ABSTRACT
Small Nucleolar RNAs (snoRNAs) are an abundant group of non-coding RNAs with well-defined roles in ribosomal RNA processing, folding and chemical modification. Besides their classic roles in ribosome biogenesis, snoRNAs are also implicated in several other cellular activities including regulation of splicing, transcription, RNA editing, cellular trafficking, and miRNA-like functions. Mature snoRNAs must undergo a series of processing steps tightly regulated by transiently associating factors and coordinated with other cellular processes including transcription and splicing. In addition to their mature forms, snoRNAs can contribute to gene expression regulation through their derivatives and degradation products. Here, we review the current knowledge on mechanisms of snoRNA maturation, including the different pathways of processing, and the regulatory mechanisms that control snoRNA levels and complex assembly. We also discuss the significance of studying snoRNA maturation, highlight the gaps in the current knowledge and suggest directions for future research in this area.
Introduction
Small nucleolar RNAs (snoRNAs) are a well-conserved, highly abundant class of non-coding RNAs predominantly localized in the nucleolus. They typically range from 60 to 300 nucleotides in length and are divided into two major families based on their conserved structural motifs: box H/ACA snoRNAs and box C/D snoRNAs [Citation1] (). A class of related snoRNAs, small Cajal Body (CB)-specific RNAs (scaRNAs) can resemble the structures of box C/D snoRNAs or box H/ACA snoRNAs or take on a composite structure of both. scaRNAs differ from C/D snoRNAs and H/ACA snoRNAs in their conserved motifs and sub-nuclear localization [Citation2–5]. The different classes of snoRNAs interact with distinct sets of core proteins forming ribonucleoprotein (RNP) complexes. The snoRNP complexes are best known for guiding modifications through complementary base-pairing interactions with target RNAs [Citation6]. In addition, some snoRNAs play critical roles in guiding RNA processing, cleavage and folding events [Citation7–10], and others have been implicated in the regulation of mRNA expression [Citation11,Citation12]. While ribosomal RNAs (rRNAs) account for the majority of known snoRNA binding substrates, scaRNAs predominantly target small nuclear RNAs (snRNAs) [Citation13–15]. In archaea, box C/D and H/ACA small RNAs can guide the modification of both rRNAs and tRNAs [Citation16–18]. The human elongator tRNAMet was recently shown to be targeted at a specific site for modification by a nucleolar and a CB-localized guide RNA, providing the first example of a eukaryotic RNA-guided tRNA modification [Citation9]. In mammalian cells, snoRNAs are also reported to impact the modification or stability of select mRNAs, although the RNP requirements for such snoRNA activities and the molecular mechanisms remain unclear [Citation19–21]. While computational approaches have allowed the identification of potential snoRNA substrates, several snoRNAs in mammalian cells lack identified or experimentally validated binding targets and are classified as orphans [Citation14,Citation17].
Figure 1. Schematic of snoRNP complexes. A) left, box C/D snoRNA (red) bound by core proteins (shades of orange). Middle, conserved box C/D and C’/D’ motifs are shown. Nop1 (human FBL) transfers a methyl group to the ribose moiety of the nucleotide that is base paired to the guide region, at a site five nucleotides upstream of the D or D’ box. Right, Nop1 catalyzes the addition of a methyl group (red) onto the 2’-hydroxyl of a ribose sugar. B) Left, a C/D snoRNA bound by Kre33 (human NAT10) in light blue. C/D snoRNA-bound Kre33 can acetylate target RNAs. Middle, base pairing of guide RNA for acetylation is different from perfect complementary binding to the guide region that is required for 2’-O-methylation by Nop1. Right, cytidine base acetylation. Acetylation is highlighted in red. This cartoon figure was generated based on information described in reference [Citation22]. C) Left, box H/ACA snoRNA (red) bound by core proteins (in shades of orange). Middle, conserved box H/ACA motifs and target RNA base pairing is shown. Cbf5 (human DKC1) isomerizes uridine to pseudouridine. Right, pseudouridylation of Uridine. Isomerization is highlighted in red.
![Figure 1. Schematic of snoRNP complexes. A) left, box C/D snoRNA (red) bound by core proteins (shades of orange). Middle, conserved box C/D and C’/D’ motifs are shown. Nop1 (human FBL) transfers a methyl group to the ribose moiety of the nucleotide that is base paired to the guide region, at a site five nucleotides upstream of the D or D’ box. Right, Nop1 catalyzes the addition of a methyl group (red) onto the 2’-hydroxyl of a ribose sugar. B) Left, a C/D snoRNA bound by Kre33 (human NAT10) in light blue. C/D snoRNA-bound Kre33 can acetylate target RNAs. Middle, base pairing of guide RNA for acetylation is different from perfect complementary binding to the guide region that is required for 2’-O-methylation by Nop1. Right, cytidine base acetylation. Acetylation is highlighted in red. This cartoon figure was generated based on information described in reference [Citation22]. C) Left, box H/ACA snoRNA (red) bound by core proteins (in shades of orange). Middle, conserved box H/ACA motifs and target RNA base pairing is shown. Cbf5 (human DKC1) isomerizes uridine to pseudouridine. Right, pseudouridylation of Uridine. Isomerization is highlighted in red.](/cms/asset/0bd5fe17-02bf-4c1f-ba7e-1caa37cf39a2/krnb_a_2254540_f0001_oc.jpg)
Box C/D snoRNAs contain two conserved motifs, the box C (RUGAUGA) and box D (CUGA) motifs, which interact and form the characteristic ‘kink-turn’ (K-turn), as well as two less conserved regions, the box C’ and box D’ motifs, which can adopt a second K-turn. The C/D and C’/D’ motifs are connected by guide regions that can have sequence-specific complementarity to target RNAs [Citation18,Citation23] (). C/D snoRNPs form by binding of a box C/D snoRNA to four conserved essential proteins including FBL (yeast Nop1), NOP56 (yeast Nop56), NOP58 (yeast Nop58), and SNU13 (also referred to as 15.5K; yeast Snu13). Targeting 2’-O-methylation of substrate RNAs is based on RNA-snoRNA base pairing in the guide region of snoRNA and occurs at the 5th nucleotide upstream of the D or D’ motifs. In yeast, the majority of snoRNA-guided rRNA 2’-O-methylations are deposited co-transcriptionally [Citation24]. In the C/D snoRNP complex, Snu13 recognizes the K-turn(s) and Nop56 and Nop58 dimerize via their coiled-coil domains to form a pseudosymmetric complex [Citation25]. Nop1/FBL is the methyltransferase responsible for transferring a methyl group to the 2’-hydroxyl of the ribose moiety of the substrate RNA [Citation26]. More recently, it was shown that select box C/D snoRNAs also function as guides for rRNA acetylation in complex with acetyltransferase NAT10 (Kre33 in yeast; ) [Citation22,Citation27,Citation28]. Although NAT10/Kre33 binds to box C/D snoRNAs, the mechanism of acetylation is more similar to that of pseudouridylation by box H/ACA snoRNAs, as the target nucleotide is free from the snoRNP, leaving it available for modification by NAT10/Kre33 [Citation22].
Box H/ACA snoRNAs form a hairpin-hinge-hairpin-tail structure and have two conserved motifs, the box H (ANANNA; where N can be any nucleotide) and box ACA motifs within the hinge and tail regions, respectively () [Citation29–32]. Upstream of each hinge and tail region, there are two pseudouridylation loops, which have sequence complementarity to target RNA. Box H/ACA snoRNAs bind to four evolutionarily conserved proteins to form RNP complexes that can modify the target RNA. The core H/ACA snoRNP proteins include DKC1 (yeast Cbf5), GAR1 (yeast Gar1), NOP10 (yeast Nop10) and NHP2 (yeast Nhp2), all of which are required for the optimal enzymatic activity of the snoRNP complex [Citation18,Citation32,Citation33]. In this complex, Nhp2 and the ACA motif facilitate the correct positioning of the guide RNA for modification of the target nucleotide by Cbf5 [Citation34]. Cbf5 on one side interacts with Nop10 which links it to Nhp2 and on the other side reaches Gar1, which facilitates substrate binding, modification, and release after pseudouridylation [Citation35–40]. The telomerase complex in vertebrates, responsible for generating telomeric repeats at the ends of chromosomes, contains an H/ACA RNP. Similar to eukaryotic H/ACA RNPs, the H/ACA domain of telomerase contains two RNA hairpins with an H box within the hinge and the ACA motif at the 3´ tail. Each RNA hairpin binds to the tetrameric complex of DKC1, GAR1, NOP10 and NHP2. Recent structural work has elucidated the high-resolution structure of the human telomerase RNP, revealing an asymmetric complex in which the H and ACA boxes are brought together by the two DKC1 molecules in the RNP [Citation41].
scaRNAs are typically found in CBs, which are subnuclear structures involved in the biogenesis of small nuclear ribonucleoproteins (snRNPs) and processing of other RNAs. scaRNAs guide most of the known modifications of snRNAs [Citation13] and fall into two main types: C/D-box scaRNAs and H/ACA-box scaRNAs. However, hybrid scaRNAs containing features of both C/D and H/ACA snoRNAs have also been identified [Citation2,Citation42]. Besides the C/D or H/ACA structural elements, scaRNAs have the CB localization elements (CAB box) and the G•U/U•G stem [Citation3,Citation43]. In addition to their RNA structural elements, the binding of the CAB box of scaRNAs with WD40 repeat-containing protein WDR79 (also referred to as TCAB1 [Citation44] and WRAP53 [Citation45]) has been shown to be essential for targeting scaRNAs to CBs [Citation46,Citation47].
In this review, we will focus on different aspects of the production of snoRNAs and the roles of snoRNA-associated factors in the transcription, assembly, processing and regulation of snoRNAs in yeast and humans. Our review primarily focuses on snoRNA maturation in the model organism Saccharomyces cerevisiae, which is the best-studied model in this regard and draws comparisons to human cells when relevant.
Genomic loci and organization of snoRNAs
The genomic organization of snoRNAs is species-dependent [Citation48,Citation49]. However, there are two predominant organization patterns across all organisms: snoRNAs are either encoded in independent transcription units or are encoded within the intron of protein-coding or non-coding genes (see ) [Citation49,Citation50]. In yeast, there are 64 snoRNA transcription units, which generate 76 snoRNAs including 47 box C/D and 29 box H/ACA snoRNAs [Citation49]. Most yeast snoRNAs are encoded in independent transcription units, with about 20% encoded by polycistronic genes and only 11% present in introns [Citation51]. This genomic organization differs dramatically from human snoRNAs, where most snoRNAs are in the introns of genes except for U3, U8, U13 and telomerase snoRNA [Citation49]. Within introns or transcription units, snoRNAs can exist as monocistronic genes or cluster together in polycistronic units.
Figure 2. Maturation of box C/D snoRNAs. A) splicing-dependent C/D snoRNA processing: snoRNAs are spliced out of their host transcripts by the spliceosome. This generates intron lariats that require debranching by Dbr1 (human DBR1). After debranching, the premature snoRNAs undergo 5’ trimming by exonucleases Rat1/Xrn1 (human XRN2/XRN1) and 3’ trimming by the nuclear exosome. Yeast TRAMP complex places short oligo-A tails at the 3’ ends of the snoRNAs for exonucleolytic cleavage and recruits RNA exosome to pre-snoRNAs. In humans, NEXT complex recruits RNA exosome to pre-snoRNAs adenylated by PAPD5. Trimming of the last few 3’-tail nucleotides requires the action of Rrp6 in yeast. Poly(A) specific nucleases PARN and TOE1 are involved in removal of 3’-tail in humans. B) splicing-independent C/D snoRNA processing: snoRNAs are released from the host transcript through endonucleolytic cleavage. In yeast, this endonuclease is Rnt1. Pre-snoRNAs then undergo 5’ and 3’ processing as described in section A. C) independently transcribed monocistronic C/D snoRNAs: These snoRNAs are capped and their cap is co-transcriptionally removed by endonuclease Rnt1 for 5’ exonucleolytic trimming. The 3’-end processing of these RNAs in yeast requires the activity of TRAMP, RNA exosome and Rrp6. D) independently transcribed polycistronic C/D snoRNAs: These snoRNAs are generated similarly to those described in section C but are cleaved between each snoRNA by Rnt1. E) assembly of C/D snoRNPs: Assembly factors chaperone the binding of core proteins and prevent premature catalytic activity of the complex. The HSP90•R2TP complex (human HSP90, RUVBL1/2, PIH1D and RPAP3) serves as a platform for assembly factors and core proteins to form the mature snoRNP. SNU13, NUFIP1 and ZNHIT3 form a heterotrimeric complex and interact with NOP58 through R2TP•ZNHIT6 binding. Association of the snoRNA-bound complex with NOP56 and FBL and loss of R2TP•ZNHIT6•ZNHIT3 lead to formation of the mature snoRNP. Processing factors are in shades of green, assembly factors in shades of blue and snoRNP core proteins in shades of orange. To simplify, the crosstalk between transcription, processing and assembly steps is not depicted here.
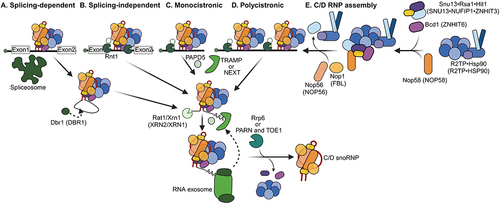
Figure 3. Maturation of box H/ACA snoRNAs. A) splicing-dependent H/ACA snoRNA processing: snoRNAs are spliced out of their host transcripts by the spliceosome. This generates intron lariats that require debranching by Dbr1 (human DBR1). After debranching, the premature snoRNAs undergo 5’ trimming by exonucleases Rat1/Xrn1 (human XRN2/XRN1) and 3’ trimming by the nuclear exosome. Yeast TRAMP complex places short oligo-A tails at the 3’ ends of the snoRNAs for exonucleolytic cleavage and recruits RNA exosome to pre-snoRNAs. In humans, NEXT complex recruits RNA exosome to pre-snoRNAs adenylated by PAPD5. Trimming of the last few 3’-tail nucleotides requires the action of Rrp6 in yeast. Poly(A) specific nucleases PARN and TOE1 are involved in removal of 3’-tail in humans. B) splicing-independent H/ACA snoRNA processing: These snoRNAs are released from their host transcript through endonucleolytic cleavage. In yeast, this endonuclease is Rnt1. They then undergo 5’ and 3’ processing similar to (A). C) independently transcribed monocistronic H/ACA snoRNAs: these snoRNAs are released from the host transcript by transcription termination and their m7G caps are subsequently hypermodified to trimethylguanosine (TMG) by Tgs1 (human TGS1). D) independently transcribed polycistronic H/ACA snoRNAs: these snoRNAs are generated as described in C but are cleaved between each snoRNA by Rnt1 at specific Rnt1 cleavage sites. Capped snoRNAs undergo hypermodification by Tgs1 to TMG. E) assembly of box H/ACA snoRNPs: SHQ1 is a critical chaperone for DKC1 that binds to DKC1 early in assembly. NUFIP1 binds NHP2 directly and is present in a pentameric complex of DKC1, SHQ1, NAF1, NOP10 and NHP2. R2TP (human HSP90, RUVBL1/2, PIH1D and RPAP3) is required for release of SHQ1. The last step in H/ACA snoRNP assembly is the release of NAF1 and binding of GAR1. Processing factors are in shades of green, assembly factors in shades of blue and snoRNP core proteins in shades of orange. To simplify, the crosstalk between transcription, processing and assembly steps is not depicted here.
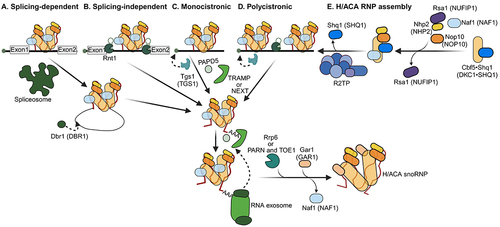
In humans, there are currently 505 snoRNAs validated by sequencing [Citation11,Citation52], while over 2000 have been predicted [Citation53]. About 90% of human snoRNAs are intronic and embedded within introns of either protein-coding genes or long non-coding RNAs (lncRNAs) [Citation52]. Intronic snoRNAs are commonly encoded in the introns of a family of protein-coding genes known as the 5’-terminal oligo-polypyrimidine (5’-TOP) genes, which are associated with ribosome biogenesis and translation [Citation54,Citation55]. 5’-TOP genes were recently shown in a genome-wide analysis to have dual-initiation promoters, providing an alternative mechanism to non-sense-mediated mRNA decay (NMD) for regulation of snoRNA expression relative to the host gene [Citation52,Citation56].
The variation in genomic organization across snoRNAs points to the importance of maturation pathways in the function of snoRNAs. Recent work has described tissue specificity and differential expression of snoRNAs in development and disease [Citation52,Citation57–60]. A frequent observation is the differential expression of snoRNAs compared to their host genes [Citation52,Citation61–63]. Specifically, ~20% of snoRNAs are enriched in brain or reproductive tissues, where the majority are within long non-coding RNA (lncRNA) genes and show a correlation in their abundance with the lncRNA [Citation52]. In contrast, the uniformly expressed snoRNAs are mostly within protein-coding host genes and generally show a lack of correlation or an inverse relationship with their host gene expression levels [Citation52]. Much of the discrepancy in expression of snoRNAs and their host genes can be explained by known mechanisms of RNA regulation [Citation48] including NMD [Citation64], dual initiation promoters [Citation56] and alternative processing of snoRNAs [Citation65]. A recent computational study showed that motif conservation, overall snoRNA stability, formation of terminal stem and the host gene expression serve as main predictors of snoRNA expression [Citation66]. Although it is evident that the genomic organization of a snoRNA dictates its maturation path, it remains unclear to what extent a snoRNA’s pathway to maturity influences its abundance and function.
snoRNA transcription
In most organisms including yeast and human, the majority of snoRNAs are transcribed by RNA Polymerase II. In yeast, only one snoRNA (SNR52) is transcribed by RNA Polymerase III and it is processed similar to snoRNAs transcribed by RNA Pol II [Citation49,Citation67,Citation68]. For a review on aspects of transcription of snoRNAs, see [Citation48]. In brief, transcription of snoRNAs involves the interaction of pre-mature snoRNAs with the transcription machinery. In S. cerevisiae, termination of transcription of short noncoding RNAs, including snoRNAs, is facilitated by the Nrd1-Nab3-Sen1 (NNS) complex and Pcf11, a component of the cleavage and polyadenylation complex [Citation69,Citation70]. The NNS complex is recruited to specific residues on the C-terminal domain of RNA Pol II where it binds the RNA through the RNA binding protein subunits, Nrd1 and Nab3. The helicase Sen1 then interacts with RNA Pol II to terminate transcription of the nascent RNA and the elongation complex dissociates [Citation71–73]. In humans, the NNS is replaced by the Cap-binding complex (CBC) and Arsenic resistance protein 2 (ARS2), which interact to terminate transcription of independently transcribed snoRNAs or those that are capped [Citation74,Citation75]. For intronic human snoRNAs, little is known about their association with transcription machinery [Citation48].
Processing of premature snoRNAs
Much like other cellular RNAs, snoRNAs are transcribed as larger precursors that need to be assembled with proteins and trimmed to form their mature structures. Folding, assembly, modification, and processing of snoRNAs are closely monitored by the action of several proteins, many of which are active members of RNA surveillance pathways [Citation76]. In this section, we will summarize the key aspects of processing of precursor snoRNAs in yeast and humans. A list of factors known to be involved in processing of snoRNAs in human and yeast is summarized in and the maturation steps are depicted in for box C/D snoRNAs and for box H/ACA snoRNAs.
Table 1. Factors involved in processing of snoRNAs in human and yeast.
snoRNA processing in yeast
S. cerevisiae has served as a key model organism for revealing many of the factors and steps involved in snoRNA maturation. In yeast, snoRNA processing is primarily defined by a snoRNAs’ genomic organization [Citation48]. With the exception of U3, snR4, snR13 and snR45, as well as some box H/ACA snoRNAs, the majority of independently transcribed, monocistronic snoRNAs undergo 5’-end processing by the endonuclease Rnt1 at the Rnt1 cleavage tetraloop AGNN motif [Citation93]. This cleavage occurs co-transcriptionally and removes 5’ stem loop extensions from premature snoRNAs [Citation95]. Cleavage by Rnt1 recruits 5’-3’ exonucleases Rat1 and Xrn1 that trim the 5’ end of the premature snoRNA [Citation110]. Box C/D and H/ACA snoRNAs differ in their maturation at the stage of cap modification. Most C/D snoRNAs are transcribed with 5’ stem-loop caps that are removed by Rnt1 cleavage [Citation93,Citation95], while the majority of H/ACA snoRNA caps are converted to trimethylguanosine (TMG) by Tgs1 [Citation87,Citation91–94,Citation111]. Polycistronic snoRNAs follow a similar maturation pathway. In this case, Rnt1 is also required for cleaving between each snoRNA [Citation112]. The maturation of polycistronic snoRNAs is otherwise the same as independently transcribed monocistronic snoRNAs [Citation48,Citation113]. Intronic snoRNAs are either spliced out of their host gene or released through a splicing-independent pathway [Citation88]. Intronic snoRNAs that are spliced from their host gene form intron lariats that are subject to debranching by Dbr1 [Citation89].
The 3’ end processing is similar for all yeast snoRNAs and requires association with the multi-subunit exonuclease RNA exosome complex [Citation114,Citation115]. RNA exosome offers three distinct routes for RNA trimming: Rrp6 trimming at the cap, threading through the exosome for Dis3 trimming and Dis3 direct access without threading [Citation98]. While there is some selectivity of different exosome substrates for a specific path, it appears that snoRNAs can use both channel-threading and direct access to Dis3 for processing, whereas snoRNA degradation mainly occurs via channel-threading [Citation116]. The precise pathways that guide snoRNAs to specific nuclear exosome conformations and exonucleases remain unknown [Citation98]. For 3’ trimming, snoRNAs undergo oligoadenylation to acquire short poly(A) tails to attract exonucleases. Polyadenylation links pre-snoRNA transcription termination to 3’ end processing and degradation [Citation117] and is carried out by the TRAMP complex [Citation118], composed of Mtr4 helicase, Trf4 or Trf5 poly(A) polymerases, and Air1 or Air2 zinc knuckle RNA-binding proteins. Three combinations of these factors have been validated to form complexes in vivo: TRAMP 4–1 (Mtr4, Trf4, Air1), TRAMP 4–2 (Mtr4, Trf4, Air2) and TRAMP 5–1 (Mtr4, Trf5, and Air1) [Citation119]. Of these complexes, TRAMP 4–1 and TRAMP 4–2 participate in snoRNA processing [Citation119]. The RNA exosome removes short poly(A) tails added by TRAMP and the last few nucleotides are removed by the exosome cofactor exonuclease Rrp6, which is in a complex with Rrp47, an exosome binding factor [Citation101,Citation111,Citation114,Citation118,Citation120,Citation121]. Exonucleases are inhibited by the presence of core snoRNP proteins, preventing complete degradation of the snoRNA [Citation29,Citation122,Citation123]. The La-homologous protein (Lhp1) is shown to bind to the poly(U) rich tracts at the 3’ end of U3 snoRNAs after Rnt1 cleavage to provide stabilization during trimming [Citation108].
snoRNA processing in humans
In contrast to what is known for yeast, knowledge of snoRNA processing in humans is limited. Aside from U3, U8, U13 and telomerase snoRNA, in humans, most snoRNAs are processed from the introns of host genes. Independently transcribed human snoRNAs are m7G capped and their 5’-end processing either involves TMG hypermethylation by TGS1 or endonucleolytic cleavage; however, information about 5’- and 3’-end processing of independently transcribed human snoRNAs is very limited [Citation48,Citation82,Citation111].
Human intronic snoRNAs largely mature through splicing of the host transcript, debranching of the intron lariat by DBR1 [Citation124] and subsequent exonucleolytic processing of the debranched snoRNA by XRN2/XRN1 [Citation97,Citation125–127]. An alternative pathway for human snoRNA maturation involves the splicing-independent processing of intronic snoRNAs by endonucleolytic cleavage [Citation50]. An endonuclease, XendoU, responsible for releasing intronic snoRNAs from the host transcript was identified in Xenopus laevis and has a human homolog HPP1 [Citation128]; however, HPP1 has not been shown to cleave human intronic snoRNAs. In humans, the spliceosomal RNA helicase, Aquarius (AQR), which drives the catalytic activation of the spliceosome together with the helicase PRP2 [Citation129], is proposed to serve as a molecular linker coupling pre-mRNA splicing to the biogenesis of C/D snoRNPs and deposition of the exon junction complex [Citation85,Citation86,Citation130]. AQR binds ~ 50 nts upstream of the intron branch site in a position-dependent manner. Its role in splicing is likely to aid in folding the intronic C/D snoRNA for association with core snoRNP proteins.
3’-End snoRNA processing in humans involves the recruitment of RNA exosome by the NEXT (Nuclear EXosome Targeting) complex to the intronic snoRNAs [Citation106]. The NEXT complex is composed of RBM7, ZCCHC8 and MTR4, in which RBM7 brings NEXT to the snoRNA [Citation106]. The poly(A)-polymerase PAPD5, a subunit of the human TRAMP complex, places a short poly(A) tail at the 3’-end of snoRNAs that recruit exonucleases including the PARN and TOE1 deadenylases [Citation105,Citation109,Citation131]. PARN and TOE1 appear to have overlapping roles in removing short poly(A) tails; however, PARN is localized to the nucleolus and hence is involved in 3’-end trimming of snoRNAs, whereas TOE1 is mainly found in Cajal bodies and involved in trimming of scaRNAs [Citation109,Citation132]. Another 3’-5’ exonuclease responsible for trimming snoRNAs from their 3’ ends, Rexo5, was identified in Drosophila melanogaster and has a human homolog REXO5 that may also play a role in 3’ trimming of human snoRNAs [Citation133].
Alternative processing and degradation of snoRNAs
Processing of RNAs provides dynamic flexibility in gene expression and can impact the expression, localization and function of the RNAs. Besides their canonical processing pathways, snoRNAs can undergo processing that leads to the generation of alternative snoRNA forms [Citation134–137]. An important distinction in snoRNA processing is whether a snoRNA is processed, stable and functional or is a degradation product of precursors or perhaps the host gene is defectively spliced. Recent work has shown that splicing defects can impact snoRNA biogenesis and lead to snoRNA degradation by NMD and the generation of hybrid mRNA-snoRNA products [Citation64,Citation138]. However, stable fragments of small RNAs that are differentially processed and derived from snoRNAs [Citation139] with defined molecular functions e.g. in snoRNA regulation [Citation65] or RNA binding protein interaction [Citation140] have also been described. Here, we will briefly review the list of RNA species that are derived from snoRNAs or their precursors including small nucleolar-derived RNAs (sdRNAs), stable lariats bearing a snoRNA (slb-snoRNAs), hybrid mRNA-snoRNAs (hmsnoRNAs), long non-coding RNAs with snoRNA ends (sno-lncRNAs), 5’ snoRNA-capped and polyadenylated lncRNA (SPA-lncRNAs) and snoRNA retaining transcripts (snoRTs).
sdRNAs
Small nucleolar derived RNAs (sdRNAs), also referred to as processed snoRNAs (psnoRNAs) [Citation122] or snoRNA-derived small RNAs (snosRNAs) [Citation141], were identified through analysis of small RNA sequencing [Citation142] and further validated by RNase protection assays [Citation143]. These RNAs generated by poorly understood processing mechanisms share similarities to miRNAs [Citation135]. However, advances in sequencing technologies and bioinformatic analyses have allowed for easier detection and differentiation of sdRNAs from miRNAs [Citation141,Citation144]. sdRNAs can be processed from either box C/D [Citation134,Citation143,Citation145] or box H/ACA snoRNAs [Citation135,Citation146]. The box C/D sdRNAs are generally 21–22 nts in length [Citation145], while H/ACA sdRNAs are around 17–19 nts [Citation135]. Both C/D and H/ACA derived sdRNAs originate from ends of mature snoRNAs [Citation139], with box C/D sdRNAs mostly processed from the 5’ end of snoRNAs and box H/ACA sdRNAs from the 3’ end of host snoRNAs [Citation147]. sdRNAs are found both in the nucleus and the cytoplasm. They are shown to be able to associate with snoRNP core proteins, as well as Argonaute proteins, and have miRNA-like or pi-RNA-like activities [Citation146–151]. In addition, sdRNAs can associate with the ribosome and impact translation in a stress-dependent manner [Citation152]. While some regulatory steps that lead to production of sdRNAs have been uncovered, the factors involved and the relationship between sdRNA expression and host snoRNA expression remain largely unknown [Citation147,Citation148].
Slb-snoRNAs
Unlike sdRNAs that are further processed, slb-snoRNAs are unusually stable intron lariats that evade debranching. A subset of snoRNAs can form slb-snoRNAs, which if exported to the cytoplasm in a specific tissue or species, can prevent further processing of snoRNAs into mature forms [Citation65]. Interestingly, even though the protected slb-snoRNAs can associate with snoRNP proteins including DKC1, the formation of slb-snoRNA prevents the snoRNA’s guide activity [Citation65]. Thus, slb-snoRNAs can play a role in regulating the expression of snoRNAs, thereby changing the pool of available snoRNPs for RNA processing, chemical RNA modification, or other snoRNA-mediated cellular activities.
hmsnoRNAs
Partially processed hybrid mRNA-snoRNA (hmsnoRNA) transcripts are produced when splicing is perturbed through inactivation of splicing factors or mutation of splice site [Citation138]. These hybrid transcripts contain both mRNA and small RNA components of the host genes. Interestingly, despite the predominant nucleolar localization of snoRNAs, the aberrant mRNA-snoRNA hybrids are processed at their 3´ ends by the nuclear exosome but degraded in the cytoplasm by Dcp1/2-mediated decapping and 5’-3’ exonuclease activity of Xrn1 [Citation138]. In the absence of Xrn1, a mutant 5´-3´ exonuclease Rat1 (Xrn2), which is delocalized from the nucleus to the cytoplasm, can target hmsnoRNAs for degradation, further suggesting the cytoplasmic decay of hmsnoRNAs. HmsnoRNAs appear to bind to Nop10 core H/ACA protein and mutated hmsnoRNAs that are unable to assemble into snoRNP are degraded by Rat1 in the nucleus [Citation138]. It remains unclear whether hmsnoRNAs can be generated under physiological conditions where splicing activity is reduced or inhibited, e.g. during stress conditions.
Sno-lncRNAs, SPA-lncRNAs and snoRTs
sno-lncRNAs are hybrid snoRNA and long non-coding RNAs excised from the introns of genes that host multiple snoRNAs [Citation140]. The most abundant sno-lncRNA in human embryonic stem cells is encoded in an imprinted genomic region that is deleted in Prader-Willi Syndrome (PWS) [Citation153–156]. sno-lncRNAs are generated from two snoRNAs with an unspliced sequence bridging them. At least some sno-lncRNAs are shown to bind to the splicing factor FOX2 and change patterns of splicing [Citation156]. A similar alternatively processed class of snoRNAs with a poly(A) tail are 5’ snoRNA-capped and polyadenylated lncRNA (SPA-lncRNAs). The SPA-lncRNAs are much longer than sno-lncRNAs, and unlike the majority of other lncRNAs that are capped by m7G, they have a snoRNP at their 5´ ends [Citation140]. The processing of SPA-lncRNAs is splicing-independent and the snoRNA structure protects their 5’ end from digestion by XRN2, while their 3’ end is processed and polyadenylated [Citation12,Citation140]. Both C/D and H/CA snoRNAs can form SPAs [Citation140]. A SPA-lncRNA derived from NOP56 gene, the cytoplasm-located SPA (cSPA), regulates box C/D snoRNA expression level in cis by controlling the splicing of NOP56 transcript thereby regulating NOP56 cellular levels [Citation12]. A cis regulatory mechanism was also recently observed for select box H/ACAs when DKC1 was knocked down [Citation157]. In this case, H/ACA snoRNA-retaining transcripts (snoRTs) bind DKC1 in the cytoplasm. In contrast to NOP56 which only associates with its host gene transcript, a group of H/ACA snoRNA-retaining transcripts associate with DKC1. NMD appears as a key pathway for processing the snoRNA-retaining transcripts [Citation12,Citation64,Citation157].
snoRNP assembly
snoRNAs are protected from degradation by co-transcriptional association with snoRNP proteins that inhibit the 5’ and 3’ trimming of pre-mature snoRNAs by endo and exonucleases [Citation48]. The conserved box sequence elements within snoRNAs as well as the stem structures are critical for regulating the stability, proper localization and assembly of snoRNAs [Citation111]. Most C/D snoRNAs contain terminal stem structures that bring together their 5’and 3’ ends and appear critical for snoRNP assembly. When a typical terminal stem is missing, other stem structures found within the snoRNA or in the flanking intronic sequences aid in snoRNP assembly [Citation158,Citation159]. Although the mechanisms of assembly of proteins onto snoRNAs are not well defined, it is known that several transiently associating proteins, which are collectively termed assembly factors, are required for the formation of functional snoRNP complexes. These assembly factors appear to be important for localization, stability, processing and function of snoRNPs and their functions extend beyond the assembly steps. While each class of snoRNPs employs a distinct set of assembly factors, biogenesis of both C/D and H/ACA classes shares some common principles and factors that we will discuss first.
Unlike mature snoRNPs, which consist of both proteins and RNA molecules, the assembly of snoRNPs from both C/D and H/ACA classes has been shown to initially involve the formation of protein-only complexes including proteins that are specific to each type of snoRNP [Citation160,Citation161] (). The protein-only complexes have been best characterized in human cell lines but many of the tested interactions appear to be conserved in yeast and play a critical role in the biogenesis of snoRNP. Another shared assembly feature of snoRNP is that, at their core, both H/ACA and C/D snoRNPs bind conserved proteins from the L7Ae family of RNA-binding proteins, namely, SNU13 (yeast Snu13) in C/D snoRNP and NHP2 (yeast Nhp2) in H/ACA complexes. The Nuclear FMRP-Interacting Protein 1 (NUFIP1) and its yeast homolog Rsa1 are shown to be required for assembling both C/D and H/ACA snoRNPs through binding to L7Ae family members to bring them to other core proteins in immature complexes and preventing premature formation of catalytically active snoRNPs [Citation161,Citation162]. In addition, Rsa1 and NUFIP1 are shown to link snoRNPs with Hsp90 chaperone and AAA+ ATPases, Rvb1 and Rvb2 [Citation162]. The HSP90•R2TP protein complex, consisting of the proteins Hsp90, Rvb1 and Rvb2, Pih1 and Tah1 (yeast homologues of human HSP90, RUVBL1, RUVBL2, PIH1D1 and RPAP3, respectively), is a well-defined protein complex responsible for chaperoning the formation of snoRNPs and several other macromolecular protein complexes [Citation163–165]. The role of R2TP in snoRNP assembly was first described in yeast [Citation166] and was comprehensively reviewed elsewhere [Citation111]. The R2TP/PFDL complex, or the particle for arrangement of quaternary structure (PAQosome), forms in mammals through the association of R2TP with a prefoldin-like (PFDL) module [Citation167]. The PAQosome binds to various proteins directly or indirectly and uses specific assembly factors that serve as adaptors to engage in assembly of RNP complexes including both H/ACA and C/D snoRNPs [Citation165,Citation168].
Assembly of box C/D snoRNPs
Box C/D snoRNPs bind to four conserved essential core proteins, NOP56, NOP58, SNU13 and FBL. For the formation of the C/D snoRNPs, SNU13 binds to the snoRNA through interaction with the K-turn motif [Citation169,Citation170] (). In humans, C/D snoRNP assembly is thought to occur in a splicing-dependent manner in coordination with the helicase AQR [Citation85,Citation171]. Some human box C/D snoRNAs can get post-transcriptionally modified, resulting in the formation of N6-methyladenine at a key A•G base pair required for RNA folding and binding of SNU13 protein [Citation172]. Assembly of the box C/D snoRNP complexes can therefore be post-transcriptionally regulated at an early step. The early pre-snoRNP complex of snoRNA and SNU13 binds to NOP58, in the presence of the heterodimer of NUFIP1 and ZNHIT3 [Citation161,Citation173]. Before assembly into pre-snoRNPs, the extended and unfolded regions of NOP58 are stabilized by the HSP90/R2TP complex [Citation174,Citation175]. In human cells, the assembly factor NOPCHAP1 links NOP58 to HSP90/R2TP by making direct interactions with NOP58 and RUVBL1/2 ATPases [Citation176]. An ATP-dependent rearrangement that involves additional assembly factors then leads to association of NOP56 with NOP58 and recruitment of FBL for formation of an active snoRNP complex.
Quantitative proteomic approaches studying box C/D snoRNP assembly in human cells have revealed the formation of an early protein-only complex containing core snoRNP proteins SNU13 and NOP58 and the assembly factors NUFIP1, ZNHIT3, ZNHIT6 and RUVBL1/2, downstream of R2TP [Citation161]. ZNHIT3 (yeast Hit1) is required for maintenance of the steady-state levels of NUFIP1 [Citation173] and is thought to be released upon binding of the protein-only complex to snoRNAs. However, RUVBL1/2, ZNHIT6 and NUFIP1 remain associated with box C/D snoRNAs until the final stages of maturation [Citation161]. Both ZNHIT3 and ZNHIT6 belong to the ZNHIT family of proteins and contain an extended Zn-finger domain, called the Zn-finger HIT domain, which appears to be interchangeable between the two proteins and required for snoRNA stability and their direct binding to RUVBL1/2 [Citation177–180]. A conserved region downstream of the ZNHIT domain in Bcd1 (yeast homologue of human ZNHIT6) was shown to be required for the interaction of the protein with snoRNAs and Snu13 [Citation180]. Furthermore, Bcd1 was shown to interact with Rvb1/2 in an ATP-dependent manner [Citation181], to be recruited co-transcriptionally to the site of snoRNP assembly and to be critical for assembly of Nop58 into pre-snoRNP complexes [Citation182]. While many of the players involved in box C/D snoRNP assembly have been revealed, the mechanisms and regulatory steps that trigger the maturation during box C/D snoRNP assembly remain unknown. The chronology of the steps and their coordination with other cellular processes, e.g. transcription and splicing, also remain poorly characterized.
Assembly of box H/ACA snoRNPs
Box H/ACA snoRNPs consist of a set of four evolutionarily conserved core proteins that bind to a hairpin-hinge-hairpin-tail structured RNA. In contrast to splicing-dependent C/D snoRNP assembly in humans, H/ACA snoRNP assembly is thought to occur in a co-transcriptional and splicing-independent manner [Citation183–185]. The core H/ACA snoRNP proteins associate with snoRNAs co-transcriptionally with the assistance of assembly factors (). Each hairpin binds to a heterotetramer composed of GAR1, NHP2, NOP10 and DKC1, which catalyzes the modification. Structural analyses have revealed that the organization of the two heterotetramers is asymmetric and that they interact with each other through dimerization of DKC1 [Citation39,Citation186]. While archaeal H/ACA snoRNPs can be reconstituted in vitro using transcribed RNA and recombinant proteins [Citation37,Citation187,Citation188], assembly of eukaryotic H/ACA snoRNPs requires essential assembly factors, including SHQ1 and NAF1 [Citation111]. Attesting to their critical roles, depletion of these assembly factors leads to degradation of H/ACA snoRNAs and causes rRNA processing defects [Citation189–191]. In the cytoplasm, SHQ1 binds to the RNA-binding surface of DKC1 before its assembly into snoRNPs, thereby stabilizing the protein and preventing it from binding to RNAs [Citation192]. In the nucleus, SHQ1 is released from H/ACA pre-snoRNP with the help of the R2TP complex [Citation193,Citation194]. R2TP directly interacts with DKC1 and SHQ1 to mediate SHQ1 release from the premature snoRNP [Citation195]. In addition, decreased expression of RUVBL1/2 results in loss of NHP2 and DKC1 [Citation193]. NAF1 recruits NHP2, NOP10 and DKC1 to nascent H/ACA snoRNAs co-transcriptionally and is replaced by GAR1, which joins later in the assembly process [Citation184,Citation191,Citation196,Citation197]. A recent study using quantitative proteomics identified additional proteins important for box H/ACA snoRNP assembly and function [Citation160]. Distinct assembly intermediate complexes were identified that lacked GAR1, and GAR1 was found to interact with protein arginine methyltransferases, PRMT1 and the complex of PRMT5 with its cofactors RIOK1 and MEP50 [Citation160]. A protein-only assembly intermediate containing DKC1, SHQ1, NAF1, NOP10 and NHP2, analogous to the complex of yeast Cbf5, Shq1, Naf1, Nop10 and Nhp2 proteins [Citation198], was also defined in mammalian cells [Citation160].
Other established factors involved in H/ACA snoRNP assembly include NUFIP1, TCAB1 (WDR79) and Nopp140. NUFIP1 interacts with NHP2 and is required for maintaining steady-state levels of box H/ACA snoRNAs, but its precise molecular contribution to the assembly of H/ACA snoRNPs remains unknown [Citation162]. Telomerase Cajal Body Protein 1 (TCAB1), an evolutionarily conserved WD40 protein, and the intrinsically disordered Nucleolar phosphoprotein p140 (Nopp140) are both required for recruitment and retention of H/ACA scaRNAs and telomerase RNAs to CBs [Citation43,Citation44,Citation46,Citation199–202]. The SMN complex also appears to be involved in H/ACA snoRNP assembly through its direct interaction with GAR1 [Citation203,Citation204], association with NUFIP1 [Citation163] and with H/ACA snoRNPs including telomerase [Citation205]. Future experiments are needed to fully characterize the biological significance of all complexes formed during H/ACA snoRNP assembly and to reveal the principles that govern the formation of a catalytically active H/ACA snoRNP complex.
snoRNA associated factors
Besides processing and assembly factors, several other proteins have been identified to affect the function of snoRNP complexes either through interaction with core snoRNP proteins or by binding directly to snoRNAs. We refer to these as snoRNP-associated factors and summarize them here in alphabetical order:
EZH2
The enhancer of zeste homolog 2 (EZH2), the catalytic subunit of Polycomb Repressive Complex 2 (PCR2), is a lysine methyltransferase that directs histone H3 lysine 27 tri-methylation to target promoters for gene silencing [Citation206,Citation207]. Besides its catalytic role, EZH2 binding at promoters can activate or repress gene expression depending on H3K27 modification marks [Citation208]. Dysregulated EZH2 levels or its mutations are common in cancer and predict poor clinical outcomes [Citation209]. Recently, EZH2 was shown to directly interact with FBL and Nop56 and bridge their interaction during box C/D snoRNP biogenesis [Citation210]. Downregulation of EZH2 results in dysregulated rRNA 2’-O-methylation pattern, impacts global translation and causes a significant reduction in IRES-mediated translation initiation [Citation210]. These outcomes are similar to what is observed when 2’-O-methylation is globally perturbed by FBL knockdown [Citation211] or when C/D snoRNP assembly is perturbed [Citation212]. While the connection of EZH2 to other snoRNP assembly steps remains unknown, these findings suggest the EZH2-mediated step of snoRNP biogenesis could be considered as a potential cancer therapeutic target.
FMRP
Fragile X mental retardation protein (FMRP) is an RNA-binding protein that is abundant in the cell, and its loss of expression leads to fragile X syndrome [Citation213]. Human FMRP was shown to interact with select box C/D snoRNAs, but it did not interact with FBL [Citation214]. This suggests that the interaction of FMRP with select snoRNAs is not part of snoRNP complexes. Interestingly, knockout cells with ablated expression of FMRP exhibit an altered 2’-O-methylation pattern at distinct sites in both small and large ribosomal subunits, and the observed 2’-O-methylation changes do not correlate with changes in snoRNA expression levels [Citation214]. Future experiments are required to reveal the significance of FMRP interactions with select snoRNAs and the mechanisms by which FMRP contributes to regulation of rRNA modification. It is possible that FMRP’s interactions with the ribosome [Citation215,Citation216] play a role in the alteration of the rRNA 2’-O-methylation.
FUS
Fused in Sarcoma (FUS) is an RNA/DNA-binding protein present in both the nucleus and cytoplasm with significant roles in binding, splicing and transporting RNAs [Citation217]. It was recently shown that FUS interacts with snoRNAs and that loss of FUS expression can result in increased snoRNA expression and alter both pseudouridylation and 2’-O-methylation levels in rRNA and spliceosomal small nuclear RNAs [Citation218]. Crosslinking immunoprecipitation followed by sequencing (CLIP-seq) and RNA immunoprecipitation (RIP) experiments showed that FUS interacts with snoRNAs at sites that overlap the snoRNAs’ host genes. It remains unclear at what stage in snoRNA processing FUS associates with snoRNAs. However, previous studies suggest that FUS binds introns of genes [Citation219]. Furthermore, knockout experiments in cell lines suggest that FUS binds snoRNAs prior to snoRNP assembly, as there is no change in core protein expression levels upon knockout of FUS [Citation218]. Loss of FUS expression leads to defects in splicing, which may be related to the role FUS plays in modulating spliceosomal RNA modification [Citation219].
Helicases
RNA helicases can modulate the activity of snoRNAs by affecting their binding or release from substrates [Citation220]. The ATP-dependent RNA helicase Prp43 associates with both classes of snoRNAs, and loss of Prp43 expression leads to accumulation of snoRNAs in Prp43 binding regions on pre-ribosomes [Citation221,Citation222]. The RNA helicase Dbp3 is responsible for regulating snoRNA•rRNA dynamics and has also been shown to be necessary for the processing of intron-encoded U18 and U24 snoRNAs [Citation223]. Dysregulation of both Prp43 and Dbp3 helicases can lead to alterations in 2’-O-methylation levels, suggesting that failure to release and recycle snoRNPs disrupts efficient rRNA modification [Citation221,Citation223]. RNA helicases DHX37 (Dhr1 in yeast), Rok1 and Has1 also mediate snoRNP release from the rRNA during ribosome biogenesis. The essential snoRNA U3 is released by DHX37/Dhr1, regulating the formation of the central pseudoknot [Citation224,Citation225]. snR30 is released by Rok1 [Citation226,Citation227]. The U14 snoRNA is released by Has1 [Citation228], and loss of Has1 leads to accumulation of specific snoRNPs [Citation229].
LARP7
La-related protein 7 (LARP7) is an RNA-binding protein, which is present in the nucleus and localizes to the nucleoplasm, nucleoli and Cajal bodies [Citation230,Citation231]. It participates in transcription termination by binding to U-rich regions at the 3’ end of the 7SK RNA [Citation230]. As part of the 7SK snRNP, LARP7 is also an integral protein of the little elongation complex (LEC) and functions in recruiting RNA Pol II to the promoter of independently transcribed snoRNAs and snRNAs [Citation232]. Dysregulation of LARP7 has been reported in several cancers, and loss of LARP7 has been shown to cause Alazami syndrome in a 7SK RNP independent manner, through a reduction in telomerase activity [Citation233]. LARP7 has recently been shown to act as a regulator of box C/D snoRNP function by interacting with FBL [Citation233,Citation234]. Specifically, LARP7 has been shown to interact with U6 snRNA and select box C/D snoRNAs that guide U6 modifications [Citation230,Citation234] to facilitate the loading of U6 snRNA onto box C/D snoRNPs for 2’-O-methylation.
NMI
N-myc interactor (NMI) is a protein that interacts with N-MYC, C-MYC, STATs as well as transcription factors containing the Zip, HLH or HLH-Zip motifs [Citation235]. It has been implicated in the regulation of endothelial-to-mesenchymal transition (EMT), a common phenomenon in tumour initiation [Citation236]. In hypoxic environments, such as those found in tumours, loss of NMI is associated with an increase in EMT in invasive breast cancer [Citation237,Citation238]. NMI was shown to bind both box C/D snoRNAs and FBL in breast cancer cells through immunoprecipitation, and it was suggested that this interaction contributes to the altered 2’-O-methylation status of rRNA and increased IRES-mediated translation in breast cancer cells cultured under hypoxic conditions [Citation239]. The molecular mechanism by which NMI interacts with box C/D snoRNAs and FBL to regulate snoRNP function and translation remains unclear.
NOP2/NSUN1
Studies in the model organism budding yeast have shown that Nop2 is an essential ribosome biogenesis factor required for chemical modification of large ribosomal subunit RNA [Citation240]. Nop2 is a 5-methylcytosine (m5C) methyltransferase from the NSUN protein family, which has seven members in humans. Overexpression of human Nop2, NSUN1, is observed in a wide range of human cancers and linked to poor clinical outcomes [Citation241]. A recent study in human cells found that NSUN1 binds snoRNP assembly factors RUVBL1 and NUFIP1, as well as snoRNP core proteins NOP56 and FBL, and contributes to stable box C/D snoRNP assembly [Citation242]. Interestingly, both wildtype and catalytically inactive NSUN1 interact with mature snoRNAs, including U8 and U3 to facilitate their assembly into RNP complexes and the methyltransferase activity of NSUN1 appears dispensable for its role in regulation of snoRNP complex stability [Citation242]. These findings suggest a role for NOP2/NSUN1 in early steps of snoRNP assembly.
NPM1
Nucleophosmin1 (NPM1) is a nucleolar RNA binding protein that plays key roles in several cellular processes including ribosome biogenesis, DNA repair and regulation of cell growth and proliferation [Citation243]. Mutations in the NPM1 gene have been linked to various types of cancers, including acute myeloid leukaemia [Citation244,Citation245]. In AML, a mutation in the NPM1 gene leads to the production of an abnormal form of the NPM1 protein that is found in the cytoplasm of cells, instead of its normal location in the nucleus. This abnormal protein is thought to contribute to the development of cancer by promoting uncontrolled cell growth and inhibiting normal cell death processes [Citation246,Citation247]. High-throughput sequencing of RNA isolated by crosslinking immunoprecipitation (HiTS-CLIP) analyses revealed that snoRNAs are abundant binding partners of NPM1 [Citation248]. Further, IP experiments showed that NPM1 directly binds FBL independent of RNA [Citation248]. An NPM1-/- MEF model showed that the loss of NPM1 expression led to site-specific decreases in 28S rRNA 2’-O-methylation status, while the snoRNA abundance was unchanged [Citation248]. Thus, NPM1 appears to play a role in regulating snoRNA-guided modifications through interaction with FBL.
PARP1
Poly-ADP-ribosyltransferase (PARP1) is an enzyme that catalyses the transfer of Poly-ADP-ribose onto proteins and has also been shown to bind RNAs. PARP1 is predominantly localized to the nucleus with roles in DNA repair [Citation249,Citation250]. Its dysregulation is associated with several cancers, which has led to its popularity as a cancer drug target [Citation251,Citation252]. PARP1 has been implicated in ribosome biogenesis through the identification of interactions of the PARP1 DNA-binding domain with both box C/D and box H/ACA snoRNAs by RNA immunoprecipitation coupled with deep sequencing (RIP-seq) in the MCF7 breast cancer cell line [Citation253]. These interactions were shown to catalyse PARP-1 activity. PARP-1 targets were identified by mass spectrometry and identified DEAD-box RNA helicase DDX21. It was shown that activation of PARP-1 was snoRNA dependent and resulted in the PARylation of DDX21. This was snoRNA-specific and did not appear to impact rRNA modification pattern, rather it upregulated rDNA transcription.
TDP43
Trans-activating response region DNA-binding protein 43 (TDP43) predominantly localizes to the nucleus and binds to DNA and RNA [Citation254]. TDP43 specifically binds to UG-rich regions in RNA [Citation255]. Its predominant role is in the regulation of splicing, and its dysregulation is associated with amyotrophic lateral sclerosis (ALS), which results in mislocalization of TDP43 to the cytoplasm [Citation256,Citation257]. TDP43 can bind directly to UG-rich motifs of some box C/D scaRNAs (2, 7, 9 and 28), and siRNA-mediated knockdown of TDP43 was shown to reduce 2’-O-methylation of U1 and U2 snoRNAs, the targets of scaRNA7 and scaRNA9, respectively [Citation255]. Reduction in TDP43 expression also led to the mislocalization of scaRNAs [Citation255].
TFIP11
TFIP11 is a splicing factor present in the nucleolus and Cajal Bodies. Yeast TFIP11 (Ntr1) is a cofactor of spliceosomal helicase Prp41 (human DHX15) [Citation258–261]. It was recently shown that TFIP11 regulates human U6 2’-O-methylation through transient interaction with snoRNPs independent of DHX15 [Citation262]. Knockdown of TFIP11 leads to decreased SNORD association with FBL and decreased 2’-O-methylation of U6 snRNA; however, TFIP11 has not been shown to directly bind core snoRNP proteins [Citation262]. This suggests a role for TFIP11 as a regulatory factor for specific snoRNPs similar to LARP7 and NPM1.
SMN
The Survival of Motor Neuron (SMN) complex is a protein complex composed of the SMN protein and six Gemins (Gemins 2–7) [Citation203,Citation263] required for the assembly of snRNPs for pre-mRNA splicing [Citation163] and involved in multiple other cellular RNA pathways [Citation264]. SMN protein deficiency causes spinal muscular atrophy (SMA), a genetic disorder that results in progressive muscle weakness and atrophy due to the degeneration of motor neurons in the spinal cord [Citation265,Citation266]. The SMN complex interacts with snoRNP core proteins GAR1 and FBL and is functionally linked to NUFIP1 [Citation203,Citation267]. Furthermore, loss of SMN expression leads to mislocalization of snoRNAs to nuclear gems and Cajal bodies [Citation203]. The precise contribution of SMN to snoRNP assembly remains unknown.
The identification of new factors regulating snoRNP maturation and function points to the incomplete picture of snoRNP-mediated regulation of gene expression. A role is emerging for snoRNP-associated factors as another level of control in gene expression regulation, but the mechanisms by which these factors function are not fully understood yet. Future work is likely to elucidate a myriad of factors involved in regulation of snoRNP function by impacting their transcription, processing, localization, assembly and recycling.
snoRNA maturation defects in disease
snoRNAs play a prominent role in ribosome biogenesis and RNA modification, and thus, defects in their maturation have a variety of consequences for the cell, many of which likely stemming from deregulated translation. Defects in snoRNA maturation can occur through changes in their sequence, transcription, processing or assembly and impact the steady-state level of snoRNAs as well as the function of mature snoRNP complexes. Given the significant role snoRNAs play in gene expression regulation, a snoRNA’s inability to accurately fulfil its function can lead to a wide array of human diseases ranging from cancer to neurodevelopmental disorders. Here, we group diseases linked to snoRNAs into two major classes: those affecting the steady-state levels of snoRNAs for which there are no data on changes in snoRNA-guided modifications and those that affect both snoRNA levels and RNA modifications.
Changes in snoRNA levels in disease
Mutations or deletions of snoRNA genes have been linked to human disease. Two large clusters of orphan box C/D snoRNA repeats are specifically expressed in the brain, including SNORD115 and SNORD116 [Citation59]. These clusters of snoRNAs undergo imprinting such that only the paternal copies are expressed (for a recent review, see [Citation155]). Deficiency resulting from a microdeletion in the SNORD116 cluster is thought to be the cause of PWS [Citation153–155]. Although sites of modification have been computationally predicted for SNORD116, no sites of modification have been validated, rather SNORD116 has been shown to function in neuron-specific regulation of mRNA expression and splicing [Citation14,Citation156,Citation268]. Another snoRNA-associated genetic neurological disorder, leukoencephalopathy with calcifications and cysts (LCC), also known as Labrune syndrome, is caused by bi-allelic mutations in the gene SNORD118, which encodes the vertebrate-specific box C/D U8 snoRNA that is required for processing of 5.8S and 28S rRNAs [Citation269].
Mutations of genes encoding snoRNP core or assembly factors have also been linked to human disease. PEHO syndrome, a rare genetic disorder characterized by progressive encephalopathy, hypotonia, optic atrophy and other neurological symptoms, is linked to mutations in the ZNHIT3 gene that encodes an evolutionarily conserved C/D snoRNP assembly factor [Citation161,Citation173]. Studies of PEHO-syndrome-associated ZNHIT3 mutations in yeast revealed that they lead to loss of protein function, resulting in reduced box C/D snoRNA levels and changes in rRNA modification pattern [Citation270]. Inherited mutations in components or assembly factors of the telomerase complex can lead to premature ageing, progressive bone marrow failure and cancer predisposition [Citation271]. Mutations in the NAF1 gene, which encodes a critical H/ACA snoRNP assembly factor, have been linked to a rare disease called Hoyeraal-Hreidarsson syndrome (HHS), a severe variant of dyskeratosis congenita and Coats plus syndrome, a rare genetic disorder characterized by abnormal blood vessel growth, retinal telangiectasia and brain abnormalities. NHP2 mutations have also been linked to dyskeratosis congenita HHS and pulmonary fibrosis [Citation272]. Similarly, mutations in SHQ1 cause early-onset dystonia, developmental delay, neurodegeneration and other neurological defects [Citation273–275]. Loss of SHQ1 protein in fibroblasts from affected individuals causes significant reduction in H/ACA snoRNAs, defects in rRNA processing and ribosome biogenesis [Citation273].
Mutations in snoRNAs and changes in their steady-state levels have also been implicated in neurodegenerative disease and cancer development and progression. For recent reviews on snoRNA dysregulation in neurodegenerative disease and cancer, see references [Citation58,Citation276,Citation277]. In the context of cancer, snoRNAs can function both as tumour suppressors or enhancers [Citation58] and are therefore considered as potential targets for developing cancer therapeutics [Citation278]. Tumour protein 53 (P53) can directly regulate snoRNP by controlling the transcription of genes involved in snoRNPs assembly or function including NOLC1, FBL and DKC1 [Citation279–281]. Because snoRNAs are highly abundant and form stable structures, which are not easily degraded in body fluids, they are also considered for their potential as biomarkers for early detection of neurodegenerative diseases and cancer.
Changes in RNA modification patterns in disease
Advances in methods to quantify snoRNA-guided RNA modifications [Citation282–288] have revealed that RNA modification patterns can be different between healthy and diseased cells in a wide range of human diseases. Whether these changes cause the disease phenotypes, contribute to symptoms or are benign consequences of other major molecular defects remains largely unknown. Changes in levels of snoRNAs [Citation57], snoRNP core proteins [Citation279,Citation289] or snoRNA-associated factors [Citation234,Citation248] can all result in alteration of snoRNA-mediated RNA modifications. In the case of ribosomal RNAs, rRNA modification changes are shown to impact the capacity of global protein synthesis, the fidelity of translation and the preference of ribosomes for specific transcripts, thereby causing or contributing to human disease [Citation15,Citation211,Citation212,Citation270,Citation290,Citation291]. In the context of the spliceosome, snoRNA-guided RNA modifications can affect the recognition and pairing of splice sites and impact the efficiency of splicing, thereby contributing critically to splicing of pre-mRNAs [Citation13,Citation292].
Deregulated expression of the box C/D methyltransferase FBL has been linked to breast tumorigenesis, with p53 repressing FBL expression and regulating rRNA methylation [Citation279]. Analyses of primary breast tumour samples have revealed rRNA 2´-O-methylation changes between cancer subtypes and tumour grades [Citation293]. Aside from cancer, changes in modification patterns have also been shown in developmental diseases. In Alazami syndrome, changes in spliceosomal RNA 2’-O-methylation are a result of the loss of LARP7 expression (see LARP7) [Citation233,Citation294]. X-linked dyskeratosis congenita (DC), which can occur through mutations in core snoRNP protein DKC1, can affect box H/ACA snoRNPs and results in changes in rRNA pseudouridylation [Citation289,Citation295]. Autosomally transmitted DC has been associated with mutations in other box H/ACA core proteins [Citation296,Citation297], as well as changes in rRNA 2’-O-methylation due to the loss of snoRNA-associated factor NPM1 [Citation248,Citation298] (see NPM1). Mutation in DKC1 or NOP10 affecting the interaction of the two proteins was also shown to cause distinct phenotypes of DC and result in reduced rRNA pseudouridylation in patients with nephrotic syndrome with cataracts, hearing impairment and enterocolitis [Citation299]. For reviews on changes in snoRNA-guided modification status in cancer and developmental disease, see [Citation58,Citation151,Citation300–302].
In summary, defects in snoRNA maturation can manifest in a wide range of human diseases and may inform the disease diagnosis. The precise molecular pathology of snoRNA-associated diseases is largely unknown, leaving the key question of how snoRNA changes mechanistically contribute to disease unanswered. Understanding snoRNA maturation and regulatory events can provide mechanistic inroads for therapeutic interventions.
Concluding remarks
snoRNAs are a diverse family of abundant non-coding RNAs that play critical roles in gene expression regulation. Due to their medium to small size and structure, quantification and sequencing of human snoRNAs have been challenging and their characterization has lagged behind other non-coding RNAs [Citation52]. However, growing evidence has established the functional diversity of snoRNAs in a wide array of critical cellular processes ranging from rRNA processing and chemical modification to splicing, mRNA stability, cholesterol trafficking and metabolic and oxidative stress regulation [Citation17]. Not surprisingly, dysregulation of snoRNA maturation or function can result in major cellular defects and contribute to or cause a wide range of human diseases, including cancer and neurodegenerative disorders [Citation155,Citation277,Citation300,Citation303,Citation304]. Understanding snoRNA maturation is therefore critical not only to uncovering mechanisms of gene expression regulation, RNA processing pathways and advancing non-coding RNA biology, but also to identifying potential diagnostic and therapeutic targets.
To become functional, snoRNAs undergo an intricate series of processing steps, the precise pathway of which largely depends on their genomic localization and function. Much like other cellular RNAs, snoRNAs are initially transcribed as longer precursors with flanking 5’ and 3’ regions that need to be trimmed. These precursors are processed by the coordinated action of several proteins and ribonucleases to generate mature snoRNAs. The maturation steps involve trimming, modification and assembly with proteins, many of which are merely regulating the process transiently and are absent from mature snoRNP complexes. The dynamic protein–protein and RNA–protein assembly/disassembly steps constitute a key aspect of the maturation of snoRNAs that control both the snoRNA processing and its binding with core proteins to form functional complexes. However, the precise mode of action of most snoRNP assembly factors and their relation to processing steps remain unknown to date. It is also largely unclear how the maturation of snoRNAs is coordinated with other cellular processes, such as transcription, ribosome biogenesis and splicing. Studies of snoRNA maturation are, therefore, complicated because they involve untangling multiple simultaneous processing and assembly events, many of which are transient and likely coordinated with other activities. Developing experimental approaches to capture the transient intermediates in this process and combining those with structural biology is required for a better understanding of these events at a molecular level.
Emerging data indicate a tissue-specific expression pattern for many of the snoRNAs in brain and reproductive tissues. In addition, new forms of snoRNAs are being discovered in the form of snoRNA fragments, snoRNA-linked larger RNAs or snoRNA-retained lariats or transcripts. While the canonical snoRNA maturation pathways are established, how these newly emerging forms of snoRNAs are produced remains largely unknown. There are likely additional processing pathways and factors involved in the maturation of snoRNAs, which may be snoRNA, tissue, developmental stage, or even disease-state specific. Future research will likely focus on identifying these novel pathways and factors and understanding how they contribute to snoRNA maturation. In addition, snoRNAs and their core proteins are reported to be associated with several other factors that are not assembly or processing factors but appear to affect the steady-state levels of snoRNAs or the enzymatic activity of snoRNP complexes. The identified factors appear to regulate snoRNA levels by directly interacting with the snoRNAs or impact the function of the mature snoRNP complexes by interacting with the core components especially FBL. The specific mechanisms that regulate these binding events have yet to be fully understood. It is also unclear how these interaction events are regulated and how the association of snoRNPs with different protein partners affects their canonical and non-canonical cellular functions.
Research on snoRNAs and their associated factors and regulators is a growing field of study, and it is likely to continue to expand with advances in high-throughput sequencing technologies for studying small structured RNAs, quantitative mapping of RNA modifications, and RNA–RNA interactions, as well as the development of new tools and approaches for studying dynamically regulated molecular pathways. While many snoRNAs have been identified, the characterization of most in higher eukaryotes and their precise contribution to gene expression regulation remains unknown. The development of new bioinformatics approaches and sequencing technologies will likely facilitate the identification and characterization of novel snoRNAs in different tissues and developmental stages. Such studies will be essential for addressing the question of how deregulated snoRNAs contribute to human diseases with tissue-specific pathological defects. They will also pave the way for understanding the mechanisms underlying these pathological defects and identifying potential therapeutic targets.
In summary, studying snoRNA maturation and function remains a highly active area of research that is important for understanding gene expression regulation, uncovering novel RNAs and regulatory RNA processing mechanisms, identifying potential therapeutic targets and advancing our understanding of non-coding RNA biology. Future experimental and computational studies are needed to elucidate the molecular mechanisms of snoRNA maturation and processing to other derivatives, investigate the contribution of snoRNAs and their associated factors to human disease and bring a mechanistic picture to the snoRNA-mediated regulatory events that affect gene expression regulation.
Data sharing plan
Data sharing is not applicable to this article as no new data were created or analysed in this study.
Acknowledgments
We thank Drs. Sohail Khoshnevis and Daniel Reines, as well as members of the Ghalei laboratory for constructive discussions and feedback on the manuscript. The figures were created with Biorender.com.
Disclosure statement
No potential conflict of interest was reported by the author(s).
Additional information
Funding
References
- Kiss T. Small nucleolar RNAs. Cell. 2002;109(2):145–148. doi: 10.1016/s0092-8674(02)00718-3
- Darzacq X, Jády BE, Verheggen C, et al. Cajal body-specific small nuclear RNAs: a novel class of 2’-O-methylation and pseudouridylation guide RNAs. EMBO J. 2002;21(11):2746–2756. PubMed PMID: 12032087; PubMed Central IDPMC126017. doi: 10.1093/emboj/21.11.2746
- Marnef A, Richard P, Pinzón N, et al. Targeting vertebrate intron-encoded box C/D 2’-O-methylation guide RNAs into the Cajal body. Nucleic Acids Res. 2014;42(10):6616–6629. Epub 20140420 PubMed PMID: 24753405; PubMed Central IDPMC4041459 doi: 10.1093/nar/gku287
- Meier UT. RNA modification in Cajal bodies. RNA Biol. 2017;14(6):693–700. Epub 20161024 PubMed PMID: 27775477; PubMed Central ID PMC5519239. doi: 10.1080/15476286.2016.1249091.
- Deryusheva S, Gall JG. scaRnas and snoRNAs: are they limited to specific classes of substrate RNAs? RNA. 2019;25(1):17–22. Epub 20181009. PubMed PMID: 30301832; PubMed Central IDPMC6298559. doi: 10.1261/rna.068593.118.
- Kiss T. NEW EMBO MEMBER’S REVIEW: small nucleolar RNA-guided post-transcriptional modification of cellular RNAs. EMBO J. 2001;20(14):3617–3622. PubMed PMID: 11447102; PubMed Central ID: PMC125535. doi: 10.1093/emboj/20.14.3617.
- Enright CA, Maxwell ES, Eliceiri GL, et al. 5‘ETS rRNA processing facilitated by four small RNAs: U14, E3, U17, and U3. RNA. 1996;2(11):1094–1099. PubMed PMID: 8903340; PubMed Central ID PMC1369439
- Sharma K, Tollervey D. Base pairing between U3 small nucleolar RNA and the 5’ end of 18S rRNA is required for pre-rRNA processing. Mol Cell Biol. 1999;19(9):6012–6019. PubMed PMID: 10454548; PubMed Central IDPMC84488 doi: 10.1128/mcb.19.9.6012
- Vitali P, Kiss T. Cooperative 2’-O-methylation of the wobble cytidine of human elongator tRNA(Met)(CAT) by a nucleolar and a Cajal body-specific box C/D RNP. Genes Dev. 2019;33(13–14):741–746. Epub 20190606 PubMed PMID: 31171702; PubMed Central IDPMC6601510 doi: 10.1101/gad.326363.119
- Kornprobst M, Turk M, Kellner N, et al. Architecture of the 90S pre-ribosome: a structural view on the birth of the eukaryotic ribosome. Cell. 2016;166(2):380–393. PubMed PMID: 27419870. doi: 10.1016/j.cell.2016.06.014
- Bergeron D, Faucher-Giguère L, Emmerichs AK, et al. Intronic small nucleolar RNAs regulate host gene splicing through base pairing with their adjacent intronic sequences. Genome Biol. 2023;24(1):160. Epub 20230706 PubMed PMID: 37415181 PubMed Central ID: PMC10324135. doi: 10.1186/s13059-023-03002-y
- Lykke-Andersen S, Ardal BK, Hollensen AK, et al. Box C/D snoRNP autoregulation by a cis-ating snoRNA in the NOP56 pre-mRNA. Molecular Cell. 2018;72(1):99–111.e5. doi: 10.1016/j.molcel.2018.08.017
- Bohnsack MT, Sloan KE. Modifications in small nuclear RNAs and their roles in spliceosome assembly and function. Biol Chem. 2018;399(11):1265–1276. Epub 2018/06/17 PubMed PMID: 29908124. doi: 10.1515/hsz-2018-0205.
- Bergeron D, Paraqindes H, Fafard-Couture É, et al. snoDB 2.0: an enhanced interactive database, specializing in human snoRNAs. Nucleic Acids Res. 2023;51(D1):D291–d6. PubMed PMID: 36165892; PubMed Central IDPMC9825428. doi: 10.1093/nar/gkac835
- Sloan KE, Warda AS, Sharma S, et al. Tuning the ribosome: the influence of rRNA modification on eukaryotic ribosome biogenesis and function. RNA Biol. 2017;14(9):1138–1152. Epub 20161202 PubMed PMID: 27911188; PubMed Central IDPMC5699541. doi: 10.1080/15476286.2016.1259781
- Omer AD, Lowe TM, Russell AG, et al. Homologs of small nucleolar RNAs in archaea. Science. 2000;288(5465):517–522. PubMed PMID: 10775111. doi: 10.1126/science.288.5465.517
- Bratkovic T, Bozic J, Rogelj B. Functional diversity of small nucleolar RNAs. Nucleic Acids Res. 2020;48(4):1627–1651. Epub 2019/12/13 PubMed PMID: 31828325; PubMed Central IDPMC7038934. doi: 10.1093/nar/gkz1140
- Watkins NJ, Bohnsack MT. The box C/D and H/ACA snoRnps: key players in the modification, processing and the dynamic folding of ribosomal RNA. Wiley Interdiscip Rev RNA. 2012;3(3):397–414. Epub 20111107 PubMed PMID: 22065625 doi: 10.1002/wrna.117
- Elliott BA, Ho HT, Ranganathan SV, et al. Modification of messenger RNA by 2’-O-methylation regulates gene expression in vivo. Nat Commun. 2019;10(1):3401. Epub 20190730 PubMed PMID: 31363086; PubMed Central IDPMC6667457. doi: 10.1038/s41467-019-11375-7
- Lu B, Chen X, Liu X, et al. C/D box small nucleolar RNA SNORD104 promotes endometrial cancer by regulating the 2’-O-methylation of PARP1. J Transl Med. 2022;20(1):618. Epub 20221224 PubMed PMID: 36566215; PubMed Central IDPMC9790134. doi: 10.1186/s12967-022-03802-z
- Bao HJ, Chen X, Liu X, et al. Box C/D snoRNA SNORD89 influences the occurrence and development of endometrial cancer through 2’-O-methylation modification of Bim. Cell Death Discov. 2022;8(1):309. Epub 20220705 PubMed PMID: 35790714; PubMed Central IDPMC9256700. doi: 10.1038/s41420-022-01102-5
- Sharma S, Yang J, van Nues R, et al. Specialized box C/D snoRNP act as antisense guides to target RNA base acetylation. PLoS Genet. 2017;13(5):e1006804. Epub 20170524 PubMed PMID: 28542199; PubMed Central IDPMC5464676 doi: 10.1371/journal.pgen.1006804
- Tollervey D, Kiss T. Function and synthesis of small nucleolar RNAs. Curr Opin Cell Biol. 1997;9(3):337–342. PubMed PMID: 9159079. doi: 10.1016/s0955-0674(97)80005-1.
- Kos M, Tollervey D. Yeast pre-rRNA processing and modification occur cotranscriptionally. Molecular Cell. 2010;37(6):809–820. Epub 2010/03/30 PubMed PMID: 20347423; PubMed Central ID PMC2860240. doi: 10.1016/j.molcel.2010.02.024.
- Yu G, Zhao Y, Li H. The multistructural forms of box C/D ribonucleoprotein particles. RNA. 2018;24(12):1625–1633. Epub 20180925 PubMed PMID: 30254138; PubMed Central ID PMC6239191. doi: 10.1261/rna.068312.118.
- Jansen RP, Hurt EC, Kern H, et al. Evolutionary conservation of the human nucleolar protein fibrillarin and its functional expression in yeast. J Cell Bio. 1991;113(4):715–729. PubMed PMID: 2026646; PubMed Central IDPMC2288999. doi: 10.1083/jcb.113.4.715
- Ito S, Horikawa S, Suzuki T, et al. Human NAT10 is an ATP-dependent RNA acetyltransferase responsible for N4-acetylcytidine formation in 18 S ribosomal RNA (rRNA). J Biol Chem. 2014;289(52):35724–35730. doi: 10.1074/jbc.c114.602698
- Ito S, Akamatsu Y, Noma A, et al. A single acetylation of 18 S rRNA is essential for biogenesis of the small ribosomal subunit in Saccharomyces cerevisiae. J Biol Chem. 2014;289(38):26201–26212. doi: 10.1074/jbc.m114.593996
- Ganot P, Caizergues-Ferrer M, Kiss T. The family of box ACA small nucleolar RNAs is defined by an evolutionarily conserved secondary structure and ubiquitous sequence elements essential for RNA accumulation. Genes Dev. 1997;11(7):941–956. PubMed PMID: 9106664. doi: 10.1101/gad.11.7.941
- Balakin AG, Smith L, Fournier MJ. The RNA world of the nucleolus: two major families of small RNAs defined by different box elements with related functions. Cell. 1996;86(5):823–834. PubMed PMID: 8797828 doi: 10.1016/s0092-8674(00)80156-7
- Bousquet-Antonelli C, Henry Y, G’Elugne JP, et al. A small nucleolar RNP protein is required for pseudouridylation of eukaryotic ribosomal RNAs. EMBO J. 1997;16(15):4770–4776. PubMed PMID: 9303321; PubMed Central IDPMC1170103. doi: 10.1093/emboj/16.15.4770
- Czekay DP, Kothe U. H/ACA small ribonucleoproteins: structural and functional comparison between archaea and eukaryotes. Front Microbiol. 2021;12:654370. Epub 20210311 PubMed PMID: 33776984; PubMed Central ID PMC7991803. doi: 10.3389/fmicb.2021.654370
- Meier UT. How a single protein complex accommodates many different H/ACA RNAs. Trends Biochem Sci. 2006;31(6):311–315. Epub 20060502 PubMed PMID: 16647858; PubMed Central IDPMC4314714. doi: 10.1016/j.tibs.2006.04.002
- Caton EA, Kelly EK, Kamalampeta R, et al. Efficient RNA pseudouridylation by eukaryotic H/ACA ribonucleoproteins requires high affinity binding and correct positioning of guide RNA. Nucleic Acids Res. 2018;46(2):905–916. PubMed PMID: 29177505; PubMed Central ID PMC5778458. doi: 10.1093/nar/gkx1167.
- Liang B, Zhou J, Kahen E, et al. Structure of a functional ribonucleoprotein pseudouridine synthase bound to a substrate RNA. Nat Struct Mol Biol. 2009;16(7):740–746. Epub 20090528 PubMed PMID: 19478803; PubMed Central ID PMC5706466. doi: 10.1038/nsmb.1624.
- Yang X, Duan J, Li S, et al. Kinetic and thermodynamic characterization of the reaction pathway of box H/ACA RNA-guided pseudouridine formation. Nucleic Acids Res. 2012;40(21):10925–10936. Epub 20120924 PubMed PMID: 23012266; PubMed Central ID PMC3510513. doi: 10.1093/nar/gks882.
- Li S, Duan J, Li D, et al. Reconstitution and structural analysis of the yeast box H/ACA RNA-guided pseudouridine synthase. Genes Dev. 2011;25(22):2409–2421. PubMed PMID: 22085967; PubMed Central IDPMC3222906. doi: 10.1101/gad.175299.111
- Duan J, Li L, Lu J, et al. Structural mechanism of substrate RNA recruitment in H/ACA RNA-guided pseudouridine synthase. Molecular Cell. 2009;34(4):427–439. PubMed PMID: 19481523. doi: 10.1016/j.molcel.2009.05.005
- Nguyen THD, Tam J, Wu RA, et al. Cryo-EM structure of substrate-bound human telomerase holoenzyme. Nature. 2018;557(7704):190–195. Epub 20180425 PubMed PMID: 29695869; PubMed Central ID PMC6223129. doi: 10.1038/s41586-018-0062-x.
- Wang C, Meier UT. Architecture and assembly of mammalian H/ACA small nucleolar and telomerase ribonucleoproteins. EMBO J. 2004;23(8):1857–1867. doi: 10.1038/sj.emboj.7600181
- Ghanim GE, Fountain AJ, Van Roon A-M, et al. Structure of human telomerase holoenzyme with bound telomeric DNA. Nature. 2021;593(7859):449–453. doi: 10.1038/s41586-021-03415-4
- Jady BE. A small nucleolar guide RNA functions both in 2’-O-ribose methylation and pseudouridylation of the U5 spliceosomal RNA. EMBO J. 2001;20(3):541–551. doi: 10.1093/emboj/20.3.541
- Richard P, Darzacq X, Bertrand E, et al. A common sequence motif determines the Cajal body-specific localization of box H/ACA scaRnas. EMBO J. 2003;22(16):4283–4293. PubMed PMID: 12912925; PubMed Central IDPMC175784. doi: 10.1093/emboj/cdg394
- Venteicher AS, Abreu EB, Meng Z, et al. A human telomerase holoenzyme protein required for Cajal body localization and telomere synthesis. Science. 2009;323(5914):644–648. PubMed PMID: 19179534; PubMed Central ID PMC2728071. doi: 10.1126/science.1165357.
- Jády BE, Ketele A, Kiss T. Human intron-encoded Alu RNAs are processed and packaged into Wdr79-associated nucleoplasmic box H/ACA RNPs. Genes Dev. 2012;26(17):1897–1910. doi: 10.1101/gad.197467.112
- Tycowski KT, Shu M-D, Kukoyi A, et al. A conserved WD40 protein binds the Cajal body localization signal of scaRNP particles. Molecular Cell. 2009;34(1):47–57. doi: 10.1016/j.molcel.2009.02.020
- Richard P. A common sequence motif determines the Cajal body-specific localization of box H/ACA scaRnas. EMBO J. 2003;22(16):4283–4293. doi: 10.1093/emboj/cdg394
- Kufel J, Grzechnik P. Small nucleolar RNAs tell a different tale. Trends Genet. 2019;35(2):104–117. doi: 10.1016/j.tig.2018.11.005
- Dieci G, Preti M, Montanini B. Eukaryotic snoRNAs: a paradigm for gene expression flexibility. Genomics. 2009;94(2):83–88. Epub 2009/05/19 PubMed PMID: 19446021. doi: 10.1016/j.ygeno.2009.05.002
- Hirose T, Shu M-D, Steitz JA. Splicing-dependent and -independent modes of assembly for intron-encoded box C/D snoRnps in mammalian cells. Molecular Cell. 2003;12(1):113–123. doi: 10.1016/s1097-2765(03)00267-3
- Piekna-Przybylska D, Decatur WA, Fournier MJ. New bioinformatic tools for analysis of nucleotide modifications in eukaryotic rRNA. RNA. 2007;13(3):305–312. Epub 20070205 PubMed PMID: 17283215; PubMed Central ID PMC1800513. doi: 10.1261/rna.373107.
- Fafard-Couture E, Bergeron D, Couture S, et al. Annotation of snoRNA abundance across human tissues reveals complex snoRNA-host gene relationships. Genome Biol. 2021;22(1):172. Epub 20210604 PubMed PMID: 34088344; PubMed Central IDPMC8176728. doi: 10.1186/s13059-021-02391-2
- Bouchard-Bourelle P, Desjardins-Henri C, Mathurin-St-Pierre D, et al. snoDB: an interactive database of human snoRNA sequences, abundance and interactions. Nucleic Acids Res. 2020;48(D1):D220–D5. Epub 2019/10/11 PubMed PMID: 31598696; PubMed Central ID: PMC6943035. doi: 10.1093/nar/gkz884
- Maxwell ES, Fournier MJ. The small nucleolar RNAs. Annu Rev Biochem. 1995;64:897–934. PubMed PMID: 7574504. doi: 10.1146/annurev.bi.64.070195.004341
- Gentilella A, Morón-Duran FD, Fuentes P, et al. Autogenous control of 5´TOP mRNA stability by 40S ribosomes. Molecular Cell. 2017;67(1):55–70.e4. doi: 10.1016/j.molcel.2017.06.005
- Nepal C, Hadzhiev Y, Balwierz P, et al. Dual-initiation promoters with intertwined canonical and TCT/TOP transcription start sites diversify transcript processing. Nat Commun. 2020;11(1). doi: 10.1038/s41467-019-13687-0
- Faucher-Giguere L, Roy A, Deschamps-Francoeur G, et al. High-grade ovarian cancer associated H/ACA snoRNAs promote cancer cell proliferation and survival. NAR Cancer. 2022;4(1):zcab050. Epub 20220114 PubMed PMID: 35047824; PubMed Central IDPMC8759569. doi: 10.1093/narcan/zcab050.
- Barros-Silva D, Klavert J, Jenster G, et al. The role of OncosnoRNAs and ribosomal RNA 2’-O-methylation in cancer. RNA Biol. 2021;18(sup1):61–74. Epub 20211114 PubMed PMID: 34775914; PubMed Central IDPMC8677010 doi: 10.1080/15476286.2021.1991167
- Cavaillé J, Buiting K, Kiefmann M, et al. Identification of brain-specific and imprinted small nucleolar RNA genes exhibiting an unusual genomic organization. Proc Nat Acad Sci. 2000;97(26):14311–14316. doi: 10.1073/pnas.250426397
- Jorjani H, Kehr S, Jedlinski DJ, et al. An updated human snoRNAome. Nucleic Acids Res. 2016;44(11):5068–5082. Epub 2016/05/14 PubMed PMID: 27174936; PubMed Central ID PMC4914119. doi: 10.1093/nar/gkw386.
- Boivin V, Deschamps-Francoeur G, Couture S, et al. Simultaneous sequencing of coding and noncoding RNA reveals a human transcriptome dominated by a small number of highly expressed noncoding genes. RNA. 2018;24(7):950–965. doi: 10.1261/rna.064493.117
- McCann KL, Kavari SL, Burkholder AB, et al. H/ACA snoRNA levels are regulated during stem cell differentiation. Nucleic Acids Res. 2020;48(15):8686–8703. PubMed PMID: 32710630; PubMed Central IDPMC7470967. doi: 10.1093/nar/gkaa612
- Warner WA, Spencer DH, Trissal M, et al. Expression profiling of snoRnas in normal hematopoiesis and AML. Blood Adv. 2018;2(2):151–163. Epub 2018/01/25 PubMed PMID: 29365324; PubMed Central IDPMC5787869 doi: 10.1182/bloodadvances.2017006668
- Lykke-Andersen S, Chen Y, Ardal BR, et al. Human nonsense-mediated RNA decay initiates widely by endonucleolysis and targets snoRNA host genes. Genes Dev. 2014;28(22):2498–2517. Epub 2014/11/19 PubMed PMID: 25403180; PubMed Central IDPMC4233243. doi: 10.1101/gad.246538.114
- Talross GJS, Deryusheva S, Gall JG. Stable lariats bearing a snoRNA (slb-snoRNA) in eukaryotic cells: a level of regulation for guide RNAs. Proc Nat Acad Sci. 2021;118(45):e2114156118. doi: 10.1073/pnas.2114156118
- Fafard-Couture É, Jacques P, Scott MS. Motif conservation, stability, and host gene expression are the main drivers of snoRNA expression across vertebrates. Genome Res. 2023;33(4):525–540. Epub 20230418 PubMed PMID: 37072185; PubMed Central IDPMC10234308. doi: 10.1101/gr.277483.122
- Harismendy O. Genome-wide location of yeast RNA polymerase III transcription machinery. EMBO J. 2003;22(18):4738–4747. doi: 10.1093/emboj/cdg466
- Guffanti E, Ferrari R, Preti M, et al. A minimal promoter for TFIIIC-dependent in vitro transcription of snoRNA and tRNA genes by RNA polymerase III. J Biol Chem. 2006;281(33):23945–23957. doi: 10.1074/jbc.m513814200
- Grzechnik P, Gdula MR, Proudfoot NJ. Pcf11 orchestrates transcription termination pathways in yeast. Genes Dev. 2015;29(8):849–861. Epub 2015/04/17 PubMed PMID: 25877920; PubMed Central IDPMC4403260. doi: 10.1101/gad.251470.114
- Steinmetz EJ, Conrad NK, Brow DA, et al. RNA-binding protein Nrd1 directs poly(A)-independent 3’-end formation of RNA polymerase II transcripts. Nature. 2001;413(6853):327–331. Epub 2001/09/21 PubMed PMID: 11565036 doi: 10.1038/35095090
- Han Z, Jasnovidova O, Haidara N, et al. Termination of non-coding transcription in yeast relies on both an RNA Pol II CTD interaction domain and a CTD-mimicking region in Sen1. EMBO J. 2020;39(7):e101548. Epub 20200228 PubMed PMID: 32107786; PubMed Central IDPMC7110113. doi: 10.15252/embj.2019101548
- Han Z, Libri D, Porrua O. Biochemical characterization of the helicase Sen1 provides new insights into the mechanisms of non-coding transcription termination. Nucleic Acids Res. 2017;45(3):1355–1370. PubMed PMID: 28180347; PubMed Central ID PMC5388409. doi: 10.1093/nar/gkw1230.
- Porrua O, Boudvillain M, Libri D. Transcription termination: variations on common themes. Trends Genet. 2016;32(8):508–522. Epub 20160628 PubMed PMID: 27371117. doi: 10.1016/j.tig.2016.05.007.
- Porrua O, Libri D. Transcription termination and the control of the transcriptome: why, where and how to stop. Nat Rev Mol Cell Biol. 2015;16(3):190–202. doi: 10.1038/nrm3943
- Giacometti S, Benbahouche NEH, Domanski M, et al. Mutually exclusive CBC-Containing complexes contribute to RNA fate. Cell Rep. 2017;18(11):2635–2650. doi: 10.1016/j.celrep.2017.02.046
- Wolin SL, Maquat LE. Cellular RNA surveillance in health and disease. Science. 2019;366(6467):822–827. Epub 20191114 PubMed PMID: 31727827; PubMed Central ID PMC6938259. doi: 10.1126/science.aax2957.
- Vasiljeva L, Buratowski S. Nrd1 interacts with the nuclear exosome for 3´ processing of RNA polymerase II transcripts. Molecular Cell. 2006;21(2):239–248. doi: 10.1016/j.molcel.2005.11.028
- Vasiljeva L, Kim M, Mutschler H, et al. The Nrd1–Nab3–Sen1 termination complex interacts with the Ser5-phosphorylated RNA polymerase II C-terminal domain. Nat Struct Mol Biol. 2008;15(8):795–804. doi: 10.1038/nsmb.1468
- Skourti-Stathaki K, Proudfoot NJ, Gromak N. Human senataxin resolves RNA/DNA hybrids formed at transcriptional pause sites to promote Xrn2-dependent termination. Molecular Cell. 2011;42(6):794–805. PubMed PMID: 21700224; PubMed Central ID PMC3145960. doi: 10.1016/j.molcel.2011.04.026.
- Porrua O, Libri D. A bacterial-like mechanism for transcription termination by the Sen1p helicase in budding yeast. Nat Struct Mol Biol. 2013;20(7):884–891. doi: 10.1038/nsmb.2592
- Barillà D, Lee BA, Proudfoot NJ. Cleavage/Polyadenylation factor IA associates with the carboxyl-terminal domain of RNA polymerase II in Saccharomyces cerevisiae. Proc Nat Acad Sci. 2001;98(2):445–450. doi: 10.1073/pnas.98.2.445
- Boulon S, Verheggen C, Jady BE, et al. PHAX and CRM1 are required sequentially to transport U3 snoRNA to nucleoli. Molecular Cell. 2004;16(5):777–787. PubMed PMID: 15574332. doi: 10.1016/j.molcel.2004.11.013
- Hallais M, Pontvianne F, Andersen PR, et al. CBC–ARS2 stimulates 3´-end maturation of multiple RNA families and favors cap-proximal processing. Nat Struct Mol Biol. 2013;20(12):1358–1366. doi: 10.1038/nsmb.2720
- Andersen PR, Domanski M, Kristiansen MS, et al. The human cap-binding complex is functionally connected to the nuclear RNA exosome. Nat Struct Mol Biol. 2013;20(12):1367–1376. doi: 10.1038/nsmb.2703
- Hirose T, Ideue T, Nagai M, et al. A spliceosomal intron binding protein, IBP160, links position-dependent assembly of intron-encoded box C/D snoRNP to pre-mRNA splicing. Molecular Cell. 2006;23(5):673–684. doi: 10.1016/j.molcel.2006.07.011
- De I, Bessonov S, Hofele R, et al. The RNA helicase Aquarius exhibits structural adaptations mediating its recruitment to spliceosomes. Nat Struct Mol Biol. 2015;22(2):138–144. doi: 10.1038/nsmb.2951
- Petfalski E, Dandekar T, Henry Y, et al. Processing of the precursors to small nucleolar RNAs and rRnas requires common components. Mol Cell Biol. 1998;18(3):1181–1189. doi: 10.1128/mcb.18.3.1181
- Ghazal G, Ge D, Gervais-Bird J, et al. Genome-wide prediction and analysis of yeast RNase III-Dependent snoRNA processing signals. Mol Cell Biol. 2005;25(8):2981–2994. doi: 10.1128/mcb.25.8.2981-2994.2005
- Ooi SL, Samarsky DA, Fournier MJ, et al. Intronic snoRNA biosynthesis in Saccharomyces cerevisiae depends on the lariat-debranching enzyme: Intron length effects and activity of a precursor snoRNA. RNA. 1998;4(9):1096–1110. doi: 10.1017/s1355838298980785
- Mohanta A, Chakrabarti K. Dbr1 functions in mRNA processing, intron turnover and human diseases. Biochimie. 2021;180:134–142. Epub 20201008 PubMed PMID: 33038423. doi: 10.1016/j.biochi.2020.10.003
- Colau G, Thiry M, Leduc V, et al. The small nucle(ol)ar RNA cap trimethyltransferase is required for ribosome synthesis and intact nucleolar morphology. Mol Cell Biol. 2004;24(18):7976–7986. doi: 10.1128/mcb.24.18.7976-7986.2004
- Girard C, Verheggen C, Neel H, et al. Characterization of a short isoform of human Tgs1 hypermethylase associating with small nucleolar ribonucleoprotein core proteins and produced by limited proteolytic processing. J Biol Chem. 2008;283(4):2060–2069. doi: 10.1074/jbc.m704209200
- Chanfreau G, Legrain P, Jacquier A. Yeast RNase III as a key processing enzyme in small nucleolar RNAs metabolism. J Mol Biol. 1998;284(4):975–988. PubMed PMID: 9837720. doi: 10.1006/jmbi.1998.2237.
- Lee CY, Lee A, Chanfreau G. The roles of endonucleolytic cleavage and exonucleolytic digestion in the 5´-end processing of S. cerevisiae box C/D snoRNAs. RNA. 2003;9(11):1362–1370. doi: 10.1261/rna.5126203
- Grzechnik P, Szczepaniak SA, Dhir S, et al. Nuclear fate of yeast snoRNA is determined by co-transcriptional Rnt1 cleavage. Nat Commun. 2018;9(1). doi: 10.1038/s41467-018-04094-y
- Proudfoot NJ. Transcriptional termination in mammals: stopping the RNA polymerase II juggernaut. Science. 2016;352(6291):aad9926–aad. doi: 10.1126/science.aad9926
- Miki TS, Großhans H. The multifunctional RNase XRN2. Biochem Soc Trans. 2013;41(4):825–830. PubMed PMID: 23863139. doi: 10.1042/BST20130001.
- Ogami K, Chen Y, Manley J. RNA surveillance by the nuclear RNA exosome: mechanisms and significance. Noncoding RNA. 2018;4(1):8. doi: 10.3390/ncrna4010008
- Davidson L, Francis L, Cordiner RA, et al. Rapid depletion of DIS3, EXOSC10, or XRN2 reveals the immediate impact of exoribonucleolysis on nuclear RNA metabolism and transcriptional control. Cell Rep. 2019;26(10):2779–91.e5. doi: 10.1016/j.celrep.2019.02.012
- Szczepińska T, Kalisiak K, Tomecki R, et al. DIS3 shapes the RNA polymerase II transcriptome in humans by degrading a variety of unwanted transcripts. Genome Res. 2015;25(11):1622–1633. doi: 10.1101/gr.189597.115
- Costello JL, Stead JA, Feigenbutz M, et al. The C-terminal region of the exosome-associated protein Rrp47 is specifically required for box C/D small nucleolar RNA 3´-maturation. J Biol Chem. 2011;286(6):4535–4543. doi: 10.1074/jbc.m110.162826
- Mitchell P, Petfalski E, Houalla R, et al. Rrp47p is an exosome-associated protein required for the 3´ processing of stable RNAs. Mol Cell Biol. 2003;23(19):6982–6992. doi: 10.1128/mcb.23.19.6982-6992.2003
- Lubas M, Christensen SM, Kristiansen SM, et al. Interaction profiling identifies the human nuclear exosome Targeting complex. Molecular Cell. 2011;43(4):624–637. doi: 10.1016/j.molcel.2011.06.028
- Fasken MB, Leung SW, Banerjee A, et al. Air1 Zinc Knuckles 4 and 5 and a conserved IWRXY motif are critical for the function and integrity of the Trf4/5-Air1/2-Mtr4 polyadenylation (TRAMP) RNA quality control complex. J Biol Chem. 2011;286(43):37429–37445. doi: 10.1074/jbc.m111.271494
- Berndt H, Harnisch C, Rammelt C, et al. Maturation of mammalian H/ACA box snoRNAs: PAPD5-dependent adenylation and PARN-dependent trimming. RNA. 2012;18(5):958–972. doi: 10.1261/rna.032292.112
- Lubas M, Andersen RP, Schein A, et al. The human nuclear exosome Targeting complex is loaded onto newly synthesized RNA to direct early ribonucleolysis. Cell Rep. 2015;10(2):178–192. doi: 10.1016/j.celrep.2014.12.026
- Falk S, Finogenova K, Melko M, et al. Structure of the RBM7–ZCCHC8 core of the NEXT complex reveals connections to splicing factors. Nat Commun. 2016;7(1):13573. doi: 10.1038/ncomms13573
- Kufel J, Allmang C, Chanfreau G, et al. Precursors to the U3 small nucleolar RNA lack small nucleolar RNP proteins but are stabilized by La binding. Mol Cell Biol. 2000;20(15):5415–5424. doi: 10.1128/mcb.20.15.5415-5424.2000
- Son A, Park J-E, Kim VN. PARN and TOE1 constitute a 3´ end maturation Module for nuclear non-coding RNAs. Cell Rep. 2018;23(3):888–898. doi: 10.1016/j.celrep.2018.03.089
- Nagarajan VK, Jones CI, Newbury SF, et al. XRN 5‘→3’ exoribonucleases: structure, mechanisms and functions. Biochim Biophys Acta. 2013;1829(6–7):590–603. Epub 20130319 PubMed PMID: 23517755; PubMed Central ID: PMC3742305. doi: 10.1016/j.bbagrm.2013.03.005
- Massenet S, Bertrand E, Verheggen C. Assembly and trafficking of box C/D and H/ACA snoRnps. RNA Biol. 2017;14(6):680–692. doi: 10.1080/15476286.2016.1243646
- Qu L-H, Henras A, Lu Y-J, et al. Seven novel methylation guide small nucleolar RNAs are processed from a common polycistronic transcript by Rat1p and RNase III in yeast. Mol Cell Biol. 1999;19(2):1144–1158. doi: 10.1128/mcb.19.2.1144
- Chanfreau G. Processing of a dicistronic small nucleolar RNA precursor by the RNA endonuclease Rnt1. EMBO J. 1998;17(13):3726–3737. doi: 10.1093/emboj/17.13.3726
- Van Hoof A, Lennertz P, Parker R. Yeast exosome mutants accumulate 3´-extended polyadenylated forms of U4 small nuclear RNA and small nucleolar RNAs. Mol Cell Biol. 2000;20(2):441–452. doi: 10.1128/mcb.20.2.441-452.2000
- Allmang C, Kufel J, Chanfreau G, et al. Functions of the exosome in rRNA, snoRNA and snRNA synthesis. EMBO J. 1999;18(19):5399–5410. PubMed PMID: 10508172; PubMed Central IDPMC1171609. doi: 10.1093/emboj/18.19.5399
- Delan-Forino C, Schneider C, Tollervey D, et al. Transcriptome-wide analysis of alternative routes for RNA substrates into the exosome complex. PLoS Genet. 2017;13(3):e1006699. doi: 10.1371/journal.pgen.1006699
- Grzechnik P, Kufel J. Polyadenylation linked to transcription termination directs the processing of snoRNA precursors in yeast. Molecular Cell. 2008;32(2):247–258. PubMed PMID: 18951092; PubMed Central ID PMC2593888. doi: 10.1016/j.molcel.2008.10.003.
- LaCava J, Houseley J, Saveanu C, et al. RNA degradation by the exosome is promoted by a nuclear polyadenylation complex. Cell. 2005;121(5):713–724. PubMed PMID: 15935758. doi: 10.1016/j.cell.2005.04.029
- Delan-Forino C, Spanos C, Rappsilber J, et al. Substrate specificity of the TRAMP nuclear surveillance complexes. Nat Commun. 2020;11(1). doi: 10.1038/s41467-020-16965-4
- Schuch B, Feigenbutz M, Makino DL, et al. The exosome‐binding factors Rrp6 and Rrp47 form a composite surface for recruiting the Mtr4 helicase. EMBO J. 2014;33(23):2829–2846. doi: 10.15252/embj.201488757
- Schmidt K, Butler JS. Nuclear RNA surveillance: role of TRAMP in controlling exosome specificity. Wiley Interdiscip Rev RNA. 2013;4(2):217–231. doi: 10.1002/wrna.1155
- Falaleeva M, Stamm S. Processing of snoRNAs as a new source of regulatory non-coding RNAs. BioEssays. 2013;35(1):46–54. doi: 10.1002/bies.201200117
- Watkins NJ, Leverette RD, Xia L, et al. Elements essential for processing intronic U14 snoRNA are located at the termini of the mature snoRNA sequence and include conserved nucleotide boxes C and D. RNA. 1996;2(2):118–133. PubMed PMID: 8601279; PubMed Central ID. PMC1369357
- Kim J-W. Human RNA lariat debranching enzyme cDNA complements the phenotypes of Saccharomyces cerevisiae dbr1 and schizosaccharomyces pombe dbr1 mutants. Nucleic Acids Res. 2000;28(18):3666–3673. doi: 10.1093/nar/28.18.3666
- Tycowski KT, Shu MD, Steitz JA. A small nucleolar RNA is processed from an intron of the human gene encoding ribosomal protein S3. Genes Dev. 1993;7(7a):1176–1190. doi: 10.1101/gad.7.7a.1176
- Kiss T, Filipowicz W. Small nucleolar RNAs encoded by introns of the human cell cycle regulatory gene RCC1. EMBO J. 1993;12(7):2913–2920. doi: 10.1002/j.1460-2075.1993.tb05953.x
- Kiss T, Filipowicz W. Exonucleolytic processing of small nucleolar RNAs from pre-mRNA introns. Genes Dev. 1995;9(11):1411–1424. doi: 10.1101/gad.9.11.1411
- Renzi F, Caffarelli E, Laneve P, et al. The structure of the endoribonuclease XendoU: from small nucleolar RNA processing to severe acute respiratory syndrome coronavirus replication. Proc Nat Acad Sci. 2006;103(33):12365–12370. doi: 10.1073/pnas.0602426103
- Schmitzová J, Cretu C, Dienemann C, et al. Structural basis of catalytic activation in human splicing. Nature. 2023;617(7962):842–850. Epub 20230510 PubMed PMID: 37165190. doi: 10.1038/s41586-023-06049-w.
- Ideue T, Sasaki YTF, Hagiwara M, et al. Introns play an essential role in splicing-dependent formation of the exon junction complex. Genes Dev. 2007;21(16):1993–1998. doi: 10.1101/gad.1557907
- Huynh TN, Parker R .The PARN, TOE1, and USB1 RNA deadenylases and their roles in non-coding RNA regulation. J Biol Chem. 2023:105139. Epub 20230804 PubMed PMID: 37544646. doi:10.1016/j.jbc.2023.105139.
- Nguyen D, Grenier St-Sauveur V, Bergeron D, et al. A polyadenylation-dependent 3’ end maturation pathway is required for the synthesis of the human telomerase RNA. Epub 20151125 PubMed PMID: 26628368 Cell Rep. 2015;13(10):2244–2257. doi: 10.1016/j.celrep.2015.11.003
- Gerstberger S, Meyer C, Benjamin-Hong S, et al. The conserved RNA exonuclease Rexo5 is required for 3´ end maturation of 28S rRNA, 5S rRNA, and snoRNAs. Cell Rep. 2017;21(3):758–772. doi: 10.1016/j.celrep.2017.09.067
- Scott MS, Ono M, Yamada K, et al. Human box C/D snoRNA processing conservation across multiple cell types. Nucleic Acids Res. 2012;40(8):3676–3688. Epub 20111222 PubMed PMID: 22199253; PubMed Central ID: PMC3333852. doi: 10.1093/nar/gkr1233
- Scott MS, Avolio F, Ono M, et al. Human miRNA precursors with box H/ACA snoRNA features. PLoS comput Biol. 2009;5(9):e1000507. doi: 10.1371/journal.pcbi.1000507
- Wajahat M, Bracken CP, Orang A. Emerging functions for snoRNAs and snoRNA-Derived fragments. Int J Mol Sci. 2021;22(19):10193. Epub 20210922PubMed PMID: 34638533; PubMed 1665 Central IDPMC8508363. doi: 10.3390/ijms221910193.
- Abel Y, Rederstorff M. snoRNAs and the emerging class of sdRnas: multifaceted players in oncogenesis. Biochimie. 2019;164:17–21. Epub 20190509 PubMed PMID: 31078583. doi: 10.1016/j.biochi.2019.05.006
- Liu Y, DeMario S, He K, et al. Splicing inactivation generates hybrid mRNA-snoRNA transcripts targeted by cytoplasmic RNA decay. Proc Natl Acad Sci U S A. 2022;119(31):e2202473119. Epub 20220725 PubMed PMID: 35878033; PubMed Central IDPMC9351541. doi: 10.1073/pnas.2202473119
- Li Z, Ender C, Meister G, et al. Extensive terminal and asymmetric processing of small RNAs from rRnas, snoRNAs, snRnas, and tRnas. Nucleic Acids Res. 2012;40(14):6787–6799. Epub 20120409 20120409PubMed PMID: 22492706; PubMed Central IDPMC3413118. doi: 10.1093/nar/gks307.
- Wu H, Yin Q-F, Luo Z, et al. Unusual processing generates SPA LncRNAs that sequester multiple RNA binding proteins. Molecular Cell. 2016;64(3):534–548. doi: 10.1016/j.molcel.2016.10.007
- Shi J, Zhou T, Chen Q. Exploring the expanding universe of small RNAs. Nat Cell Biol. 2022;24(4):415–423. doi: 10.1038/s41556-022-00880-5
- Kawaji H, Nakamura M, Takahashi Y, et al. Hidden layers of human small RNAs. BMC Genomics. 2008;9(1):157. doi: 10.1186/1471-2164-9-157
- Kishore S, Khanna A, Zhang Z, et al. The snoRNA MBII-52 (SNORD 115) is processed into smaller RNAs and regulates alternative splicing. Hum Mol Genet. 2010;19(7):1153–1164. Epub 20100106 PubMed PMID: 20053671; PubMed Central IDPMC2838533. doi: 10.1093/hmg/ddp585
- Dupuis-Sandoval F, Poirier M, Scott MS. The emerging landscape of small nucleolar RNAs in cell biology. Wiley Interdiscip Rev RNA. 2015;6(4):381–397. Epub 2015/04/17 PubMed PMID: 25879954; PubMed Central ID: PMC4696412. doi: 10.1002/wrna.1284
- Ono M, Scott MS, Yamada K, et al. Identification of human miRNA precursors that resemble box C/D snoRNAs. Nucleic Acids Res. 2011;39(9):3879–3891. Epub 20110118 PubMed PMID: 21247878; PubMed Central IDPMC3089480. doi: 10.1093/nar/gkq1355
- Ender C, Krek A, Friedländer MR, et al. A human snoRNA with MicroRNA-Like functions. Molecular Cell. 2008;32(4):519–528. doi: 10.1016/j.molcel.2008.10.017
- Taft RJ, Glazov EA, Lassmann T, et al. Small RNAs derived from snoRNAs. RNA. 2009;15(7):1233–1240. doi: 10.1261/rna.1528909
- Macias S, Plass M, Stajuda A, et al. DGCR8 HITS-CLIP reveals novel functions for the microprocessor. Nat Struct Mol Biol. 2012;19(8):760–766. doi: 10.1038/nsmb.2344
- Brameier M, Herwig A, Reinhardt R, et al. Human box C/D snoRNAs with miRNA like functions: expanding the range of regulatory RNAs. Nucleic Acids Res. 2011;39(2):675–686. Epub 20100915 PubMed PMID: 20846955; PubMed Central IDPMC3025573. doi: 10.1093/nar/gkq776
- Zhong F, Zhou N, Wu K, et al. A snoRNA-derived piRNA interacts with human interleukin-4 pre-mRNA and induces its decay in nuclear exosomes. Nucleic Acids Res. 2015;43(21):10474–10491. Epub 20150923 PubMed PMID: 26405199 PubMed Central ID: PMC4666397. doi: 10.1093/nar/gkv954
- Lafontaine DLJ. Noncoding RNAs in eukaryotic ribosome biogenesis and function. Nat Struct Mol Biol. 2015;22(1):11–19. doi: 10.1038/nsmb.2939
- Mleczko AM, Machtel P, Walkowiak M, et al. Levels of sdRnas in cytoplasm and their association with ribosomes are dependent upon stress conditions but independent from snoRNA expression. Sci Rep. 2019;9(1). doi: 10.1038/s41598-019-54924-2
- Sahoo T, Del Gaudio D, German JR, et al. Prader-Willi phenotype caused by paternal deficiency for the HBII-85 C/D box small nucleolar RNA cluster. Nature Genet. 2008;40(6):719–721. doi: 10.1038/ng.158
- Duker AL, Ballif BC, Bawle EV, et al. Paternally inherited microdeletion at 15q11.2 confirms a significant role for the SNORD116 C/D box snoRNA cluster in Prader–Willi syndrome. Eur J Hum Genet. 2010;18(11):1196–1201. doi: 10.1038/ejhg.2010.102
- Cavaillé J. Box C/D small nucleolar RNA genes and the Prader-Willi syndrome: a complex interplay. Wiley Interdiscip Rev RNA. 2017;8(4):e1417. doi: 10.1002/wrna.1417
- Yin Q-F, Yang L, Zhang Y, et al. Long noncoding RNAs with snoRNA ends. Molecular Cell. 2012;48(2):219–230. doi: 10.1016/j.molcel.2012.07.033
- Zacchini F, Venturi G, De Sanctis V, et al. Human dyskerin binds to cytoplasmic H/ACA-box-containing transcripts affecting nuclear hormone receptor dependence. Genome Bio. 2022;23(1). doi: 10.1186/s13059-022-02746-3
- Villa T, Ceradini F, Bozzoni I. Identification of a novel element required for processing of intron-encoded box C/D small nucleolar RNAs in Saccharomyces cerevisiae. Mol Cell Biol. 2000;20(4):1311–1320. PubMed PMID: 10648617; PubMed Central ID: PMC85272. doi: 10.1128/mcb.20.4.1311-1320.2000.
- Darzacq X, Kiss T. Processing of intron-encoded box C/D small nucleolar RNAs lacking a 5‘,3’-terminal stem structure. Mol Cell Biol. 2000;20(13):4522–4531. PubMed PMID: 10848579; PubMed Central IDPMC85834. doi: 10.1128/mcb.20.13.4522-4531.2000
- Schlotter F, Mérouani S, Flayac J, et al. Proteomic analyses reveal new features of the box H/ACA RNP biogenesis. Nucleic Acids Res. 2023;51(7):3357–3374. PubMed PMID: 36869663; PubMed Central ID PMC10123114. doi: 10.1093/nar/gkad129.
- Bizarro J, Charron C, Boulon S, et al. Proteomic and 3D structure analyses highlight the C/D box snoRNP assembly mechanism and its control. J Cell Bio. 2014;207(4):463–480. Epub 2014/11/19 PubMed PMID: 25404746; PubMed Central IDPMC4242836. doi: 10.1083/jcb.201404160
- Boulon SV, Marmier-Gourrier N, Pradet-Balade BRR, et al. The Hsp90 chaperone controls the biogenesis of L7Ae RNPs through conserved machinery. J Cell Bio. 2008;180(3):579–595. doi: 10.1083/jcb.200708110
- Bizarro J, Dodre M, Huttin A, et al. NUFIP and the HSP90/R2TP chaperone bind the SMN complex and facilitate assembly of U4-specific proteins. Nucleic Acids Res. 2015;43(18):8973–8989. Epub 2015/08/16 PubMed PMID: 26275778; PubMed Central ID PMC4605303. doi: 10.1093/nar/gkv809.
- Boulon S, Pradet-Balade B, Verheggen C, et al. HSP90 and its R2TP/Prefoldin-like cochaperone are involved in the cytoplasmic assembly of RNA polymerase II. Molecular Cell. 2010;39(6):912–924. doi: 10.1016/j.molcel.2010.08.023
- Lynham J, Houry WA. The role of Hsp90-R2TP in macromolecular complex assembly and Stabilization. Biomolecules. 2022;12(8):1045. doi: 10.3390/biom12081045
- Zhao R, Davey M, Hsu Y-C, et al. Navigating the chaperone network: an integrative map of physical and genetic interactions mediated by the Hsp90 chaperone. Cell. 2005;120(5):715–727. doi: 10.1016/j.cell.2004.12.024
- Houry WA, Bertrand E, Coulombe B. The PAQosome, an R2TP-Based chaperone for quaternary structure formation. Trends Biochem Sci. 2018;43(1):4–9. Epub 20171205 PubMed PMID: 29203338. doi: 10.1016/j.tibs.2017.11.001.
- Cloutier P, Poitras C, Durand M, et al. R2TP/Prefoldin-like component RUVBL1/RUVBL2 directly interacts with ZNHIT2 to regulate assembly of U5 small nuclear ribonucleoprotein. Nat Commun. 2017;8(1): doi: 10.1038/ncomms15615
- Klein DJ. The kink-turn: a new RNA secondary structure motif. EMBO J. 2001;20(15):4214–4221. doi: 10.1093/emboj/20.15.4214
- Watkins NJ, Segault V, Charpentier B, et al. A common core RNP structure shared between the small nucleolar box C/D RNPs and the spliceosomal U4 snRNP. Cell. 2000;103(3):457–466. Epub 2000/11/18 PubMed PMID: 11081632. doi: 10.1016/s0092-8674(00)00137-9
- Hirose T, Steitz JA. Position within the host intron is critical for efficient processing of box C/D snoRNAs in mammalian cells. Proc Natl Acad Sci USA. 2001;98(23):12914–12919. Epub 20011023 PubMed PMID: 11606788; PubMed Central IDPMC60799. doi: 10.1073/pnas.231490998
- Huang L, Ashraf S, Wang J, et al. Control of box C/D snoRNP assembly by N(6)-methylation of adenine. EMBO Rep. 2017;18(9):1631–1645. Epub 20170616 PubMed PMID: 28623187; PubMed Central ID: PMC5579392. doi: 10.15252/embr.201743967
- Rothe B, Saliou JM, Quinternet M, et al. Protein Hit1, a novel box C/D snoRNP assembly factor, controls cellular concentration of the scaffolding protein Rsa1 by direct interaction. Nucleic Acids Res. 2014;42(16):10731–10747. Epub 2014/08/30 PubMed PMID: 25170085; PubMed Central ID PMC4176330. doi: 10.1093/nar/gku612.
- Yu G, Zhao Y, Tian S, et al. Yeast R2TP interacts with extended Termini of Client protein Nop58p. Sci Rep. 2019;9(1):20228. Epub 20191227 PubMed PMID: 31882871; PubMed Central ID PMC6934851. doi: 10.1038/s41598-019-56712-4.
- Kakihara Y, Makhnevych T, Zhao L, et al. Nutritional status modulates box C/D snoRNP biogenesis by regulated subcellular relocalization of the R2TP complex. Genome Biol. 2014;15(7):404. Epub 2014/07/26 PubMed PMID: 25060708; PubMed Central ID: PMC4165372. doi: 10.1186/s13059-014-0404-4
- Abel Y, Paiva ACF, Bizarro J, et al. NOPCHAP1 is a PAQosome cofactor that helps loading NOP58 on RUVBL1/2 during box C/D snoRNP biogenesis. Nucleic Acids Res. 2021;49(2):1094–1113. PubMed PMID: 33367824; PubMed Central ID: PMC7826282. doi: 10.1093/nar/gkaa1226
- Bragantini B, Tiotiu D, Rothé B, et al. Functional and structural insights of the Zinc-finger HIT protein family members involved in box C/D snoRNP biogenesis. J Mol Biol. 2016;428(11):2488–2506. doi: 10.1016/j.jmb.2016.04.028
- Verheggen C, Pradet-Balade B, snoRnps BE. ZNHIT proteins and the R2TP pathway. Oncotarget. 2015;6(39):41399–41400. Epub 2015/12/02 PubMed PMID: 26623726; PubMed Central IDPMC4747161 doi: 10.18632/oncotarget.6388
- Cloutier P, Poitras C, Durand M, et al. R2TP/Prefoldin-like component RUVBL1/RUVBL2 directly interacts with ZNHIT2 to regulate assembly of U5 small nuclear ribonucleoprotein. Nat Commun. 2017;8(1):15615. PubMed PMID: 28561026; PubMed Central ID: PMC5460035. doi: 10.1038/ncomms15615.
- Khoshnevis S, Dreggors RE, Hoffmann TFR, et al. A conserved Bcd1 interaction essential for box C/D snoRNP biogenesis. J Biol Chem. 2019;294(48):18360–18371. Epub 20190919 PubMed PMID: 31537647; PubMed Central IDPMC6885627. doi: 10.1074/jbc.RA119.010222
- McKeegan KS, Debieux CM, Watkins NJ. Evidence that the AAA+ proteins TIP48 and TIP49 bridge interactions between 15.5K and the related NOP56 and NOP58 proteins during box C/D snoRNP biogenesis. Mol Cell Biol. 2009;29(18):4971–4981. Epub 2009/07/22 PubMed PMID: 19620283; PubMed Central ID: PMC2738292. doi: 10.1128/mcb.00752-09.
- Paul A, Tiotiu D, Bragantini B, et al. Bcd1p controls RNA loading of the core protein Nop58 during C/D box snoRNP biogenesis. RNA. 2019;25(4):496–506. Epub 2019/02/01 PubMed PMID: 30700579; PubMed Central ID: PMC6426285. doi: 10.1261/rna.067967.118
- Richard P, Kiss AM, Darzacq X, et al. Cotranscriptional recognition of human intronic box H/ACA snoRNAs occurs in a splicing-independent manner. Mol Cell Biol. 2006;26(7):2540–2549. PubMed PMID: 16537900; PubMed Central IDPMC1430331 doi: 10.1128/mcb.26.7.2540-2549.2006
- Fatica A, Dlakić M, Tollervey D. Naf1 p is a box H/ACA snoRNP assembly factor. RNA. 2002;8(12):1502–1514. PubMed PMID: 12515383; PubMed Central ID. doi: 10.1017/S1355838202022094
- Yang PK, Hoareau C, Froment C, et al. Cotranscriptional recruitment of the pseudouridylsynthetase Cbf5p and of the RNA binding protein Naf1p during H/ACA snoRNP assembly. Mol Cell Biol. 2005;25(8):3295–3304. PubMed PMID: 15798213; PubMed Central IDPMC1069627 doi: 10.1128/mcb.25.8.3295-3304.2005
- Ghanim GE, Fountain AJ, van Roon AM, et al. Structure of human telomerase holoenzyme with bound telomeric DNA. Nature. 2021;593(7859):449–453. Epub 20210421 PubMed PMID: 33883742; PubMed Central IDPMC7610991 doi: 10.1038/s41586-021-03415-4
- Charpentier B, Muller S, Branlant C. Reconstitution of archaeal H/ACA small ribonucleoprotein complexes active in pseudouridylation. Nucleic Acids Res. 2005;33(10):3133–3144. Epub 20050602 PubMed PMID: 15933208; PubMed Central IDPMC1142404. doi: 10.1093/nar/gki630
- Baker DL, Youssef OA, Chastkofsky MIR, et al. RNA-Guided RNA modification: functional organization of the archaeal H/ACA RNP. Genes Dev. 2005;19(10):1238–1248. doi: 10.1101/gad.1309605
- Yang PK, Rotondo G, Porras T, et al. The Shq1p.Naf1p complex is required for box H/ACA small nucleolar ribonucleoprotein particle biogenesis. J Biol Chem. 2002;277(47):45235–45242. Epub 20020911 PubMed PMID: 12228251 doi: 10.1074/jbc.M207669200
- Dez C, Noaillac-Depeyre J, Caizergues-Ferrer M, et al. Naf1p, an essential nucleoplasmic factor specifically required for accumulation of box H/ACA small nucleolar RNPs. Mol Cell Biol. 2002;22(20):7053–7065. PubMed PMID: 12242285; PubMed Central ID: PMC139812. doi: 10.1128/mcb.22.20.7053-7065.2002
- Hoareau-Aveilla C, Bonoli M, Caizergues-Ferrer M, et al. hNaf1 is required for accumulation of human box H/ACA snoRNP, scaRnps, and telomerase. RNA. 2006;12(5):832–840. Epub 20060406 PubMed PMID: 16601202; PubMed Central ID PMC1440901. doi: 10.1261/rna.2344106.
- Walbott H, Machado-Pinilla R, Liger D, et al. The H/ACA RNP assembly factor SHQ1 functions as an RNA mimic. Genes Dev. 2011;25(22):2398–2408. PubMed PMID: 22085966; PubMed Central ID: PMC3222905. doi: 10.1101/gad.176834.111
- Machado-Pinilla R, Liger D, Leulliot N, et al. Mechanism of the AAA+ ATPases pontin and reptin in the biogenesis of H/ACA RNPs. RNA. 2012;18(10):1833–1845. Epub 2012/08/28 PubMed PMID: 22923768; PubMed Central ID PMC3446707. doi: 10.1261/rna.034942.112.
- Grozdanov PN, Roy S, Kittur N, et al. SHQ1 is required prior to NAF1 for assembly of H/ACA small nucleolar and telomerase RNPs. RNA. 2009;15(6):1188–1197. Epub 20090421 PubMed PMID: 19383767; PubMed Central ID PMC2685518. doi: 10.1261/rna.1532109.
- Venteicher AS, Meng Z, Mason PJ, et al. Identification of ATPases pontin and reptin as telomerase components essential for holoenzyme assembly. Cell. 2008;132(6):945–957. doi: 10.1016/j.cell.2008.01.019
- Leulliot N, Godin KS, Hoareau-Aveilla C, et al. The box H/ACA RNP assembly factor Naf1p contains a domain homologous to Gar1p mediating its interaction with Cbf5p. J Mol Biol. 2007;371(5):1338–1353. Epub 20070616 PubMed PMID: 17612558. doi: 10.1016/j.jmb.2007.06.031
- Darzacq X, Kittur N, Roy S, et al. Stepwise RNP assembly at the site of H/ACA RNA transcription in human cells. J Cell Bio. 2006;173(2):207–218. Epub 20060417 PubMed PMID: 16618814; PubMed Central ID: PMC2063812. doi: 10.1083/jcb.200601105
- Li S, Duan J, Li D, et al. Structure of the Shq1-Cbf5-Nop10-Gar1 complex and implications for H/ACA RNP biogenesis and dyskeratosis congenita. EMBO J. 2011;30(24):5010–5020. Epub 20111125 PubMed PMID: 22117216; PubMed Central IDPMC3242979. doi: 10.1038/emboj.2011.427
- Jády BE, Bertrand E, Kiss T. Human telomerase RNA and box H/ACA scaRnas share a common Cajal body-specific localization signal. J Cell Bio. 2004;164(5):647–652. Epub 20040223 PubMed PMID: 14981093; PubMed Central IDPMC2172171. doi: 10.1083/jcb.200310138
- Bizarro J, Bhardwaj A, Smith S, et al. Nopp140-mediated concentration of telomerase in Cajal bodies regulates telomere length. Mol Biol Cell. 2019;30(26):3136–3150. Epub 20191030 PubMed PMID: 31664887; PubMed Central ID PMC6938241. doi: 10.1091/mbc.E19-08-0429.
- Yang Y, Isaac C, Wang C, et al. Conserved composition of mammalian box H/ACA and box C/D small nucleolar ribonucleoprotein particles and their interaction with the common factor Nopp140. Mol Biol Cell. 2000;11(2):567–577. PubMed PMID: 10679015; PubMed Central ID PMC14794. doi: 10.1091/mbc.11.2.567.
- Isaac C, Yang Y, Meier UT. Nopp140 functions as a molecular link between the nucleolus and the coiled bodies. J Cell Bio. 1998;142(2):319–329. PubMed PMID: 9679133; PubMed Central IDPMC2133063. doi: 10.1083/jcb.142.2.319
- Pellizzoni L, Baccon J, Charroux B, et al. The survival of motor neurons (SMN) protein interacts with the snoRNP proteins fibrillarin and GAR1. Curr Biol. 2001;11(14):1079–1088. PubMed PMID: 11509230. doi: 10.1016/s0960-9822(01)00316-5
- Whitehead SE, Jones KW, Zhang X, et al. Determinants of the interaction of the spinal muscular atrophy disease protein SMN with the dimethylarginine-modified box H/ACA small nucleolar ribonucleoprotein GAR1. J Biol Chem. 2002;277(50):48087–48093. Epub 20020919 PubMed PMID: 12244096. doi: 10.1074/jbc.M204551200
- Bachand F, Boisvert FM, Côté J, et al. The product of the survival of motor neuron (SMN) gene is a human telomerase-associated protein. Mol Biol Cell. 2002;13(9):3192–3202. PubMed PMID: 12221125; PubMed Central ID: PMC124152. doi: 10.1091/mbc.e02-04-0216
- Cao R, Wang L, Wang H, et al. Role of histone H3 lysine 27 methylation in Polycomb-group silencing. Science. 2002;298(5595):1039–1043. Epub 20020926 PubMed PMID: 12351676. doi: 10.1126/science.1076997
- Margueron R, Reinberg D. The Polycomb complex PRC2 and its mark in life. Nature. 2011;469(7330):343–349. PubMed PMID: 21248841; PubMed Central ID PMC3760771. doi: 10.1038/nature09784.
- Kim J, Lee Y, Lu X, et al. Polycomb- and methylation-independent roles of EZH2 as a transcription activator. Cell Rep. 2018;25(10):2808–20.e4. PubMed PMID: 30517868; PubMed Central IDPMC6342284. doi: 10.1016/j.celrep.2018.11.035
- Li Q, Liu KY, Liu Q, et al. Antihistamine drug ebastine inhibits cancer growth by Targeting Polycomb group protein EZH2. Mol Cancer Ther. 2020;19(10):2023–2033. Epub 20200827 PubMed PMID: 32855270 PubMed Central ID: PMC7541747. doi: 10.1158/1535-7163.Mct-20-0250
- Yi Y, Li Y, Meng Q, et al. A PRC2-independent function for EZH2 in regulating rRNA 2’-O methylation and IRES-dependent translation. Nat Cell Biol. 2021;23(4):341–354. Epub 20210401 PubMed PMID: 33795875 PubMed Central ID: PMC8162121. doi: 10.1038/s41556-021-00653-6
- Erales J, Marchand V, Panthu B, et al. Evidence for rRNA 2’-O-methylation plasticity: control of intrinsic translational capabilities of human ribosomes. Proc Natl Acad Sci USA. 2017;114(49):12934–12939. Epub 2017/11/22 PubMed PMID: 29158377 PubMed Central ID: PMC5724255. doi: 10.1073/pnas.1707674114
- Khoshnevis S, Dreggors-Walker RE, Marchand V, et al. Ribosomal RNA 2´- O -methylations regulate translation by impacting ribosome dynamics. Proc Natl Acad Sci, USA. 2022;119(12):e2117334119. Epub 2022/03/17 PubMed PMID: 35294285; PubMed Central ID PMC8944910. doi: 10.1073/pnas.2117334119.
- Pieretti M, Zhang FP, Fu YH, et al. Absence of expression of the FMR-1 gene in fragile X syndrome. Cell. 1991;66(4):817–822. PubMed PMID: 1878973. doi: 10.1016/0092-8674(91)90125-i
- D’Souza MN, Gowda NKC, Tiwari V, et al. FMRP interacts with C/D box snoRNA in the nucleus and regulates ribosomal RNA methylation. iScience. 2018;9:399–411. doi: 10.1016/j.isci.2018.11.007
- Simsek D, Tiu GC, Flynn RA, et al. The mammalian ribo-interactome reveals ribosome functional diversity and heterogeneity. Cell. 2017;169(6):1051–65.e18. doi: 10.1016/j.cell.2017.05.022
- Chen E, Sharma R, Manjuli S, et al. Fragile X mental retardation protein regulates translation by binding directly to the ribosome. Molecular Cell. 2014;54(3):407–417. doi: 10.1016/j.molcel.2014.03.023
- Sévigny M, Bourdeau Julien I, Venkatasubramani JP, et al. FUS contributes to mTOR-dependent inhibition of translation. J Biol Chem. 2020;295(52):18459–18473. doi: 10.1074/jbc.ra120.013801
- Gawade K, Plewka P, Häfner SJ, et al. FUS regulates a subset of snoRNA expression and modulates the level of rRNA modifications. Sci Rep. 2023;13(1). doi: 10.1038/s41598-023-30068-2
- Sama RRK, Ward CL, Bosco DA. Functions of FUS/TLS from DNA repair to stress response: implications for ALS. ASN Neuro. 2014;6(4):175909141454447. doi: 10.1177/1759091414544472
- Mitterer V, Pertschy B. RNA folding and functions of RNA helicases in ribosome biogenesis. RNA Biol. 2022;19(1):781–810. doi: 10.1080/15476286.2022.2079890
- Leeds NB, Small EC, Hiley SL, et al. The splicing factor Prp43p, a DEAH box ATPase, functions in ribosome biogenesis. Mol Cell Biol. 2006;26(2):513–522. doi: 10.1128/mcb.26.2.513-522.2006
- Bohnsack MT, Martin R, Granneman S, et al. Prp43 bound at different sites on the pre-rRNA performs distinct functions in ribosome synthesis. Mol Cell. 2009;36(4):583–592. Epub 2009/11/28 PubMed PMID: 19941819; PubMed Central IDPMC2806949. doi: 10.1016/j.molcel.2009.09.039
- Aquino R, Gerald R, Krogh N, et al. RNA helicase-mediated regulation of snoRNP dynamics on pre-ribosomes and rRNA 2´-O-methylation. Nucleic Acids Res. 2021;49(7):4066–4084. doi: 10.1093/nar/gkab159
- Sardana R, Liu X, Granneman S, et al. The DEAH-box helicase Dhr1 dissociates U3 from the pre-rRNA to promote formation of the Central pseudoknot. PLoS Biol. 2015;13(2):e1002083. doi: 10.1371/journal.pbio.1002083
- Choudhury P, Hackert P, Memet I, et al. The human RNA helicase DHX37 is required for release of the U3 snoRNP from pre-ribosomal particles. RNA Biol. 2019;16(1):54–68. doi: 10.1080/15476286.2018.1556149
- Khoshnevis S, Askenasy I, Johnson MC, et al. The DEAD-box protein Rok1 orchestrates 40S and 60S ribosome assembly by promoting the release of Rrp5 from pre-40S ribosomes to allow for 60S maturation. PLoS Biol. 2016;14(6):e1002480. Epub 20160609 PubMed PMID: 27280440; PubMed Central IDPMC4900678. doi: 10.1371/journal.pbio.1002480
- Bohnsack MT, Kos M, Tollervey D. Quantitative analysis of snoRNA association with pre‐ribosomes and release of snR30 by Rok1 helicase. EMBO Rep. 2008;9(12):1230–1236. doi: 10.1038/embor.2008.184
- Brüning L, Hackert P, Martin R, et al. RNA helicases mediate structural transitions and compositional changes in pre-ribosomal complexes. Nat Commun. 2018;9(1). doi: 10.1038/s41467-018-07783-w
- Gnanasundram SV, Kos-Braun IC, Koš M. At least two molecules of the RNA helicase Has1 are simultaneously present in pre-ribosomes during ribosome biogenesis. Nucleic Acids Res. 2019;47(20):10852–10864. PubMed PMID: 31511893; PubMed Central IDPMC6846684. doi: 10.1093/nar/gkz767
- Hasler D, Meister G, Fischer U. Stabilize and connect: the role of LARP7 in nuclear non-coding RNA metabolism. RNA Biol. 2021;18(2):290–303. doi: 10.1080/15476286.2020.1767952
- Stavraka C, Blagden S. The La-related proteins, a family with connections to cancer. Biomolecules. 2015;5(4):2701–2722. Epub 2015/10/27 PubMed PMID: 26501340; PubMed Central ID PMC4693254. doi: 10.3390/biom5042701.
- Egloff S, Vitali P, Tellier M, et al. The 7SK snRNP associates with the little elongation complex to promote snRNA gene expression. EMBO J. 2017;36(7):934–948. doi: 10.15252/embj.201695740
- Hasler D, Meduri R, Bąk M, et al. The Alazami syndrome-associated protein LARP7 guides U6 small nuclear RNA modification and contributes to splicing robustness. Molecular Cell. 2020;77(5):1014–31.e13. doi: 10.1016/j.molcel.2020.01.001
- Wang X, Li Z-T, Yan Y, et al. LARP7-mediated U6 snRNA modification ensures splicing fidelity and spermatogenesis in mice. Molecular Cell. 2020;77(5):999–1013.e6. doi: 10.1016/j.molcel.2020.01.002
- Zhu M-H, John S, Berg M, et al. Functional association of Nmi with Stat5 and Stat1 in IL-2- and IFN γ-mediated signaling. Cell. 1999;96(1):121–130. doi: 10.1016/s0092-8674(00)80965-4
- Samant RS, Shevde LA. NMI and EMT. Oncoscience. 2014;1(7):476–477. Epub 2015/01/17 PubMed PMID: 25594046; PubMed Central ID PMC4278318. doi: 10.18632/oncoscience.55.
- Pruitt HC, Metge BJ, Weeks SE, et al. Conditional knockout of N-Myc and STAT interactor disrupts normal mammary development and enhances metastatic ability of mammary tumors. Oncogene. 2018;37(12):1610–1623. doi: 10.1038/s41388-017-0037-7
- Metge BJ, Mitra A, Chen D, et al. N-Myc and STAT interactor regulates autophagy and chemosensitivity in breast cancer cells. Sci Rep. 2015;5(1):11995. doi: 10.1038/srep11995
- Metge BJ, Kammerud SC, Pruitt HC, et al. Hypoxia re-programs 2´-O-Me modifications on ribosomal RNA. iScience. 2021;24(1):102010. doi: 10.1016/j.isci.2020.102010
- Sharma S, Yang J, Watzinger P, et al. Yeast Nop2 and Rcm1 methylate C2870 and C2278 of the 25S rRNA, respectively. Nucleic Acids Res. 2013;41(19):9062–9076. Epub 20130802 PubMed PMID: 23913415; PubMed Central IDPMC3799443. doi: 10.1093/nar/gkt679
- Du E, Li J, Sheng F, et al. A pan-cancer analysis reveals genetic alterations, molecular mechanisms, and clinical relevance of m(5) C regulators. Clin Transl Med. 2020;10(5):e180. Epub 2020/10/01 PubMed PMID: 32997404; PubMed Central IDPMC7507430. doi: 10.1002/ctm2.180
- Liao H, Gaur A, McConie H, et al. Human NOP2/NSUN1 regulates ribosome biogenesis through non-catalytic complex formation with box C/D snoRnps. Nucleic Acids Res. 2022;50(18):10695–10716. PubMed PMID: 36161484; PubMed Central ID PMC9561284. doi: 10.1093/nar/gkac817.
- Box JK, Paquet N, Adams MN, et al. Nucleophosmin: from structure and function to disease development. BMC Mol Biol. 2016;17(1): doi: 10.1186/s12867-016-0073-9
- Zarka J, Short NJ, Kanagal-Shamanna R, et al. Nucleophosmin 1 mutations in acute Myeloid Leukemia. Genes. 2020;11(6):649. doi: 10.3390/genes11060649
- Sharma N, Liesveld JL. NPM 1 mutations in AML-The landscape in 2023. Cancers (Basel). 2023;15(4). Epub 2023/02/26 PubMed PMID: 36831522; PubMed Central ID PMC9954410. doi: 10.3390/cancers15041177
- Grisendi S, Mecucci C, Falini B, et al. Nucleophosmin and cancer. Nat Rev Cancer. 2006;6(7):493–505. doi: 10.1038/nrc1885
- Chiarella S, De Cola A, Scaglione GL, et al. Nucleophosmin mutations alter its nucleolar localization by impairing G-quadruplex binding at ribosomal DNA. Nucleic Acids Res. 2013;41(5):3228–3239. Epub 2013/01/19 PubMed PMID: 23328624; PubMed Central IDPMC3597674. doi: 10.1093/nar/gkt001
- Nachmani D, Bothmer AH, Grisendi S, et al. Germline NPM1 mutations lead to altered rRNA 2´-O-methylation and cause dyskeratosis congenita. Nature Genet. 2019;51(10):1518–1529. doi: 10.1038/s41588-019-0502-z
- Krishnakumar R, Kraus WL. The PARP side of the nucleus: molecular actions, physiological outcomes, and clinical targets. Molecular Cell. 2010;39(1):8–24. doi: 10.1016/j.molcel.2010.06.017
- Gupte R, Liu Z, Kraus WL. Parps and ADP-ribosylation: recent advances linking molecular functions to biological outcomes. Genes Dev. 2017;31(2):101–126. doi: 10.1101/gad.291518.116
- Bitler BG, Watson ZL, Wheeler LJ, et al. PARP inhibitors: clinical utility and possibilities of overcoming resistance. Gynecol Oncol. 2017;147(3):695–704. doi: 10.1016/j.ygyno.2017.10.003
- Weil MK, Chen AP. PARP inhibitor treatment in ovarian and breast cancer. Curr Probl Cancer. 2011;35(1):7–50. doi: 10.1016/j.currproblcancer.2010.12.002
- Kim D-S, Camacho CV, Nagari A, et al. Activation of PARP-1 by snoRNAs controls ribosome biogenesis and Cell growth via the RNA helicase DDX21. Molecular Cell. 2019;75(6):1270–85.e14. doi: 10.1016/j.molcel.2019.06.020
- Ayala YM, Zago P, D’Ambrogio A, et al. Structural determinants of the cellular localization and shuttling of TDP-43. J Cell Sci. 2008;121(22):3778–3785. doi: 10.1242/jcs.038950
- Izumikawa K, Nobe Y, Ishikawa H, et al. TDP-43 regulates site-specific 2’-O-methylation of U1 and U2 snRnas via controlling the Cajal body localization of a subset of C/D scaRnas. Nucleic Acids Res. 2019;47(5):2487–2505. PubMed PMID: 30759234; PubMed Central IDPMC6412121. doi: 10.1093/nar/gkz086
- Sreedharan J, Blair IP, Tripathi VB, et al. TDP-43 mutations in familial and sporadic amyotrophic lateral sclerosis. Science. 2008;319(5870):1668–1672. doi: 10.1126/science.1154584
- Neumann M, Sampathu DM, Kwong LK, et al. Ubiquitinated TDP-43 in frontotemporal lobar degeneration and amyotrophic lateral sclerosis. Science. 2006;314(5796):130–133. PubMed PMID: 17023659. doi: 10.1126/science.1134108
- Fourmann JB, Tauchert MJ, Ficner R, et al. Regulation of Prp43-mediated disassembly of spliceosomes by its cofactors Ntr1 and Ntr2. Nucleic Acids Res. 2017;45(7):4068–4080. PubMed PMID: 27923990; PubMed Central IDPMC5397206. doi: 10.1093/nar/gkw1225
- Tanaka N, Aronova A, Schwer B. Ntr1 activates the Prp43 helicase to trigger release of lariat-intron from the spliceosome. Genes Dev. 2007;21(18):2312–2325. doi: 10.1101/gad.1580507
- Yoshimoto R, Kataoka N, Okawa K, et al. Isolation and characterization of post-splicing lariat-intron complexes. Nucleic Acids Res. 2009;37(3):891–902. Epub 20081222 PubMed PMID: 19103666; PubMed Central ID: PMC2647322. doi: 10.1093/nar/gkn1002
- Christian H, Hofele RV, Urlaub H, et al. Insights into the activation of the helicase Prp43 by biochemical studies and structural mass spectrometry. Nucleic Acids Res. 2014;42(2):1162–1179. Epub 20131027 PubMed PMID: 24165877; PubMed Central IDPMC3902948. doi: 10.1093/nar/gkt985
- Duchemin A, O’Grady T, Hanache S, et al. DHX15-independent roles for TFIP11 in U6 snRNA modification, U4/U6.U5 tri-snRNP assembly and pre-mRNA splicing fidelity. Nat Commun. 2021;12(1):6648. Epub 20211117 PubMed PMID: 34789764 PubMed Central ID: PMC8599867. doi: 10.1038/s41467-021-26932-2
- Battle DJ, Kasim M, Yong J, et al. The SMN complex: An assembly Machine for RNPs. Cold Spring Harb Symp Quant Biol. 2006;71:313–320. doi: 10.1101/sqb.2006.71.001
- Singh RN, Howell MD, Ottesen EW, et al. Diverse role of survival motor neuron protein. Biochim Biophys Acta, Gene Regul Mech. 2017;1860(3):299–315. Epub 20170115 PubMed PMID: 28095296; PubMed Central IDPMC5325804. doi: 10.1016/j.bbagrm.2016.12.008
- Arbab M, Matuszek Z, Kray KM, et al. Base editing rescue of spinal muscular atrophy in cells and in mice. Science. 2023;380(6642):eadg6518. Epub 2023/03/31 PubMed PMID: 36996170 PubMed Central ID: PMC10270003. doi: 10.1126/science.adg6518
- Lefebvre S, Burglen L, Reboullet S, et al. Identification and characterization of a spinal muscular atrophy-determining gene. Cell. 1995;80(1):155–165. Epub 1995/01/13 PubMed PMID: 7813012. doi: 10.1016/0092-8674(95)90460-3
- Jones KW, Gorzynski K, Hales CM, et al. Direct interaction of the spinal muscular atrophy disease protein SMN with the small nucleolar RNA-associated protein fibrillarin. J Biol Chem. 2001;276(42):38645–38651. Epub 20010816 PubMed PMID: 11509571. doi: 10.1074/jbc.M106161200
- Baldini L, Robert A, Charpentier B, et al. Phylogenetic and Molecular analyses identify SNORD116 targets involved in the Prader-Willi syndrome. Mol Biol Evol. 2022;39(1). PubMed PMID: 34893870; PubMed Central ID PMC8789076. doi: 10.1093/molbev/msab348
- Mcfadden EJ, Baserga SJ. U8 variants on the brain: a small nucleolar RNA and human disease. RNA Biol. 2022;19(1):412–418. doi: 10.1080/15476286.2022.2048563
- Dreggors-Walker RE, Cohen LN, Khoshnevis S, et al. Studies of mutations of assembly factor Hit1 in budding yeast suggest translation defects as the molecular basis for PEHO syndrome. J Biol Chem. 2022;298(9):102261. Epub 20220714 PubMed PMID: 35843310; PubMed Central IDPMC9418376. doi: 10.1016/j.jbc.2022.102261
- Savage SA, Niewisch MR. Dyskeratosis congenita and related telomere biology disorders. In: Adam M, Mirzaa G, Pagon R, Wallace S, Bean L Gripp K, Eds. et al., editors. GeneReviews(®). Seattle (WA). Seattle: University of Washington. Copyright © 1993–2023, University of Washington, Seattle. GeneReviews is a registered 2235 trademark of the University of Washington, Seattle. All rights reserved.; 1993.
- Benyelles M, O’Donohue MF, Kermasson L, et al. NHP2 deficiency impairs rRNA biogenesis and causes pulmonary fibrosis and Høyeraal-Hreidarsson syndrome. PubMed PMID: 31985013 Hum Mol Genet. 2020;29(6):907–922. doi: 10.1093/hmg/ddaa011
- AlHargan A, AlMuhaizea MA, Almass R, et al. SHQ1-associated neurodevelopmental disorder: report of the first homozygous variant in unrelated patients and review of the literature. Hum Genome Var. 2023;10(1):7. Epub 20230222 PubMed PMID: 36810590; PubMed Central ID: PMC9944922. doi: 10.1038/s41439-023-00234-z
- Sleiman S, Marshall AE, Dong X, et al. Compound heterozygous variants in SHQ1 are associated with a spectrum of neurological features, including early-onset dystonia. Hum Mol Genet. 2022;31(4):614–624. PubMed PMID: 34542157. doi: 10.1093/hmg/ddab247
- Chi CS, Tsai CR, Lee HF. Biallelic SHQ1 variants in early infantile hypotonia and paroxysmal dystonia as the leading manifestation. Hum Genet. 2023;142(8):1029–1041. Epub 20230227 PubMed PMID: 36847845. doi: 10.1007/s00439-023-02533-5.
- Watson CN, Belli A, Di Pietro V. Small non-coding RNAs: new class of biomarkers and potential therapeutic targets in neurodegenerative disease. Front Genet. 2019;10:364. Epub 20190426 PubMed PMID: 31080456; PubMed Central ID: PMC6497742. doi: 10.3389/fgene.2019.00364.
- Liang J, Wen J, Huang Z, et al. Small nucleolar RNAs: Insight into their function in cancer. Front Oncol. 2019;9:587. Epub 20190709 PubMed PMID: 31338327; PubMed Central ID PMC6629867. doi: 10.3389/fonc.2019.00587
- Zhang D, Zhou J, Gao J, et al. Targeting snoRNAs as an emerging method of therapeutic development for cancer. Am J Cancer Res. 2019;9(8):1504–1516. Epub 20190801. PubMed PMID: 31497339; PubMed Central ID.
- Marcel V, Ghayad SE, Belin S, et al. p53 acts as a safeguard of translational control by regulating fibrillarin and rRNA methylation in cancer. Cancer Cell. 2013;24(3):318–330. PubMed PMID: 24029231 PubMed Central ID: PMC7106277. doi: 10.1016/j.ccr.2013.08.013
- Krastev DB, Slabicki M, Paszkowski-Rogacz M, et al. A systematic RNAi synthetic interaction screen reveals a link between p53 and snoRNP assembly. Nat Cell Biol. 2011;13(7):809–818. Epub 20110605 PubMed PMID: 21642980. doi: 10.1038/ncb2264
- Simeonova I, Jaber S, Draskovic I, et al. Mutant mice lacking the p53 C-terminal domain model telomere syndromes. Cell Rep. 2013;3(6):2046–2058. Epub 20130613 PubMed PMID: 23770245. doi: 10.1016/j.celrep.2013.05.028
- Marchand V, Blanloeil-Oillo F, Helm M, et al. Illumina-based RiboMethSeq approach for mapping of 2’-O-Me residues in RNA. Nucleic Acids Res. 2016;44(16):e135. Epub 20160614 PubMed PMID: 27302133; PubMed Central IDPMC5027498. doi: 10.1093/nar/gkw547
- Marchand V, Bourguignon-Igel V, Helm M, et al. Analysis of pseudouridines and other RNA modifications using HydraPsiSeq protocol. Methods. 2022;203:383–391. Epub 20210901 PubMed PMID: 34481083. doi: 10.1016/j.ymeth.2021.08.008
- Krogh N, Jansson MD, Hafner SJ, et al. Profiling of 2’-O-Me in human rRNA reveals a subset of fractionally modified positions and provides evidence for ribosome heterogeneity. Nucleic Acids Res. 2016;44(16):7884–7895. Epub 2016/06/04 PubMed PMID: 27257078; PubMed Central ID: PMC5027482. doi: 10.1093/nar/gkw482
- Birkedal U, Christensen-Dalsgaard M, Krogh N, et al. Profiling of ribose methylations in RNA by high-throughput sequencing. Angew Chem (Int Ed In English). 2015;54(2):451–455. Epub 2014/11/25 PubMed PMID: 25417815. doi: 10.1002/anie.201408362
- Dai Q, Moshitch-Moshkovitz S, Han D, et al. Nm-seq maps 2’-O-methylation sites in human mRNA with base precision. Nat Methods. 2017;14(7):695–698. Epub 2017/05/16 PubMed PMID: 28504680; PubMed Central IDPMC5712428. doi: 10.1038/nmeth.4294
- Carlile TM, Rojas-Duran MF, Zinshteyn B, et al. Pseudouridine profiling reveals regulated mRNA pseudouridylation in yeast and human cells. Nature. 2014;515(7525):143–146. Epub 2014/09/06 PubMed PMID: 25192136; PubMed Central ID: PMC4224642. doi: 10.1038/nature13802
- Schwartz S, Bernstein DA, Mumbach MR, et al. Transcriptome-wide mapping reveals widespread dynamic-regulated pseudouridylation of ncRNA and mRNA. Cell. 2014;159(1):148–162. Epub 2014/09/16 PubMed PMID: 25219674; PubMed Central ID PMC4180118. doi: 10.1016/j.cell.2014.08.028.
- Ruggero D, Grisendi S, Piazza F, et al. Dyskeratosis congenita and cancer in mice deficient in ribosomal RNA modification. Science. 2003;299(5604):259–262. PubMed PMID: 12522253. doi: 10.1126/science.1079447
- Belin S, Beghin A, Solano-Gonzalez E, et al. Dysregulation of ribosome biogenesis and translational capacity is associated with tumor progression of human breast cancer cells. PLoS One. 2009;4(9):e7147. Epub 20090925 PubMed PMID: 19779612; PubMed Central IDPMC2744998. doi: 10.1371/journal.pone.0007147
- Jansson MD, Häfner SJ, Altinel K, et al. Regulation of translation by site-specific ribosomal RNA methylation. Nat Struct Mol Biol. 2021;28(11):889–899. Epub 20211110 PubMed PMID: 34759377. doi: 10.1038/s41594-021-00669-4
- Morais P, Adachi H, Yu YT. Spliceosomal snRNA epitranscriptomics. Front Genet. 2021;12:652129. Epub 2021/03/20 PubMed PMID: 33737950; PubMed Central ID PMC7960923. doi: 10.3389/fgene.2021.652129
- Marcel V, Kielbassa J, Marchand V, et al. Ribosomal RNA 2‘O-methylation as a novel layer of inter-tumour heterogeneity in breast cancer. NAR Cancer. 2020;2(4):zcaa036. Epub 20201222 PubMed PMID: 34316693 PubMed Central ID: PMC8210124. doi: 10.1093/narcan/zcaa036
- Holohan B, Kim W, Lai T-P, et al. Impaired telomere maintenance in Alazami syndrome patients with LARP7 deficiency. BMC Genomics. 2016;17(S9). doi: 10.1186/s12864-016-3093-4
- Bellodi C, Mcmahon M, Contreras A, et al. H/ACA small RNA dysfunctions in disease reveal key roles for noncoding RNA modifications in hematopoietic stem Cell differentiation. Cell Rep. 2013;3(5):1493–1502. doi: 10.1016/j.celrep.2013.04.030
- Vulliamy T, Beswick R, Kirwan M, et al. Mutations in the telomerase component NHP2 cause the premature ageing syndrome dyskeratosis congenita. Proc Nat Acad Sci. 2008;105(23):8073–8078. doi: 10.1073/pnas.0800042105
- Walne AJ, Vulliamy T, Marrone A, et al. Genetic heterogeneity in autosomal recessive dyskeratosis congenita with one subtype due to mutations in the telomerase-associated protein NOP10. Hum Mol Genet. 2007;16(13):1619–1629. Epub 20070516 PubMed PMID: 17507419; PubMed Central IDPMC2882227. doi: 10.1093/hmg/ddm111
- Wang H, Shi D, Jiang P, et al. SARS-CoV-2 N protein potentiates host NPM1-snoRNA translation machinery to enhance viral replication. Signal Transduct Ther. 2022;7(1): doi: 10.1038/s41392-022-01210-9
- Balogh E, Chandler JC, Varga M, et al. Pseudouridylation defect due to DKC1 and NOP10 mutations causes nephrotic syndrome with cataracts, hearing impairment, and enterocolitis. Epub 2020/06/20 PubMed PMID: 32554502; PubMed Central ID: PMC7334496 Proc Natl Acad Sci U S A. 2020;117(26):15137–15147. doi: 10.1073/pnas.2002328117
- Kumari K, Groza P, Aguilo F. Regulatory roles of RNA modifications in breast cancer. NAR Cancer. 2021;3(3):zcab036. Epub 20210916 PubMed PMID: 34541538; PubMed Central ID: PMC8445368. doi: 10.1093/narcan/zcab036.
- Pelletier J, Thomas G, Volarević S. Ribosome biogenesis in cancer: new players and therapeutic avenues. Nat Rev Cancer. 2018;18(1):51–63. doi: 10.1038/nrc.2017.104
- Barbieri I, Kouzarides T. Role of RNA modifications in cancer. Nat Rev Cancer. 2020;20(6):303–322. doi: 10.1038/s41568-020-0253-2
- McMahon M, Contreras A, Ruggero D. Small RNAs with big implications: new insights into H/ACA snoRNA function and their role in human disease. Wiley Interdiscip Rev RNA. 2015;6(2):173–189. Epub 20141031 PubMed PMID: 25363811; PubMed Central IDPMC4390053. doi: 10.1002/wrna.1266
- Schaffer JE. Death by lipids: the role of small nucleolar RNAs in metabolic stress. J Biol Chem. 2020;295(25):8628–8635. Epub 20200511 PubMed PMID: 32393576; PubMed Central IDPMC7307203. doi: 10.1074/jbc.AW120.011105