognizing and localizing subunits. Together, they cooperate with each other in vivo to catalyse m6A modification of mRNA [Citation23–25]. WTAP promotes m6A modification of mRNA by recruiting METTL3-METTL14 heterodimers co-localized in nucleolar patches [Citation26]. ZC3H13-WTAP-Virilizer-Hakai complex has also been identified as an important regulatory complex of RNA m6A and ZC3H13 is required for nuclear localization of other components, as well as METTL3 and METTL14 [Citation27]. In addition to mRNA, m6A modifications are also present in other types of RNAs, including rRNA, long non-coding RNA (lncRNA), microRNA and small nuclear RNA (snRNA). Recent studies have revealed that METTL5 methylates 18S rRNA [Citation28], while ZCCHC4 acts as a eukaryotic 28S m6A methylation transferase [Citation29]. RNA-binding motif protein 15 (RBM15) and its paralog RBM15B can mediate m6A formation of the long non-coding RNA [Citation30]. METTL16 is a functionally specific methyltransferase that independently affects m6A modification and is involved in the methylation modification of U6 snRNA, long non-coding RNAs, and some mRNAs [Citation31,Citation32].
Fat mass and obesity-associated protein (FTO), the first identified RNA demethylase, is responsible for removing remove mRNA m6A [Citation33]. FTO can also regulate m1A and m6Am, another form of m6A, which is enriched at the cap of a portion of mRNA and certain small nuclear RNAs [Citation34,Citation35]. Furthermore, AlkB homologue 5 (ALKBH5)-mediated m6A erasure controls the splicing and stability of long 3’-UTR mRNAs in cells [Citation36]. There could be unknown m6A demethylase to be identified.
In addition to methyltransferases and demethylases, m6A modifications of RNAs require binding to m6A-binding proteins that specifically recognize the m6A sites to regulate RNA metabolism. The fate of m6A-modified mRNAs is dependent on the function of the m6A-binding proteins that recognize them, which may affect the stability, splicing, and/or translation of the target mRNAs [Citation6]. Up to now, several m6A binding proteins have been identified, among which the family proteins of YTH structural domain, insulin-like growth factor 2 mRNA binding protein family and eukaryotic translation initiation factor 3 (EIF3) have been the most widely studied. While numerous review articles have focused onm6A’s role in RNA stability and splicing, we will emphasize the RNA translation regulation by m6A and its binding proteins in immune cells.
m6A-binding proteins regulate mRNA translation
YTHDF family members
In earlier studies, members of the YTHDF family were found to play different functions in m6A modification. YTHDF1 improving translation efficiency by interacting with the translation machinery [Citation37], while YTHDF2 was associated with mRNA degradation by recruiting the CCR4-NOT deadenylation complex [Citation38]. Jin et al. found that YTHDF1, YTHDF2, and YTHDF3 reciprocally and physically interacted with each other via endogenous co-IP experiments. In Non-small cell lung cancer (NSCLC) tumour and normal tissues, the expression of YAP was regulated by m6A modification, whereby balancing the function of YTHDF1 and YTHDF2 via the YTHDF3 hub. Specifically, the m6A modification of YAP pre-mRNA was first recognized by YTHDF3, followed by a competitive binding of YTHDF1 and YTHDF2 to YTHDF3, which determined the fate of YAP pre-mRNA. After YTHDF2 binding to YTHDF3, which carried YAP pre-mRNA with m6A, YTHDF2 presented YAP mRNA to AGO2, which then recruited other molecules to form RISC system to promote YAP mRNA degradation. Following YTHDF1 binding of YTHDF3, which carried m6A-modified YAP pre-mRNA, YTHDF1 presented YAP mRNA to eIF3a-contained translation initiation complex to promote translation of YAP mRNA, leading to an increase in YAP protein level [Citation39].
Several investigations have identified the role of YTHDF1-mediated translation in immune cells ). METTL3 mediated mRNA m6A methylation in the 3’UTR region of TLR4 signalling adaptor Tirap, CD40, and CD80 in dendritic cell (DC). Tirap mRNA level was similar in Mettl3KO DC compared with Mettl3WT DC, CD40 and CD80 had similar mRNA level but decreased protein level in Mettl3 KO DC. Tirap, CD80, and CD40 were all found to have a lower translation efficiency in Mettl3KO mature DC (maDC) compared with that in Mettl3WT maDC, which was verified by qPCR of ribosome-associated RNA separated from 80S monosome fraction and genome-wide ribosome profiling. The m6A-dependent translational regulation was mediated by YTHDF1, thereby stimulating T cell activation and enhancing TLR4/NF-κB signalling-induced cytokine production [Citation40]. Persistent neoantigen-specific immunity was found to be regulated by m6A methylation of mRNA through YTHDF1. Han et al. demonstrated a significant reduction in the translation efficiency of Ythdf1−/− DCs compared to wild-type DCs, as revealed by ribosome profiling, particularly for YTHDF1-targeted and m6A-marked transcripts. YTHDF1 recognized m6A-tagged transcripts encoding lysosomal proteases, thereby increasing translation of lysosomal proteases in DCs, which in turn inhibited cross-presentation of dendritic cells and cross-priming of CD8+ T cells. YTHDF1 deficient mice displayed elevated antigen-specific CD8+ T cell antitumor responses, and the therapeutic efficacy of PD-L1 checkpoint blockade was enhanced in Ythdf1−/− mice [Citation41]. Although there is no direct evidence that YTHDF1 regulates T cell translation, Li et al. identified that YTHDF1 as an immunosuppressive molecule for T cell functions in diverse tumours by analysing the tumour immune microenvironment. YTHDF1 regulated immunosuppressive molecules PD-L1 and VISTA expression in an m6A-dependent manner. Although YTHDF1 knockdown did not affect the transcriptional levels of PD-L1 and VISTA, polysome profiling demonstrated that YTHDF1 knockdown resulted in reduced translation efficiency of PD-L1 and VISTA mRNA in colorectal cancer (CRC) cells [Citation42].
Figure 1. The role of m6A-binding proteins on regulating mRNA translation. (A) the YTHDF family is involved in mRNA translation. (B) the IGF2BP family regulates mRNA translation. (C) role of other m6A-binding proteins in mRNA translation. Need to tap into the existing m6A binding proteins for translation regulation in immune cells and explore the role of novel m6A binding proteins within it.
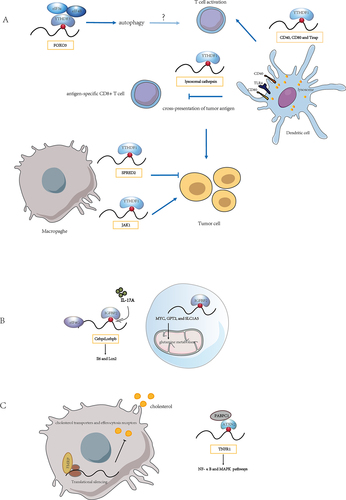
Tumour-infiltrating myeloid cells (TIMs) are crucial cell populations involved in tumour immune escape. YTHDF1 recognized METTL3-mediated m6A modification of JAK1 mRNA in TIMs, and METTL3-m6A-YTHDF1 enhanced Jak1 mRNA translation in polysome with subsequent promotion of STAT3 phosphorylation. Remarkably, deletion of METTL3 in myeloid attenuated tumour growth in mice [Citation43]. Yang et al. observed that METTL3-deficient mice exhibited increased infiltration of M1 or M2 tumour-associated macrophages (TAM) and regulatory T cells within tumours, resulting in impaired efficacy of PD-1 checkpoint blockers. The mRNA decay assays indicated that m6A modification did not significantly affect the decay of Spred2, whereas polysome profiling and qRT-PCR showed that the Spred2 mRNA level in translation-active polysomes of Mettl3-deficient bone marrow-derived macrophages (BMDMs) was significantly lower than that in WT BMDMs. Moreover, YTHDF1 mediated the translation of SPRED2, leading to enhanced NF-κB activation and promoting tumour progression [Citation44]. It appears that in different cell types, YTHDF1 exerts its influence on cellular functions by recognizing different target genes and regulating their translation.
In addition to YTHDF1, recent studies have revealed that loss of function of m6A modification due to METTL3 depletion inhibits YTHDF3-mediated autophagic flux. Through profiling global proteome changes in mouse embryonic fibroblasts (MEFs) with nutrient starvation, YTHDF3 was detected to be significantly upregulated during nutrient deficiency. m6A modification sites around the FOXO3 mRNA stop codon were recognized by YTHDF3 through the recruitment of eIF3A and eIF4B to promote FOXO3 translation and subsequently initiate autophagy [Citation45]. Autophagy is involved in maintaining cellular energy homoeostasis and cellular adaptation to nutrient deficiencies. Considering the crucial role of the transcription factor Foxo3 in myeloid cell function and its involvement in the polarization of CD4+ T cells into pathogenic T helper 1 (Th1) cells, which produce interferon-γ (IFN-γ) and granulocyte monocyte colony-stimulating factor (GM-CSF) [Citation46], it is plausible that YTHDF3-mediated translation regulation may also impact T cell function. Furthermore, inhibition of ALKBH5 can effectively inhibit the growth of MYC-dysregulated B-cell lymphoma. Mechanistically, MYC down-regulated the protein expression of specific MYC-repressed genes (MRGs) by reducing m6A modification through ALKBH5. RIP assay results indicated that YTHDF3 exclusively bound to the MRG transcripts SPI1 and PHF12, thereby functioning in MYC-mediated MRG expression via m6A modification. Polysome profiling analysis suggested that YTHDF3 regulated the translation of MRG transcripts [Citation47].
Despite numerous studies suggesting the involvement of the YTHDF family in mRNA translation and regulation, there are contrasting perspectives in the literature. Jaffrey et al. reported that YTHDF family members do not induce translation in HeLa cells [Citation48], and their roles in regulating stability and differentiation become apparent only when all three YTHDF family members were depleted simultaneously. Furthermore, YTHDF1 promotes the translation of the m6A-modified mRNA it recognizes through its interaction with eIF3 in previous studies, which appears to involve a different mechanism from the typical role of eIF3 in translation. EIF3 is known to facilitate the interaction of initiation factors and ribosomes necessary for productive translation. It binds to highly specific mRNA programs through the mRNA 5’ UTR and functions as a translational activator or repressor through various RNA stem-loop binding modes [Citation49]. Nonetheless, some published YTHDF1 binding m6A sites were located in the 3’UTR region. Recently, Zou et al. demonstrated that YTHDF1 indeed promotes translation in HeLa cells using YTHDF1 target transcripts rather than all methylated mRNA for analysis, and they identified the key approaches in the analyses of Zaccara et al. that may contribute to divergent conclusions [Citation50]. Only ~753 high confidence transcripts were directly bound by YTHDF1 as identified with photoactivatable ribonucleoside-enhanced crosslinking and immunoprecipitation (PAR-CLIP). While Zaccara et al. investigated the effects of YTHDF1 knockdown on translation or stability of ~60% of the transcriptome, yet YTHDF1 primarily bound only ~ 10% of the transcriptome. Thus, the effects of YTHDF1 knockdown on the other m6A-modified mRNA could be indirect, which may be a functional consequence of the ~50% of m6A-modified mRNAs not bound by YTHDFs analysed by Zaccara et al. In addition, exploring the function of YTHDF proteins in translation and decay based only on actively translated RNA may lead to the inclusion of indirect effects in the analysis [Citation50]. Hu et al. also defined mRNA by m6A-selective allyl chemical labelling and sequencing (m6A-SAC-seq), a novel method for quantitative and whole-transcriptome mapping of m6A at single-nucleotide resolution. The m6A fractions of transcripts showed an effect on translation efficiency, with knockdown of either METTL3 or YTHDF1 further decreasing translation efficiency. Translation efficiency can be notably perturbed by the m6A sites located in the CDS but less in the 3’UTR and 5’UTR [Citation51]. Thus, when analysing the role of YTHDF proteins in translational regulation, it is necessary to consider the m6A modification sites and the specificity of the targets, and as well as to apply appropriate investigation approaches and account for possible indirect effects of them, such as those involving other RNA-binding proteins.
IGF2BP protein family
IGF2BP protein family members, which stabilize m6A-containing mRNAs and promote their translation through their K homology (KH) domains [Citation52]. Excessive cytokine activity underlies many autoimmune diseases, particularly through the interleukin-17 (IL-17) and tumour necrosis factor-α (TNFα) signalling axis. IGF2BP2 occupation of Cebpd and Cebpb mRNAs was enhanced by m6A modification in MEFs and human renal epithelial cells (HK-2). IGF2BP2 promoted IL-17-mediated stabilization of Cebpd mRNA and facilitated the translation of C/EBPβ/δ in response to IL-17A, IL-17F and TNF, which was detected by performing RIP of eIF4G (eukaryotic initiation factor 4 gamma) and assessing the occupancy of Cebpd and Cebpb mRNAs within the translation initiation complex [Citation53]. Yu et al. identifiedIGF2BP2 recruited EIF proteins, such as EIF4E and EIF3A, to MYC, GPT2 and SLC1A5 mRNA to fine-tune their translation and regulated glutamine metabolism in acute myeloid leukaemia [Citation54]. Glutamine has been recognized as an immunomodulatory nutrient [Citation55], and glutamine metabolism tailored the immune responses of macrophages via metabolic and epigenetic reprogramming [Citation56]. Therefore, it will be fascinating to explore in future research whether IGF2BP affects glutamine metabolism through translational regulation and thus participates in the immune response ).
Other readers
‘Reader’ proteins directly bind and recognize m6A marks on RNA, influencing various RNA fate processes such as splicing, export, stability, and translation. Recently, a number of novel m6A readers have been identified. In oesophageal squamous cell carcinoma (ESCC) cells, Li et al. found that altering METTL3 levels did not significantly alter TNFRSF1A (also known as TNFR1) mRNA level. However, increased m6A modification of the TNFR1 transcript affected its protein level. Common m6A recognition proteins, namely ‘reader’ proteins (including YTHDF1–3, YTHDC1–2 and IGF2BP1–3), failed to interact with TNFR1 transcripts, suggesting that there may be other functional proteins involved in this process. Subsequently, Li et al. identified a novel functional protein called ATXN2, which is involved in m6A methylation and demonstrated its role in enhancing the translation of TNFR1-mediated signalling events by polysome profiling. This activation of the NF-κB and MAPK pathways promoted the development of ESCC [Citation57]. ATXN2 can directly interact with target RNAs via its Lsm and Lsm-AD domains and interact with poly A-binding protein (PABPC1) through its PAM2 motif. The ATXN2-PABPC1 complex plays a role in translation initiation [Citation58,Citation59]. Drosophila FMR1 preferentially binds mRNAs containing m6A-marked ‘AGACU’ motif, which is mainly dependent on the hydrophobic network within FMR1 KH2 domain and contributes to maternal RNA degradation [Citation60]. FMRP, positively regulated by IRE1, was also found to act as a translation repressor via polyribosome profiling and promote atherosclerosis by affecting macrophage cholesterol metabolism [Citation61]. The discovery of novel m6A-recognizable RBPs will greatly facilitate our understanding of the role of RNA modifications in regulating immune cell function ).
m6A methyltransferases regulates mRNA translation
It is reported that METTL3 depletion inhibited translation, and both wild-type and catalytically inactive METTL3 promoted translation when tethered to a reporter mRNA. Contrary to current models that invoke m6A reader proteins downstream of nuclear METTL3, METTL3 associated with ribosomes and promoted translation in the cytoplasm [Citation62]. Gregory et al. also identified METTL3 enhanced translation only when tethered to a reporter mRNA in close proximity to the stop codon, supporting a mechanism of ribosomal recycling and translational control of the mRNA cycle. And METTL3-eIF3h interaction was required for enhanced translation, formation of dense polyribosomes, and oncogenic transformation [Citation63].
METTL3 was also associated with a large proportion of non-m6A-modified transcripts. In gastric cancer cells, cytoplasmic METTL3 promoted specific interactions between the PABPC1 and eIF4F complex, independent of m6A modification, thereby regulating the translation of non-m6A-modified epigenetic mRNAs. This suggests that mRNA preference for METTL3 May be conferred by other molecular determinants rather than m6A modification [Citation64].
Nucleus METTL3 and cytoplasmic METTL3 May play different roles in the regulation of gene expression and cellular function. The intracellular and extracellular environment affect METTL3 expression, nuclear translocation and function. For instance, lactate accumulated in tumour microenvironment potently induced METTL3 upregulation in TIMs via H3K18 lactylation. Two lactylation modification sites in the zinc-finger domain of METTL3 were essential for METTL3 to capture target RNA for promoting the immunosuppressive capacity of TIMs [Citation43]. Lu et al. reported that IL-6 mRNA transcripts were subject to METTL3-mediated m6A modifications that promoted METTL3 deacetylation and nuclear translocation, thereby inducing global m6A abundance and then impeded cancer metastasis [Citation65]. Innate signals phosphorylated METTL3 at serine 67 via TBK1, a key kinase of antiviral pathways. And METTL3-mediated m6A modification secured antiviral immunity by promoting mRNA stability and protein translation [Citation66]. The investigation of the nuclear translocation of METTL3 and the different targets and mechanisms of nuclear and cytoplasmic METTL3 will be essential to explore the regulatory mechanisms of immune cells in different environments.
In addition, another m6A methyltransferase METTL14 was implicated in m6A modification and the downregulation of P2RX6 protein translation in renal cell carcinoma cells. TP-P2RX6 was involved in regulating Ca2+ mediated p-ERK1/2/MMP9 signalling to increase migration and invasion of renal cell carcinoma cells [Citation67]. As a functionally specific methyltransferase, METTL16 exerted both methyltransferase activity-dependent and independent functions in gene regulation. In the nucleus, METTL16 functioned as an m6A writer, depositing m6A into its hundreds of specific messenger RNA targets. In the cytosol, METTL16 interacted directly with EIF3a and EIF3b, as well as ribosomal RNA, through its Mtase structural domain in an m6A-independent manner. The interaction facilitated the assembly of the translation initiation complex and the translation of mRNA transcripts, which contributed to the tumorigenic effects of hepatocellular carcinoma [Citation68] ().
Figure 2. The role of m6A methyltransferases on regulating mRNA translation. The m6A transferases perform distinct functions in the nucleus and cytoplasm, with translation regulated in an m6A-dependent and m6A-independent manner. The intra- and extracellular environment may influence the nucleoplasmic distribution of m6A transferases.
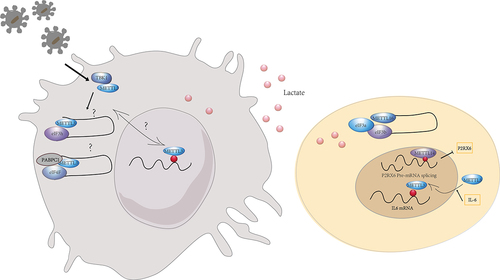
m6A modification regulates ncRNA
While recent research has focused on m6A modifications in mRNAs and their impact on mRNA processing and translation, it has become evident that m6A also played regulatory roles in non-coding RNAs. Extensive pre-mRNA back-splicing generated a number of circular RNAs (circRNAs) in human transcriptome. Consensus m6A motifs are enriched in circRNAs and a single m6A site is sufficient to drive translation initiation. Mao et al. revealed that m6A-driven translation of circRNAs was widespread, with hundreds of endogenous circRNAs having translation potential through polysome profiling, computational prediction and mass spectrometry [Citation69]. This m6A-driven translation required initiation factor eIF4G2 and m6A reader YTHDF3. It was enhanced by methyltransferase METTL3/14, inhibited by demethylase FTO, and upregulated by environmental stress such as heat shock ().
Figure 3. The role of m6A modification on ncRnas. (A) m6A modification mediates the translation of circRnas. (B) m6A modification regulates lncRNA and then lncRNA affects mRNA translation. (C) m6A modification is involved in mRNA translation by affecting rRnas. m6A modifications may affect the translational regulation in immune cells directly or indirectly by impacting ncRnas.
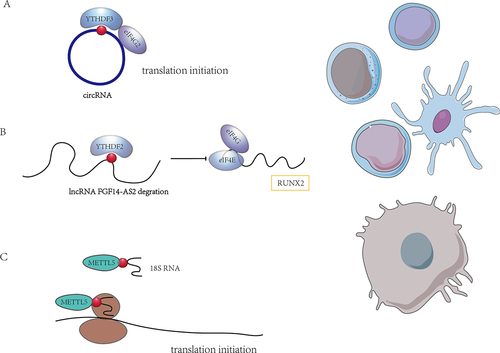
In addition to circRNAs lncRNAs are also under the influence of m6A regulation and engaged in translational regulation. LncRNA FGF14-AS2 was downregulated by YTHDF2-mediated RNA degradation in an m6A-dependent manner. FGF14-AS2 then inhibited the translation of RUNX2 by suppressing the assembly of the eIF4E/eIF4G complex and phosphorylation of eIF4E, thereby reducing the transcription of RANKL, an important regulator of osteoblast differentiation [Citation70] ().
Furthermore, METTL5-TRMT112 methyltransferase complex installed the m6A modification at position 1832 of human 18S rRNA and TRMT112 were required for METTL5 stability. In hepatocellular carcinoma (HCC) model, the depletion of METTL5-mediated 18S rRNA m6A modification leaded to impaired 80S ribosome assembly and reduced translation of mRNAs involved in fatty acid metabolism [Citation71] ().
Although current studies of m6A regulation of ncRNAs and its impact on translation are relatively limited, their role in immune cells has not been well attended to. The expression of lncRNA was highly cell- and stage-specific during T cell development and differentiation and lncRNAs played a critical role in the T-cell program [Citation72]. CircRNAs, with high stability, abundance and conservation, were involved in the remodelling of tumour immune microenvironment [Citation73]. CircRNAs also contributed to the activation of innate immunity in humans, where m6A RNA modification on human circRNAs suppressed innate immunity and exogenous circRNAs induced antigen-specific T cell activation, antibody production and anti-tumour immunity in vivo [Citation74]. Given the potential functions that non-coding RNAs in immune cells, it becomes crucial to future investigate the role of non-coding RNAs in RNA modification-dependent translational regulation. Such efforts will have significant implications for understanding the underlying mechanisms by which immune cells exert their roles.
m6A modifications and other RNA modifications
Prevalent modifications on mRNAs include m6A, N1-methyladenosine (m1A), 5-methylcytosine (m5C), 7-Methylguanosine (m7G), adenosine-to-inosine RNA editing, alternative polyadenylation and pseudouridine (Ψ) [Citation75,Citation76]. Most of these modifications contribute to pre-mRNA splicing, nuclear export, transcript stability and translation initiation in eukaryotic cells, constituting key mechanisms of epigenetic regulation in immune response and tumorigenesis [Citation75,Citation76]. We used an unsupervised clustering method to analyse the expression patterns of RNA modification ‘writers’ in colorectal cancer (CRC) samples and identified their functional interactions and potential roles in tumour microenvironment (TME), drug sensitivity, and immunotherapy [Citation77]. However, the mechanism of interaction between these RNA modifications remains unclear.
Certain RNA modification regulators have multiple identities that regulate different RNA modifications. For instance, FTO is known to bind multiple RNA species, including mRNA, snRNA and tRNA. FTO exhibits its catalytic activity on different substrates, including internal m6A and cap m6Am in mRNA, internal m6A in U6 RNA, internal and cap m6Am in snRNA, and m1A in tRNA demethylation [Citation35]. The distribution of FTO in different cell lines affected its access to distinct RNA substrates. It was also found that FTO had different effects on different substrates, with FTO-mediated demethylation having a greater effect on the transcript levels of mRNAs possessing internal m6A compared to mRNAs possessing cap m6Am. In addition, FTO could directly repress translation by catalysing m1A tRNA demethylation ().
Figure 4. The crosstalk between m6A modifications and other RNA modifications. (A) FTO plays a role in the demethylation of various RNA modifications, including m6A, m6Am and m1A. m1A-mediated tRNA modifications play an important role in the regulation of translation required for T cell activation, and the function of FTO in this is still unknown. (B) the binding protein YTHDF1 binds m1A in addition to m6A and thus mediates translation initiation. (C) in addition to binding m6A, YTHDC1 also has a weak RNA helicase function. There may be crosstalk between multiple RNA modifications, and how m6A regulators coordinate their functions in multiple RNA modifications and the roles they play in immune cells need to be further investigated.
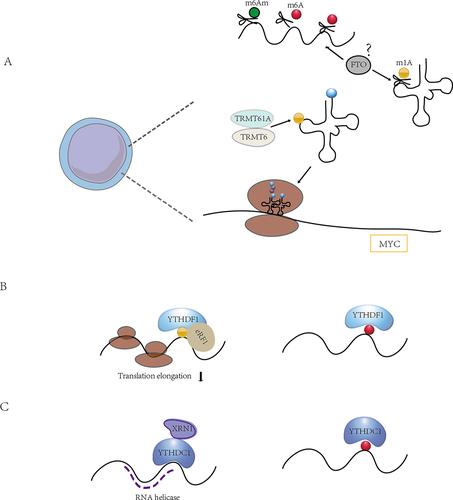
Xie at al. proposed that m1A-modified A71 on exon 1 of ATP5D (one of the most important subunits of adenosine-5’−triphosphate synthase) negatively regulated translation elongation in cancer cells via increasing binding to the YTHDF1/eRF1 complex and promoting the release of mRNA from the ribosomal complex, which in turn participates in glycolysis regulated by the m1A demethylase ALKBH3 [Citation78]. It is well established that m1A can regulate mRNA translation, and our recent research demonstrated that T cells upregulated tRNAs m1A58 methyltransferases TRMT61A and TRMT6 upon exit from quiescence, and that subsequent m1A-modified early tRNAs enhanced translation efficiency, ensuring rapid and necessary synthesis of MYC and a specific set of key functional proteins to guide naïve T cells from quiescence into a proliferative state and rapid expansion upon activation [Citation18]. It is still unknown whether m6A regulation of mRNA is involved in m1A-induced translational regulation in T cell differentiation and other physiological or pathological activities of immune cells ().
Furthermore, it has been revealed that YTHDC2 functions beyond binding m6A modifications. The YTH structural domain of YTHDC2 is an m6A binder in vitro, but mice with YTH point mutations are fertile, and loss of the weak 3‘→5’ RNA helicase activity leads to sterility. The weak helicase activity of YTHDC2 is enhanced by its interaction with the 5‘→3’ exonuclease XRN1 [Citation79]. CDS m6A positively regulated translation by resolving mRNA secondary structure, and the extended facilitation of CDS methylation required m6A reader YTHDC2, which containing the RNA helicase [Citation80]. Hu et al. also recognized the modified m6A sites tended to adopt predicted structures with lower minimum free energies (MFE) than non-methylated sites via m6A-SAC-seq, indicating that m6A tended to mark regions that form secondary structures, which in turn enhanced translation efficiency by relaxing secondary structure [Citation51] (). The interactions between RNA helicase activity and m6A binding domain during the m6A regulation of translation control require further investigation.
Conclusions and future perspectives
In recent years, rapid progress has been made in understanding the post-transcriptional regulation of genes by RNA modifications. RNA modification determines immune cell fate and regulates immune cell functional behaviour. m6A modification is involved in multiple pathways of mRNA metabolism, and translational regulation is essential for immune cells to rapidly respond to stimuli and adapt to the microenvironment. However, there are still more gaps in the study on the translational regulation of m6A in immune cells, and current studies are mostly focused on its role in regulating immune cell infiltration within the tumour microenvironment. In this review, we summarized current researches in the regulation of translation by m6A modifications with a view to uncovering possible pathways in the involvement of m6A modifications in the regulation of immune cell function through translational control. The m6A regulation of translation is mainly carried out through the recognition of m6A by YTHDF1, and whether other indirect mechanisms are involved is one of the questions that need to be addressed. Furthermore, other m6A binding proteins that can affect translation need to be identified and validated.
In addition, the subcellular localization of m6A regulators is also important. For example, METTL3 showed multiple functions that may depend on its cellular localization. m6A regulator expression and cellular localization were influenced by intracellular and extracellular environments. Exploring the effects of environmental stimuli or cellular signals on m6A regulators can offer insights into regulatory mechanisms underlying immune cells function in normal physiological states and pathological conditions.
There may be some indirect regulatory effects on the regulation of translation by m6A-related enzymes. METTL3 directly activated the PI3K-Akt-mTOR signalling pathway to promote retinoblastoma cancer cell proliferation, migration, and invasion, rather than being regulated by m6A methylation [Citation81]. Akt activated the mTORC1 pathway, therefore downstream activation of S6K1 phosphorylated ribosomal 40S protein S6, initiating the translation of the mRNA 5’-end and stimulating ribosomal protein synthesis [Citation82,Citation83]. The extension of ribosomes in the CDS region were affected by the sequence features, secondary structure of the CDS itself. Complex secondary structure, binding proteins on RNA affected ribosome migration, and thus translation speed. m6A binding protein YTHDC2, through its RNA helicase activity like clearing roadblocks to lift the secondary structure of RNA, promoted the smooth migration of ribosomes, which may ultimately be manifested as m6A-promoted translation. However, whether m6A is necessary for YTHDC2 to fulfill its roadblock-clearing function needs to be further clarified. In addition, most of the current studies have demonstrated translational regulation of m6A by analysing possible m6A-binding proteins that bind target genes, and by the fact that reader proteins have little effect on the mRNA levels of target genes, but do affect protein levels. This may be the result of some indirect regulatory effects and the mechanisms involved need to be further clarified.
Numerous non-coding RNAs exist in cells, especially the tRNAs and rRNAs, which perform vital post-transcriptional regulation of mRNAs. It is known that tRNAs and rRNAs are the most heavily decorated RNA species by various modifications, and the physiological function of those modification is far from understood. By moderating the fate and function of these non-coding RNAs, it may directly or indirectly affect the translation of mRNAs. Finally, a part of m6A regulators participate in regulating multiple modifications, and those RNA modifications they mediate have different regulatory functions and mechanisms in mRNA translation. The combined or crosstalk effect among these RNA modifications may affect the specificity of their target mRNAs, thereby generating different fates of immune cells.
TCR signalling controls the development, activation and survival of T cells by involving several layers and numerous mechanisms of gene regulation. Signals from the newly rearranged determine fate of thymocytes during T cell development and TCR repertoire selection [Citation84]. Peripheral mature T cells exert effector functions after antigen recognition. TCR stimulation of effector T cells can cause activation-induced cell death (AICD), and AICD is crucial for maintenance of peripheral T cell homoeostasis after antigen clearance [Citation85]. Although it has been reported that m6A is involved in AICD by influencing mRNA decay, the contribution of m6A-dependent translational regulation in this has not been reported.
m6A modifications are only observed in a specific subset of mRNAs and can be bound by different reading proteins, so each cell type may establish unique regulatory networks leading to different outcomes. In analysing the role of m6A in translational regulation, it is essential to consider the specificity of m6A modification sites and targets, and it is necessary to clarify how different m6A reading proteins and other RNA-binding proteins act synergistically or antagonistically in different specific contexts. Therefore, it is relevant to characterize the function of immune cells in homoeostatic and disease conditions through the investigation of RNA modification regulators, including m6A modification, and to excavate the complex role exerted by RNA regulatory proteins in regulating mRNA translation. This will provide theoretical support for the development of epigenetic therapies for the treatment of autoimmune diseases, infectious diseases and cancer from the perspective of RNA modifications.
Author contributions
YJ.Z. and H-B.L. conceived the chapter. YJ.Z. wrote the manuscript. WG.H. and H-B.L. revised the manuscript.
Acknowledgments
We would like to thank all members of the H-B. L laboratory for discussion. We apologize to all scientists whose valuable contributions could not be cited in this review due to limitations of volume.
Disclosure statement
No potential conflict of interest was reported by the authors.
Data availability statement
Data sharing is not applicable to this article as no new data were created or analysed in this study.
Additional information
Funding
References
- Maniatis T, Reed R. An extensive network of coupling among gene expression machines. Nature. 2002;416(6880):499–506. doi: 10.1038/416499a
- Nouaille S, Mondeil S, Finoux AL, et al. The stability of an mRNA is influenced by its concentration: a potential physical mechanism to regulate gene expression. Nucleic Acids Res. 2017;45(20):11711–11724. doi: 10.1093/nar/gkx781
- Desrosiers R, Friderici K, Rottman F. Identification of methylated nucleosides in messenger RNA from Novikoff hepatoma cells. Proc Natl Acad Sci U S A. 1974;71(10):3971–3975. doi: 10.1073/pnas.71.10.3971
- Meyer KD, Saletore Y, Zumbo P, et al. Comprehensive analysis of mRNA methylation reveals enrichment in 3′ UTRs and near stop codons. Cell. 2012;149(7):1635–1646. doi: 10.1016/j.cell.2012.05.003
- Meyer KD, Patil DP, Zhou J, et al. 5′ UTR m6A promotes cap-independent translation. Cell. 2015;163(4):999–1010. doi: 10.1016/j.cell.2015.10.012
- Roignant JY, Soller M. m(6)A in mRNA: an ancient mechanism for fine-tuning gene expression. Trends Genet. 2017;33(6):380–390. doi: 10.1016/j.tig.2017.04.003
- Ries RJ, Zaccara S, Klein P, et al. m(6)A enhances the phase separation potential of mRNA. Nature. 2019;571(7765):424–428. doi: 10.1038/s41586-019-1374-1
- Tong J, Flavell RA, Li HB. RNA m(6)A modification and its function in diseases. Front Med. 2018;12(4):481–489. doi: 10.1007/s11684-018-0654-8
- Chao Y, Li HB, Zhou J. Multiple functions of RNA methylation in T cells: a review. Front Immunol. 2021;12:627455. doi: 10.3389/fimmu.2021.627455
- Li HB, Tong J, Zhu S, et al. m(6)A mRNA methylation controls T cell homeostasis by targeting the IL-7/STAT5/SOCS pathways. Nature. 2017;548(7667):338–342. doi: 10.1038/nature23450
- Tong J, Cao G, Zhang T, et al. m(6)A mRNA methylation sustains Treg suppressive functions. Cell Res. 2018;28(2):253–256. doi: 10.1038/cr.2018.7
- Ito-Kureha T, Leoni C, Borland K, et al. The function of Wtap in N(6)-adenosine methylation of mRnas controls T cell receptor signaling and survival of T cells. Nat Immunol. 2022;23(8):1208–1221. doi: 10.1038/s41590-022-01268-1
- Murakami S, Jaffrey SR. Hidden codes in mRNA: control of gene expression by m(6)A. Mol Cell. 2022;82(12):2236–2251. doi: 10.1016/j.molcel.2022.05.029
- Shi H, Wei J, Where HC. When, and how: context-dependent functions of RNA methylation writers, readers, and erasers. Mol Cell. 2019;74(4):640–650. doi: 10.1016/j.molcel.2019.04.025
- Kafasla P, Skliris A, Kontoyiannis DL. Post-transcriptional coordination of immunological responses by RNA-binding proteins. Nat Immunol. 2014;15(6):492–502. doi: 10.1038/ni.2884
- Piccirillo CA, Bjur E, Topisirovic I, et al. Translational control of immune responses: from transcripts to translatomes. Nat Immunol. 2014;15(6):503–511. doi: 10.1038/ni.2891
- Wolf T, Jin W, Zoppi G, et al. Dynamics in protein translation sustaining T cell preparedness. Nat Immunol. 2020;21(8):927–937. doi: 10.1038/s41590-020-0714-5
- Liu Y, Zhou J, Li X, et al. tRNA-m(1)A modification promotes T cell expansion via efficient MYC protein synthesis. Nat Immunol. 2022;23(10):1433–1444. doi: 10.1038/s41590-022-01301-3
- Boo SH, Ha H, Kim YK. m(1)A and m(6)A modifications function cooperatively to facilitate rapid mRNA degradation. Cell Rep. 2022;40(10):111317. doi: 10.1016/j.celrep.2022.111317
- Bokar JA, Rath-Shambaugh ME, Ludwiczak R, et al. Characterization and partial purification of mRNA N6-adenosine methyltransferase from HeLa cell nuclei. Internal mRNA methylation requires a multisubunit complex. J Biol Chem. 1994;269(26):17697–17704. doi: 10.1016/S0021-9258(17)32497-3
- Bokar JA, Shambaugh ME, Polayes D, et al. Purification and cDNA cloning of the AdoMet-binding subunit of the human mRNA (N6-adenosine)-methyltransferase. RNA. 1997;3(11):1233–1247.
- Wang P, Doxtader KA, Nam Y. Structural basis for cooperative function of Mettl3 and Mettl14 methyltransferases. Molecular Cell. 2016;63(2):306–317. doi: 10.1016/j.molcel.2016.05.041
- Liu J, Yue Y, Han D, et al. A METTL3–METTL14 complex mediates mammalian nuclear RNA N6-adenosine methylation. Nat Chem Biol. 2014;10(2):93–95. doi: 10.1038/nchembio.1432
- Weng H, Huang H, Wu H, et al. METTL14 inhibits Hematopoietic stem/Progenitor differentiation and promotes leukemogenesis via mRNA m(6)A modification. Cell Stem Cell. 2018;22(2):191–205 e9. doi: 10.1016/j.stem.2017.11.016
- Wang X, Feng J, Xue Y, et al. Correction: corrigendum: structural basis of N6-adenosine methylation by the METTL3–METTL14 complex. Nature. 2017;542(7640):260. doi: 10.1038/nature21073
- Ping XL, Sun BF, Wang L, et al. Mammalian WTAP is a regulatory subunit of the RNA N6-methyladenosine methyltransferase. Cell Res. 2014;24(2):177–189. doi: 10.1038/cr.2014.3
- Wen J, Lv R, Ma H, et al. Zc3h13 regulates nuclear RNA m(6)A methylation and mouse embryonic stem cell self-renewal. Mol Cell. 2018;69(6):1028–1038 e6. doi: 10.1016/j.molcel.2018.02.015
- van Tran N, Ernst FGM, Hawley BR, et al. The human 18S rRNA m6A methyltransferase METTL5 is stabilized by TRMT112. Nucleic Acids Res. 2019;47(15):7719–7733. doi: 10.1093/nar/gkz619
- Pinto R, Vagbo CB, Jakobsson ME, et al. The human methyltransferase ZCCHC4 catalyses N6-methyladenosine modification of 28S ribosomal RNA. Nucleic Acids Res. 2020;48(2):830–846. doi: 10.1093/nar/gkz1147
- Patil DP, Chen CK, Pickering BF, et al. m(6)A RNA methylation promotes XIST-mediated transcriptional repression. Nature. 2016;537(7620):369–373. doi: 10.1038/nature19342
- Pendleton KE, Chen B, Liu K, et al. The U6 snRNA m(6)A methyltransferase METTL16 regulates SAM synthetase intron retention. Cell. 2017;169(5):824–835 e14. doi: 10.1016/j.cell.2017.05.003
- Brown JA, Kinzig CG, DeGregorio SJ, et al. Methyltransferase-like protein 16 binds the 3 ’-terminal triple helix of MALAT1 long noncoding RNA. Proceedings of the National Academy of Sciences of the United States of America. 2016;113:14013–14018. doi: 10.1073/pnas.1614759113
- Jia GF, Fu Y, Zhao X, et al. N6-methyladenosine in nuclear RNA is a major substrate of the obesity-associated FTO. Nat Chem Biol. 2011;7(12):885–887. doi: 10.1038/nchembio.687
- Mauer J, Sindelar M, Despic V, et al. FTO controls reversible m(6)Am RNA methylation during snRNA biogenesis. Nat Chem Biol. 2019;15(4):340–347. doi: 10.1038/s41589-019-0231-8
- Wei J, Liu F, Lu Z, et al. Differential m(6)A, m(6)A(m), and m(1)A demethylation mediated by FTO in the cell nucleus and cytoplasm. Mol Cell. 2018;71(6):973–985 e5. doi: 10.1016/j.molcel.2018.08.011
- Tang C, Klukovich R, Peng H, et al. ALKBH5-dependent m6A demethylation controls splicing and stability of long 3′-UTR mRnas in male germ cells. Proc Natl Acad Sci U S A. 2018;115(2):E325–E333. doi: 10.1073/pnas.1717794115
- Wang X, Zhao BS, Roundtree IA, et al. N-6-methyladenosine modulates messenger RNA translation efficiency. Cell. 2015;161(6):1388–1399. doi: 10.1016/j.cell.2015.05.014
- Du H, Zhao Y, He J, et al. YTHDF2 destabilizes m6A-containing RNA through direct recruitment of the CCR4–NOT deadenylase complex. Nat Commun. 2016;7(1):12626. doi: 10.1038/ncomms12626
- Jin D, Guo J, Wu Y, et al. m6A demethylase ALKBH5 inhibits tumor growth and metastasis by reducing YTHDFs-mediated YAP expression and inhibiting miR-107/LATS2–mediated YAP activity in NSCLC. Mol Cancer. 2020;19(1):40. doi: 10.1186/s12943-020-01161-1
- Wang H, Hu X, Huang M, et al. Mettl3-mediated mRNA m(6)A methylation promotes dendritic cell activation. Nat Commun. 2019;10(1):1898. doi: 10.1038/s41467-019-09903-6
- Han D, Liu J, Chen C, et al. Anti-tumour immunity controlled through mRNA m(6)A methylation and YTHDF1 in dendritic cells. Nature. 2019;566(7743):270–274. doi: 10.1038/s41586-019-0916-x
- Li T, Tan YT, Chen YX, et al. Methionine deficiency facilitates antitumour immunity by altering m 6 a methylation of immune checkpoint transcripts. Gut. 2023;72(3):501–511. doi: 10.1136/gutjnl-2022-326928
- Xiong J, He J, Zhu J, et al. Lactylation-driven METTL3-mediated RNA m(6)A modification promotes immunosuppression of tumor-infiltrating myeloid cells. Mol Cell. 2022;82(9):1660–1677 e10. doi: 10.1016/j.molcel.2022.02.033
- Yin H, Zhang X, Yang P, et al. RNA m6A methylation orchestrates cancer growth and metastasis via macrophage reprogramming. Nat Commun. 2021;12(1):1394. doi: 10.1038/s41467-021-21514-8
- Hao WC, Dian MJ, Zhou Y, et al. Autophagy induction promoted by m(6)A reader YTHDF3 through translation upregulation of FOXO3 mRNA. Nat Commun. 2022;13(1): doi: 10.1038/s41467-022-32963-0
- Stienne C, Michieletto MF, Benamar M, et al. Foxo3 transcription factor Drives Pathogenic T Helper 1 differentiation by inducing the expression of Eomes. Immunity. 2016;45(4):774–787. doi: 10.1016/j.immuni.2016.09.010
- Wu G, Suo C, Yang Y, et al. MYC promotes cancer progression by modulating m 6 a modifications to suppress target gene translation. EMBO Rep. 2021;22(3):e51519. doi: 10.15252/embr.202051519
- Zaccara S, Jaffrey SR. A unified model for the function of YTHDF proteins in regulating m(6)A-Modified mRNA. Cell. 2020;181(7):1582–1595 e18. doi: 10.1016/j.cell.2020.05.012
- Lee AS, Kranzusch PJ, Cate JH. eIF3 targets cell-proliferation messenger RNAs for translational activation or repression. Nature. 2015;522(7554):111–114. doi: 10.1038/nature14267
- Zou Z, Sepich-Poore C, Zhou X, et al. The mechanism underlying redundant functions of the YTHDF proteins. Genome Biol. 2023;24(1):17. doi: 10.1186/s13059-023-02862-8
- Hu L, Liu S, Peng Y, et al. m(6)A RNA modifications are measured at single-base resolution across the mammalian transcriptome. Nat Biotechnol. 2022;40(8):1210–1219. doi: 10.1038/s41587-022-01243-z
- Huang H, Weng H, Sun W, et al. Recognition of RNA N(6)-methyladenosine by IGF2BP proteins enhances mRNA stability and translation. Nat Cell Biol. 2018;20(3):285–295. doi: 10.1038/s41556-018-0045-z
- Bechara R, Amatya N, Bailey RD, et al. The m 6 a reader IMP2 directs autoimmune inflammation through an IL-17– and TNFα-dependent C/EBP transcription factor axis. Sci Immunol. 2021;6(61):6. doi: 10.1126/sciimmunol.abd1287
- Weng H, Huang F, Yu Z, et al. The m(6)A reader IGF2BP2 regulates glutamine metabolism and represents a therapeutic target in acute myeloid leukemia. Cancer Cell. 2022;40(12):1566–1582 e10. doi: 10.1016/j.ccell.2022.10.004
- Karinch AM, Pan M, Lin CM, et al. Glutamine metabolism in sepsis and infection. J Nutr. 2001;131(9):2535S–2538S. discussion 2550S-1S. doi: 10.1093/jn/131.9.2535S
- Liu PS, Wang H, Li X, et al. α-ketoglutarate orchestrates macrophage activation through metabolic and epigenetic reprogramming. Nat Immunol. 2017;18(9):985–994. doi: 10.1038/ni.3796
- Li R, Zeng L, Zhao H, et al. ATXN2-mediated translation of TNFR1 promotes esophageal squamous cell carcinoma via m(6)A-dependent manner. Mol Ther. 2022;30(3):1089–1103. doi: 10.1016/j.ymthe.2022.01.006
- Ralser M, Albrecht M, Nonhoff U, et al. An integrative approach to gain insights into the cellular function of human ataxin-2. J Mol Biol. 2005;346(1):203–214. doi: 10.1016/j.jmb.2004.11.024
- Kozlov G, Safaee N, Rosenauer A, et al. Structural basis of binding of P-body-associated proteins GW182 and ataxin-2 by the Mlle domain of poly(A)-binding protein. J Biol Chem. 2010;285(18):13599–13606. doi: 10.1074/jbc.M109.089540
- Zhang G, Xu Y, Wang X, et al. Dynamic FMR1 granule phase switch instructed by m6A modification contributes to maternal RNA decay. Nat Commun. 2022;13(1):859. doi: 10.1038/s41467-022-28547-7
- Yildirim Z, Baboo S, Hamid SM, et al. Intercepting IRE1 kinase-FMRP signaling prevents atherosclerosis progression. EMBO Mol Med. 2022;14(4):e15344. doi: 10.15252/emmm.202115344
- Lin S, Choe J, Du P, et al. The m(6)A methyltransferase METTL3 promotes translation in human cancer cells. Mol Cell. 2016;62(3):335–345. doi: 10.1016/j.molcel.2016.03.021
- Choe J, Lin S, Zhang W, et al. mRNA circularization by METTL3–eIf3h enhances translation and promotes oncogenesis. Nature. 2018;561(7724):556–560. doi: 10.1038/s41586-018-0538-8
- Wei X, Huo Y, Pi J, et al. METTL3 preferentially enhances non-m(6)A translation of epigenetic factors and promotes tumourigenesis. Nat Cell Biol. 2022;24(8):1278–1290. doi: 10.1038/s41556-022-00968-y
- Li Y, He X, Lu X, et al. METTL3 acetylation impedes cancer metastasis via fine-tuning its nuclear and cytosolic functions. Nat Commun. 2022;13(1):6350. doi: 10.1038/s41467-022-34209-5
- Chen J, Wei X, Wang X, et al. TBK1-METTL3 axis facilitates antiviral immunity. Cell Rep. 2022;38(7):110373. doi: 10.1016/j.celrep.2022.110373
- Gong DK, Zhang J, Chen YH, et al. The m(6)A-suppressed P2RX6 activation promotes renal cancer cells migration and invasion through ATP-induced Ca2+ influx modulating ERK1/2 phosphorylation and MMP9 signaling pathway. J Exp Clin Cancer Res. 2019;38(1): doi: 10.1186/s13046-019-1223-y
- Su R, Dong L, Li YC, et al. METTL16 exerts an m(6)A-independent function to facilitate translation and tumorigenesis. Nat Cell Biol. 2022;24(2):205–216. doi: 10.1038/s41556-021-00835-2
- Yang Y, Fan X, Mao M, et al. Extensive translation of circular RNAs driven by N(6)-methyladenosine. Cell Res. 2017;27(5):626–641. doi: 10.1038/cr.2017.31
- Zhang M, Wang J, Jin Y, et al. YTHDF2-mediated FGF14-AS2 decay promotes osteolytic metastasis of breast cancer by enhancing RUNX2 mRNA translation. Br J Cancer. 2022;127(12):2141–2153. doi: 10.1038/s41416-022-02006-y
- Peng H, Chen B, Wei W, et al. N(6)-methyladenosine (m(6)A) in 18S rRNA promotes fatty acid metabolism and oncogenic transformation. Nat Metab. 2022;4(8):1041–1054. doi: 10.1038/s42255-022-00622-9
- Zhang Y, Li B, Bai Q, et al. The lncRNA Snhg1-Vps13D vesicle trafficking system promotes memory CD8 T cell establishment via regulating the dual effects of IL-7 signaling. Signal Transduct Target Ther. 2021;6(1):126. doi: 10.1038/s41392-021-00492-9
- Pan Z, Zhao R, Li B, et al. EWSR1-induced circNEIL3 promotes glioma progression and exosome-mediated macrophage immunosuppressive polarization via stabilizing IGF2BP3. Mol Cancer. 2022;21(1):16. doi: 10.1186/s12943-021-01485-6
- Chen YG, Chen R, Ahmad S, et al. N6-methyladenosine modification controls circular RNA immunity. Mol Cell. 2019;76(1):96–109 e9. doi: 10.1016/j.molcel.2019.07.016
- Wiener D, Schwartz S. The epitranscriptome beyond m(6)A. Nat Rev Genet. 2021;22(2):119–131. doi: 10.1038/s41576-020-00295-8
- Barbieri I, Kouzarides T. Role of RNA modifications in cancer. Nat Rev Cancer. 2020;20(6):303–322. doi: 10.1038/s41568-020-0253-2
- Chen H, Yao J, Bao R, et al. Cross-talk of four types of RNA modification writers defines tumor microenvironment and pharmacogenomic landscape in colorectal cancer. Mol Cancer. 2021;20(1):29. doi: 10.1186/s12943-021-01322-w
- Wu Y, Chen Z, Xie G, et al. RNA m 1 a methylation regulates glycolysis of cancer cells through modulating ATP5D. Proc Natl Acad Sci U S A. 2022;119(28):e2119038119. doi: 10.1073/pnas.2119038119
- Li L, Krasnykov K, Homolka D, et al. The XRN1-regulated RNA helicase activity of YTHDC2 ensures mouse fertility independently of m(6)A recognition. Mol Cell. 2022;82(9):1678–1690 e12. doi: 10.1016/j.molcel.2022.02.034
- Mao Y, Dong L, Liu XM, et al. m(6)A in mRNA coding regions promotes translation via the RNA helicase-containing YTHDC2. Nat Commun. 2019;10(1):5332. doi: 10.1038/s41467-019-13317-9
- Zhang H, Zhang P, Long C, et al. M 6 a methyltransferase METTL3 promotes retinoblastoma progression via PI3K/AKT/mTOR pathway. J Cell Mol Med. 2020;24(21):12368–12378. doi: 10.1111/jcmm.15736
- Magnuson B, Ekim B, Fingar DC. Regulation and function of ribosomal protein S6 kinase (S6K) within mTOR signalling networks. Biochem J. 2012;441(1):1–21. doi: 10.1042/BJ20110892
- Kimball SR, Shantz LM, Horetsky RL, et al. Leucine regulates translation of specific mRnas in L6 myoblasts through mTOR-mediated changes in availability of eIF4E and phosphorylation of ribosomal protein S6. J Biol Chem. 1999;274(17):11647–11652. doi: 10.1074/jbc.274.17.11647
- Daley SR, Teh C, Hu DY, et al. Cell death and thymic tolerance. Immunol Rev. 2017;277(1):9–20. doi: 10.1111/imr.12532
- Zhan Y, Carrington EM, Zhang Y, et al. Life and death of activated T cells: how are they different from naive T cells? Front Immunol. 2017;8:1809. doi: 10.3389/fimmu.2017.01809