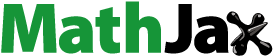
ABSTRACT
Background
Pre-exercise protein consumption does not seem to influence substrate metabolism during exercise compared to fasted exercise, however it is unclear if the protein dose impacts on this effect.
Methods
In a randomized, double-blinded within-subject design trial, healthy, active males and females (n = 15, 25 ± 5 yrs, O2peak: 47.5 ± 8.8 ml/kg/min) completed 1 h of cycling exercise at 60% peak power output 30 min after having consumed either 0, 20, or 40 g of whey protein. Indirect calorimetry was used to measure substrate oxidation during exercise and baseline and post-exercise resting energy expenditure. Blood samples were taken throughout the trials to measure metabolic responses. Free-living food intake post-trial was collected using food diaries.
Results
Fat oxidation rates during exercise did not differ between the three conditions (p = 0.19) with small effect sizes between conditions (Cohen’s dz: 0 vs. 20 g = 0.22, 0 vs. 40 g = 0.47, 20 vs. 40 g = 0.27). Serum insulin was higher in the protein groups vs. 0 g (p < 0.05), whereas non-esterified fatty acids were higher in the 0 g compared to 20 and 40 g (p < 0.05). Glucose was significantly lower after 15 min of exercise in 20 and 40 g vs. 0 g (p = 0.01). Resting energy expenditure was elevated post-exercise (p < 0.001), without an interaction for protein dose (p = 0.90). Post-trial free-living energy intake was not different between conditions (p = 0.31), but 24-h energy intake was significantly higher in 40 vs. 0 g (p = 0.04).
Conclusion
Protein doses up to 40 g do not seem to impair fat oxidation rates during exercise compared to fasted exercise and could be considered as a nutritional strategy for exercising individuals who struggle to include fasted exercise in their training.
1. Introduction
Over recent decades, research into the interaction between exercise and timing of nutrient intake has generated several nutritional strategies to support exercise performance and induce training adaptations. One such strategy related to endurance exercise is called “train-low compete high” to improve fat oxidative capacity by manipulating carbohydrate intake around exercise where training sessions are performed in a carbohydrate-restricted or low-glycogen state [Citation1,Citation2], including in the fasted state [Citation3]. Acutely, fasted exercise promotes fat oxidation in part due to higher availability of plasma non-esterified fatty acids (NEFA) in the blood compared to exercise after a carbohydrate-rich meal [Citation4]. In turn, this leads to increased expression and phosphorylation status of proteins involved in substrate selection, but with limited evidence for increased activation of transcription factors to promote mitochondrial biogenesis [Citation5,Citation6]. Despite the repeatedly demonstrated benefits of training with low carbohydrate availability on fat oxidative capacity in skeletal muscle, there is limited evidence that this strategy translates to improved performance with studies showing both positive [Citation7,Citation8] or no changes [Citation1–3] in performance compared to training with high carbohydrate availability.
Regardless of the inconclusive evidence on the effect of fasted or low-carbohydrate available exercise training on performance, exercising in an overnight fasted state is indeed a strategy frequently applied by endurance athletes, with one survey finding that around 63% of athletes incorporate this in their training program [Citation9]. However, reasons why athletes do not engage in fasted exercise include getting hungry and feeling terrible during the workout. In addition to subjective challenges of fasted training, another disadvantage could be that training with low carbohydrate availability might increase skeletal muscle protein oxidation due to a greater contribution of protein catabolism to energy production [Citation10,Citation11]. Indeed, protein consumption around an acute bout of exercise is needed to induce a positive net protein balance [Citation12] and endurance exercise requires a relatively large quantity of protein to maximize muscle protein synthesis [Citation13]. Thus, it could be speculated that fasted exercise might compromise muscle remodeling if applied consistently whilst not compensated for with appropriate daily protein intake.
One solution to overcome these challenges is the provision of dietary protein before exercise. Although protein is an insulinotropic macronutrient [Citation14] and therefore expected to suppress lipolysis, a few recent investigations into pre-exercise protein ingestion on substrate oxidation demonstrated that NEFA concentrations and subsequently fat oxidation rates were not affected compared to fasted exercise, despite a temporary increase in insulin concentrations following pre-exercise protein consumption [Citation15–18]. Whilst these studies have used casein [Citation18] and whey [Citation15,Citation16] as protein sources or compared casein with whey [Citation17], one unexplored question is whether the quantity of protein consumed influences substrate metabolism. Previous studies have used different pre-exercise feeding patterns including 0.45 g/kg body weight [Citation16], 25 g protein [Citation17] or pre-, during and post-exercise (totaling 50–60 g protein) [Citation15,Citation18]. However, no study to date has directly investigated whether there is a dose-dependent relationship between pre-exercise protein intake and fat oxidation during endurance exercise.
In addition to its effect on substrate metabolism, fasted exercise is often touted as a strategy to support weight loss practices. Indeed, in a recent meta-analysis, Frampton et al. [Citation19] demonstrated that ad libitum and 24-h energy intake was lower after a single session of fasted exercise compared to exercise in the fed state in the absence of providing post-exercise standardized meals. However, pre-exercise meals consisted of a mixed macronutrient profile or carbohydrate-only, and little is known how pre-exercise protein consumption affects free-living energy intake following an acute bout of exercise. Moreover, Frampton et al. [Citation19] also reported that the reduction in energy intake following fasted exercise occurred in conjunction with a decrease in energy expenditure compared to exercise in the fed state, which the authors attribute to higher diet-induced thermogenesis for the fed condition. Interestingly, some studies demonstrated that pre-exercise protein consumption provided higher rates of resting energy expenditure (REE) post-exercise compared to carbohydrates but not to the fasted condition [Citation17,Citation20,Citation21].
Therefore, the aim of this experiment is to investigate the effect of different doses of protein, ingested prior to exercise, on substrate oxidation and metabolic responses during exercise, pre- and post-exercise resting energy expenditure and free-living energy intake following the trial. We hypothesized that fat oxidation during exercise would be maintained during different doses of pre-exercise protein feeding. However, it is hypothesized that there is a dose-dependent increase in REE post-exercise, which also translates to a dose-dependent increase in subsequent energy intake.
2. Methods
2.1. Participants
Participation was open to healthy active male and female adults (18–35 years old). Participants needed to meet the current ACSM activity guidelines of >150 min of vigorous exercise per week [Citation22]. Females were able to participate if they were naturally and regularly menstruating or using monophasic or progestin-only oral contraceptives. Testing for naturally menstruating females took place during their self-reported early or mid-follicular phase. Participants were excluded if they had a known food allergy or intolerance associated with the treatments, were taking medication known to affect protein or lipid metabolism or were suffering from or had previous history of renal, gastrointestinal, or cardiovascular complications or any other contraindication to the study procedures (i.e. musculoskeletal injury). Prior to giving informed consent, participants received an information sheet and a verbal outline of the risks and benefits of taking part in the study. The study was approved by the Newcastle University Ethical Committee (1909/2392).
2.2. Study design
The study was a double-blinded, placebo-controlled within-subject design. Following confirmation of eligibility to partake in the study and signing of informed consent, participants underwent body composition testing and a maximal exercise test to exhaustion on a cycle ergometer to establish peak power output (PPO) and peak oxygen consumption (O2peak) that was used to calculate 60% of PPO. Next, a randomization was executed (www.randomizer.org) to establish the order of treatments over the experimental visits. Randomization and treatment preparation was conducted by a member not involved in data collection and analysis. The randomization key was locked away and only released after statistical analysis was completed. Testing took place between November 2021 and December 2022 in the Newcastle University Sport Centre. Participants were asked to avoid strenuous exercise and alcohol consumption 48 h before the trial days and abstain from caffeine 24 h before the trial. They were asked to record their food intake the day before the trial by use of a written food diary and asked to consume the same diet the day before every subsequent experimental trial. During the experimental trials, participants cycled for 1 h at 60% PPO, 30 min following the ingestion of a beverage containing either 0, 20, or 40 g of protein. Indirect calorimetry measurements and venous blood samples were collected at multiple time points throughout the experimental trials. Time between experimental visits was at least 3 days to allow the body to fully recover and enable the participant to follow pre-trial protocols ().
Figure 1. Overview of the experimental visits. Participants arrived in the morning after an overnight fast. After measuring resting energy expenditure for 15 minutes and drawing a fasted blood sample, participants consumed a 500-mL beverage containing either 0, 20, or 40 g of whey protein in a randomized, cross-over design. Thirty minutes after ingestion, participants cycled for 1 h at 60% of peak power output (PPO).
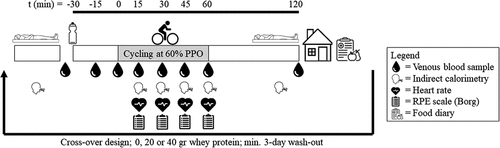
2.3. Body composition and maximal aerobic capacity assessment
Height, weight, and body composition including relative and absolute fat mass and fat-free mass were measured using bio-electrical impedance (mBCA 515, Seca, UK). Next, an incremental ramp test-to-exhaustion on a cycle ergometer (Velotron RacerMate, Seattle) was conducted to measure PPO and O2peak. Following a 3-min warm up at 50 W, power output increased by 1W every 3 s. Participants were asked to cycle at a self-selected cadence but to keep this above 70 rpm. The test was terminated upon volitional exhaustion. Rate of perceived exertion (RPE) using Borg scale and heart rate were measured every 1.5 min. Breath-by-breath measurements were taken throughout the protocol using a metabolic cart (Vyntus CPX, Vyaire, UK) to establish
O2peak, which was calculated as the highest average value over 30 s.
2.4. Experimental protocol
On the morning of the experimental trials, participants arrived at the laboratory after an overnight fast using the least amount of physical activity. Upon arrival, a measurement of resting energy expenditure was taken for 15 min using the Douglas Bag (150 L) system following best practice including a resting period prior to measurement, supine position, accustoming breathing rate for the first 5 min and machine calibration [Citation23]. After this, a cannula (Venflon 20 G, BD Sciences) was inserted into the antecubital vein to obtain a fasted venous blood sample (t = −30 min) and flushed with 3 mL 0.9% saline solution to keep the line patent. Next, the participant ingested one of the three treatment beverages within 3 min and sat upright. The exercise started 30 min after consumption of the treatment, and participants cycled for 60 min at 60% of PPO (Velotron Racermate, Seattle) at a self-selected cadence. If, during the first trial, the exercise intensity was too vigorous, participants were allowed to decrease the exercise intensity to 50% PPO, which was repeated during the subsequent visits. Every 15 min throughout the exercise, expired gases were collected for 1–3 min using Douglas Bag system to analyze substrate oxidation along with heart rate and RPE. Forty-five minutes after exercise, another resting energy expenditure measurement was taken for 15 min whilst the participant was in a supine position. Venous blood samples were collected throughout the trial at t = −15, 0 (start exercise), 15, 30, 45, 60, and 120 min. After each trial, participants were asked to record their food intake using a written food diary for the remainder of the day (~13:00 till sleep). For maximal memory retention, recording details included time, location, product brand, and preparation. Food intake was analyzed by an experienced researcher using MyFitnessPal software [Citation24]. Outcomes were presented as post-trial intake (self-reported) and total 24-h intake (self-reported plus content of treatment beverages as detailed below).
2.5. Protein beverages
The protein beverages contained either 20 or 40 g whey protein hydrolyzate (20 g: 440 kJ, 2/1.5/20 g fat/CHO/PRO; 40 g: 880 kJ, 4/3/40 g fat/CHO/PRO) (Science in Sport, UK), dissolved in 500 mL water and vanilla-flavored or strawberry-flavored drops (MyFlav Drops, MyProtein, UK), whereas the non-caloric placebo only contained the vanilla- or strawberry-flavored drops. The beverages were prepared to be similar in taste and appearance in nontransparent bottles.
2.6. Data analysis
2.6.1. Indirect calorimetry
Fraction of inspired (FiO2 and FiCO2) and expired air (FeO2 and FeCO2) were analyzed together with total expired air volume (Ve) (5200 MiniMP, Servomex). Using standardized equations, O2 and
CO2 were computed. Resting energy expenditure was calculated using Weir’s Equation [Citation25] and averaged to kcal/min. Substrate oxidation (g/min) was calculated using equations for moderate-to-high exercise intensities but not corrected for protein oxidation [Citation26]. Negative values for fat oxidation (RER > 1.0) were corrected to 0.
2.6.2. Blood analysis
Venous blood samples were collected in serum separating vacutainers (BD Sciences, UK) and turned five times before being stored at room temperature for at least 30 min prior to centrifugation at 1500 g at 4°C for 10 min. Serum samples were aliquoted and stored at −80°C until analysis. Serum metabolites were analyzed for glucose, triglycerides, non-esterified fatty acids, and glycerol using commercially available kits (Randox Daytona+, UK) and for lactate (Biosen, EFK Diagnostic). Serum insulin concentrations were analyzed using commercially available enzyme-linked immunosorbent assay kit (Mercodia, Sweden) according to manufacturer’s instructions.
2.7. Statistical analysis
Sample size calculations were based on the primary outcome, fat oxidation, and calculated in G*Power 3.1.9.4. The number of participants required to reduce the probability of a type II error was selected according to a priori power analysis. Power (1-β) was set at 0.80 and two tailed α level set at 0.05. Using a reported mean effect size of 0.41 for fat oxidation between a fasted and protein-fed condition at an exercise intensity above the ventilatory threshold [Citation16], the minimum number of participants required was 12. To account for drop-outs, the number was increased to 15.
Statistical analysis was conducted using the Statistical Package for the Social Sciences software program (IBM SPSS version 28, Armonk, NY). All outcomes were analyzed for normality (Shapiro-Wilk) and sphericity (Mauchly’s). Any non-normally distributed data were log-transformed and where sphericity was violated, a Greenhouse–Geisser correction was applied. REE, substrate oxidation rates, metabolic responses from the blood samples, heart rate, and RPE during exercise were analyzed using two-way repeated measures ANOVA with time and treatment as within-subject variables. Energy and macronutrient intake after each experimental trial was analyzed using one-way repeated measures ANOVA. Where a significant main effect was observed, pairwise comparisons were analyzed using Bonferroni post hoc tests in order to locate specific differences. Cohen’s dz effect sizes (ES) were analyzed between protein doses and interpreted using 0.20, 0.50, and 0.80 as small, moderate, or large effect sizes, respectively [Citation27]. Statistical significance was set at P < 0.05.
3. Results
3.1. Participant characteristics
Sixty people responded to the recruitment information. Following initial contact, 24 did not follow up and 7 declined to participate and 6 did not meet the inclusion criteria. A total of 23 participants completed the screening visit, but 4 withdrew from the study following the screening. A total of 19 were randomized into the study. Four participants dropped out after their first or second trial due to changes in time commitment, resulting in data inclusion of 15 participants. Participant characteristics are presented in . Nine of the participants opted to reduce the exercise intensity to 50% PPO, eight at 30 min and one at 15 min into the exercise after collection of the measurements at the respective time points.
Table 1. Participant characteristics data.
3.2. Exercise substrate oxidation rates and physiological responses
All raw mean data for every 15 min throughout exercise are presented in . Fat oxidation significantly increased over time (p < 0.001) with concomitant decreases in CHO oxidation (p < 0.001). There was no main effect of protein dose on fat oxidation (p = 0.19; Estimated mean difference (95% CI): 0 g vs. 20 g: −0.02 g/min (−0.08, 0.04); ES = 0.22, 0 g vs. 40 g: −0.05 g/min (−0.12, 0.02); ES = 0.47, 20 g vs. 40 g: −0.03 g/min (−0.11, 0.05); ES = 0.27) or a time*protein dose interaction effect (p = 0.44) and similar non-significant effects for CHO oxidation (protein dose: p = 0.63, protein dose*time: p = 0.26). There was no main effect for protein dose on respiratory exchange ratio (RER) (p = 0.08) or interaction effect (p = 0.053), but a main time effect (p < 0.001) indicated a significant decrease in RER throughout the exercise. No main effect was present for RPE for protein dose (p = 0.75) or protein dose*time (p = 0.90), but RPE increased throughout exercise (p = 0.001). All main effects for heart rate were non-significant (p > 0.05)
Table 2. Substrate metabolism, heart rate, and rate of perceived exertion (RPE) responses throughout 1 h of cycling exercise at 60% of peak power output.
3.3. Blood serum responses
Full blood collection for all three experimental visits was completed for n = 9, and results are presented from this sample pool. There was a main effect for protein dose (p = 0.004) on insulin concentrations with differences between 0 g vs. 20 g (p = 0.004; ES = 1.6) and 0 g vs. 40 g (p = 0.036; ES = 1.1), without differences between 20 g vs. 40 g (p = 0.97; ES = 0.35). Insulin was significantly higher at the start of exercise (t = 0) in both 20 g and 40 g compared to 0 g (p < 0.05) but declined in the protein groups (). A main effect for protein dose on NEFA was present (p = 0.002) with significant differences between 0 vs. 20 g (p = 0.04; ES 1.0) and 0 vs. 40 g (p = 0.003; ES = 1.7) but not between 20 vs. 40 g (p = 1.00; ES = 0.15). Throughout the trial, NEFA concentrations in 0 g were significantly higher compared to 20 and 40 g at several time points (). A main time effect was present for triglycerides (p < 0.001) with higher concentrations during the exercise period compared to the baseline sample, but no main effect for protein dose (p = 0.68) or interaction effect (p = 0.87) was present (). A main effect (p = 0.009) was present for protein dose on glycerol concentrations where glycerol was significantly higher in 0 vs. 20 g (p = 0.03; ES = 1.1), but not 0 vs. 40 g (p = 0.098; ES = 0.86) or 20 vs. 40 g (p = 1.00; ES = 0.02). Glycerol concentrations significantly increased throughout the exercise compared to baseline (p < 0.001) and was significantly higher in 0 vs. 20 g at several time points (). Glucose was largely unaffected between protein dose (p = 0.61), but a significant interaction (p < 0.000) was present where after 15 min of exercise both protein groups had a significantly lower glucose concentration compared to 0 g (0 vs. 20 g: p = 0.011; 0 vs. 40 g: p = 0.015) (). No main effect of protein dose (p = 0.88) or protein dose*time interaction (p = 0.92) was present for lactate, but concentrations were significantly higher throughout the exercise period (p = 0.000) ().
Figure 2. Serum (a) insulin, (b) non-esterified fatty acids (NEFA), (c) triglycerides, (d) glycerol, (e) glucose and (f) lactate concentrations following the consumption of either 0, 20, or 40 g of whey protein hydrolysate thirty minutes prior to 1 h of cycling exercise at 60% of peak power output (N = 9). Data are mean ± standard deviation. The grey area indicates the exercising window. a, b and c indicate a significant difference for 40, 20, or 0 g protein respectively compared to the fasted baseline sample (t = −30 min; p < 0.05). # and ‡ denote a significant difference (p < 0.05) between 0 vs. 20 or 0 vs. 40 g, respectively, at the indicated time point.
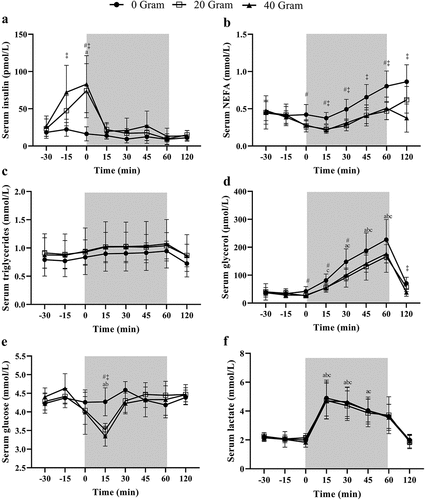
3.4. Resting energy expenditure and post-trial energy intake
A main time effect was present with resting energy expenditure increased after exercise (p < 0.001; ), but no main effect of protein dose (p = 0.41) or protein dose*time interaction (p = 0.91). Mean estimates (95% CI) post-pre differences within conditions were as follows: 0 g: 0.17 kcal/min (−0.01, 0.36) p = 0.06; ES = 0.54, 20 g: 0.21 kcal/min (0.07, 0.34) p = 0.005; ES = 0.90 and 40 g: 0.17 kcal/min (0.04, 0.30) p = 0.016; ES = 0.73, whereas between condition differences were non-significant with trivial effect sizes (0 vs. 20 g: −0.035 kcal/min (−0.28, 0.21); ES = 0.10, 0 vs. 40 g: 0.006 kcal/min (−0.34, 0.35); ES = 0.01 and 20 vs. 40 g: 0.04 kcal/min (−0.19, 0.27); ES = 0.14; p > 0.99 for all contrasts). Thirteen food diaries were returned by participants and analyzed (). Post-trial food intake did not differ between the three protein doses. Mean ± SD post-trial energy intake was 1841 ± 790, 1963 ± 789, and 2099 ± 1001 kcal for 0 g, 20 g, and 40 g respectively (p = 0.31), with mean (95% CI) estimated difference of −122 kcal (−588, 343); ES = 0.20, −258 kcal (−721, 205); ES = 0.42 and −135 kcal (−579, 308); ES = 0.23 between 0 vs. 20 g, 0 vs. 40 g and 20 vs. 40 g respectively. No significant differences were present between protein doses for carbohydrate intake (p = 0.09), protein intake (p = 0.06) and fat intake (p = 0.79). However, following un-blinding of treatment allocation, a significant main effect of protein dose on total 24-h energy intake (p = 0.03) and protein intake (p < 0.001) was found. Mean (95% CI) estimated differences for 24-h energy intake were −226 kcal (−692, 239); p = 0.60, ES = 0.37, −467 kcal (−930, −4); p = 0.04, ES = 0.78 and −240 kcal (−684, 203); p = 0.48, ES = 0.41 for 0 vs. 20 g, 0 vs. 40 g and 20 vs. 40 g, respectively.
Figure 3. Average resting energy expenditure (REE in kcal/min) following a 15-min indirect calorimetry measurement before (pre) and 45 minutes after completion of 1 h of cycling exercise at 60% of peak power output started 30 minutes after consumption of either 0, 20, or 40 g whey protein (post) (N = 14). Bars are mean ± standard deviation. A significant main effect for time was present with increased REE in post vs. Pre (p < 0.05).
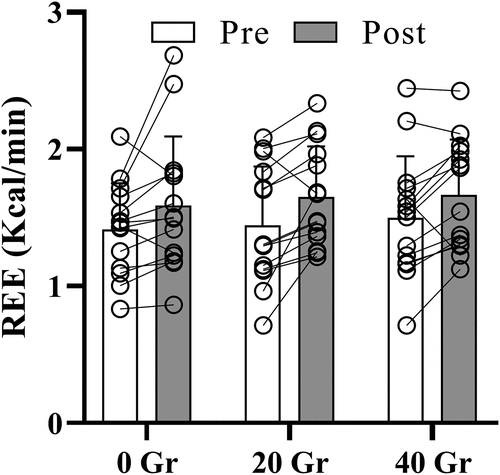
Table 3. Free-living post-trial and total 24-h energy and macronutrient intake.
4. Discussion
The aim of the current study was to investigate whether there was a dose-dependent effect of pre-exercise protein ingestion on fat oxidation rates during exercise. A non-caloric drink, as a fasted exercise condition, was compared against 20 g and 40 g of whey protein hydrolyzate, doses which are frequently associated with optimal muscle anabolic responses to resistance exercise [Citation28,Citation29] and endurance exercise [Citation13]. The primary findings indicate that fat oxidation rates during exercise were not different compared to fasted exercise despite elevated insulin and lower NEFA concentrations following both 20 g and 40 g of protein ingestion 30 min prior to exercise. In addition, resting energy expenditure was increased 45 min post-exercise without any effect of protein dose and no differences in post-trial energy intake, but significant differences in total 24-h energy intake was observed.
Fat oxidation rates during fixed-intensity cycling were not affected by pre-exercise protein ingestion of different quantities compared to fasted exercise. The findings of this study are in line with previous studies [Citation15,Citation17,Citation18,Citation21]. Exercise in the fasted state, whether performed in a low muscle glycogen state or not, is accompanied with higher fat oxidation rates compared to a CHO-fed state, which is primarily due to the absence of insulin’s effect on suppressing mechanisms involved in lipolysis and beta-oxidation [Citation15]. Protein is also an insulinotropic macronutrient, and increased concentrations were observed in both our and previous studies [Citation18], however fat oxidation rates were not impaired. A rapid decrease of insulin following the onset of exercise to baseline levels could provide an explanation for this observation (). However, blood serum NEFA responses were significantly lower throughout the exercise following the consumption of 20 g and 40 g of whey protein compared to 0 g. This is in contrast with earlier studies that demonstrated no differences [Citation15] or a tendency toward different NEFA concentrations between fasted or pre-exercise protein ingestion despite increased insulin [Citation18]. One potential reason for this difference could be the type of protein used. The current study used a whey protein hydrolyzate whereas others used a whey isolate [Citation15] or casein hydrolyzate [Citation30,Citation31]. Protein absorption kinetics are dependent on protein type [Citation32] and fraction, where hydrolyzates are more rapidly absorbed compared to intact proteins, resulting in a larger insulin peak [Citation18]. However, comparing pre-exercise whey with casein has found no difference in fat oxidation rates, but these studies did not investigate insulin and NEFA concentrations [Citation33]. More importantly, in the context of inducing skeletal muscle adaptations, a primary reason why athletes engage in low-CHO availability exercise, Taylor et al. [Citation18] showed that a total of 50 g casein, of which 20 g consumed pre-exercise, induced similar levels of AMPK and PGC-1α activation compared to the fasted state, with similar fat oxidation rates, elevated insulin concentrations but a tendency toward decreased circulating NEFA concentrations in the protein group. As we demonstrated similar effects on insulin concentrations and fat oxidation rates, despite significantly lower NEFA, this points toward evidence that protein consumption up to 40 g of protein prior to exercise might not negatively influence metabolic adaptations associated with fasted exercise, although the absence of skeletal muscle biopsies in the current study does not enable to confirm this. Findings of both acute [Citation34,Citation35] and chronic [Citation1,Citation3,Citation8] interventions provided evidence that exercise in low-CHO available exercise conditions resulted in higher activation of skeletal muscle proteins involved in energy metabolism with a shift toward fat oxidative capacity in chronic adaptations. However, as exercising in a low-glycogen available state seems to enhance amino acid oxidation, resulting in a negative net leg protein balance [Citation10], such nutritional strategy might have negative implications on muscle quality over a prolonged time. Indeed, in a resistance exercise setting, a combination of protein and carbohydrate intake before compared to after resistance exercise resulted in positive net protein balance during the exercise period [Citation36]. No measurements of muscle protein turnover were included in the current study, so this precludes us from making any conclusion if in an endurance-exercise setting similar effects might be observed and this should be a point of focus in future experiments.
Resting energy expenditure was significantly elevated post-exercise, which is a common finding following moderate and high-intensity exercise [Citation33]. We hypothesized that due to diet-induced thermogenesis following different protein doses, the protein groups would have higher post-exercise REE compared to 0 g. However, no significant differences were observed between the different doses, despite a large effect size in pre- to post-exercise change within the 40 g condition but moderate effect sizes for 0 and 20 g. Findings are somewhat similar compared to previous studies, who demonstrated significantly higher REE following pre-exercise protein compared to CHO-fed, but not compared to fasted [Citation17,Citation20,Citation21]. The timing of the post-exercise measurement, might explain some discrepancies. For example, Ratliff et al. [Citation21], using a similar protocol, found differences in REE immediately post-exercise (1.5 h following consumption) but not 60 min after exercise, between pre-exercise CHO and protein intake, but not protein compared to fasted the latter time point more reflective of the time of measurement in the current study. Gieske et al. [Citation17], only including 30 min of exercise, found differences between CHO compared to casein and whey intake immediately post-exercise and a tendency toward significance compared to a fasted condition. Wingfield et al. [Citation20] showed higher REE throughout a 1 h post-exercise period comparing CHO to PRO but did not include a fasted condition. It is therefore possible that the combination of a more rapid absorption of the protein hydrolyzate in our study and the delay in post-exercise REE measurement (~2 h following protein ingestion) failed to capture any effect of diet-induced thermogenesis. Free-living post-trial food intake was unaffected by protein dose, however when nutritional information of the protein treatments was included in the statistical analysis, 24-h energy intake was significantly higher in the 40 g condition compared to the fasted group. These findings corroborate with evidence that 24-h energy intake was influenced by feeding status prior to exercise [Citation19] with similar [Citation37] or higher [Citation38] pre-exercise energy intake of a mixed macronutrient profile, thus we provide new evidence that this response is similar with a protein-only pre-exercise beverage, although caution is warranted due to limited validity of self-reported energy intake using food diaries [Citation39].
4.1. Limitations and future recommendations
Although the study was designed to investigate physiological responses to pre-exercise protein ingestion, it should be noted that the application of the exercise protocol is limited to real-world implementation. The starting exercise intensity was selected at 60% PPO in line with previous research [Citation15], however this exercise intensity can fall in different exercise domains depending on the training status of a participant. Indeed, lactate concentrations during exercise suggested exercising moderate-to-severe exercise domain for a large number of participants and 60% of participants reduced their exercise intensity to 50% PPO. No familiarization was included prior to the experimental visits as no performance elements were involved and during piloting, 60% PPO was successful in a trained cyclist. Since all reductions in PPO occurred in the first experimental visit and repeated in subsequent trials, outcomes will have remained largely unaffected. As fasted exercise is anecdotally performed during low-intensity exercise session or evidence providing reduced interval-based exercise quality in the fasted state [Citation1], incorporation of pre-exercise protein strategies should be carefully evaluated within the context of the training prescription. Recommendations for future studies are to use different exercise intensity prescription, although Rothschild et al. [Citation16] analyzed fat oxidation at different exercise intensities following pre-exercise protein, finding no difference. Moreover, lower exercise intensities might need to be used in both a laboratory and real-life setting as we observed a sharp drop in serum glucose concentrations to hypoglycemic levels at the onset of exercise, likely a combination of peak insulin and a rapid increase in energy demand resulting in an increased glycolytic flux. Although this effect was seen in all participants, it should be noted that metabolic responses were only measured in n = 9 participants which limits the power of these effects. Providing the challenges associated with fasted exercise, including perceptions of hunger and elevated protein oxidation, accumulating evidence seems to suggest that pre-exercise protein intake maintains fat oxidation rates during a single exercise session. It is still unknown how longer-term training with pre-exercise protein intake influences skeletal muscle remodeling and adaptation compared to fasted training as only one study so far looked at this for a 3-week training period [Citation40], hence future studies should further investigate long-term adaptations to pre-exercise protein consumption on adaptations.
5. Conclusion
In summary, the findings in this study showed that there is limited evidence for a dose-dependent effect of pre-exercise protein ingestion of 20 and 40 g, 30 min prior to 1 h of exercise on substrate oxidation, resting energy expenditure and free-living energy intake compared to fasted exercise. As fasted exercise, or exercise with low CHO availability, might enhance metabolic adaptation in skeletal muscle but poses challenges, pre-exercise protein intake at doses up to 40 g could be considered as a nutritional strategy to yield similar effect in exercising individuals who struggle to include fasted exercise into their training, however longer-term training studies will need to investigate this.
Acknowledgments
The authors would like to thank Mr William Pearmain for the randomization procedure and beverage preparation. The authors would like to thank all participants for their time commitment.
Disclosure statement
No potential conflict of interest was reported by the author(s).
Additional information
Funding
References
- Hulston, CJ, Venables, MC, Mann, CH, et al. Training with low muscle glycogen enhances fat metabolism in well-trained cyclists. Med Sci Sports Exerc. 2010 Nov;42(11):2046–910. doi: 10.1249/MSS.0b013e3181dd5070
- Yeo, WK, Paton, CD, Garnham, AP, et al. Skeletal muscle adaptation and performance responses to once a day versus twice every second day endurance training regimens. J Appl Physiol ( 1985). 2008 Nov;105(5):1462–1470.
- Van Proeyen, K, Szlufcik, K, Nielens, H, et al. Beneficial metabolic adaptations due to endurance exercise training in the fasted state. J Appl Physiol. 2011 Jan;110(1):236–245. 1985.
- Vieira, AF, Costa, RR, Macedo, RC, et al. Effects of aerobic exercise performed in fasted v. fed state on fat and carbohydrate metabolism in adults: a systematic review and meta-analysis. Br J Nutr. 2016 Oct;116(7):1153–1164.
- Russell, AP, Hesselink, MK, Lo, SK, et al. Regulation of metabolic transcriptional co-activators and transcription factors with acute exercise. FASEB J. 2005 Jun;19(8):986–988.
- Stocks, B, Dent, JR, Ogden, HB, et al. Postexercise skeletal muscle signaling responses to moderate- to high-intensity steady-state exercise in the fed or fasted state. Am J Physiol Endocrinol Metab. 2019 Feb 1;316(2):E230–E238. doi: 10.1152/ajpendo.00311.2018
- Hansen, AK, Fischer, CP, Plomgaard, P, et al. Skeletal muscle adaptation: training twice every second day vs. training once daily. J Appl Physiol. (1985). 2005 Jan;98(1):93–99. doi: 10.1152/japplphysiol.00163.2004
- Cochran, AJ, Myslik, F, MacInnis, MJ, et al. Manipulating carbohydrate availability between twice-daily sessions of high-intensity interval training over 2 weeks improves time-trial performance. Int J Sport Nutr Exerc Metab. 2015 Oct;25(5):463–470.
- Rothschild, JA, Kilding, AE, Plews, DJ. Prevalence and determinants of fasted training in endurance athletes: a survey analysis. Int J Sport Nutr Exerc Metab. 2020 Sep 1;30(5):345–356. doi: 10.1123/ijsnem.2020-0109
- Howarth, KR, Phillips, SM, MacDonald, MJ, et al. Effect of glycogen availability on human skeletal muscle protein turnover during exercise and recovery. J Appl Physiol ( 1985). 2010 Aug;109(2):431–438.
- Lemon, PW, Mullin, JP. Effect of initial muscle glycogen levels on protein catabolism during exercise. J Appl Physiol Respir Environ Exerc Physiol. 1980 Apr;48(4):624–629. doi: 10.1152/jappl.1980.48.4.624
- Biolo, G, Maggi, SP, Williams, BD, et al. Increased rates of muscle protein turnover and amino acid transport after resistance exercise in humans. Am J Physiol. 1995 Mar;268(3 Pt 1):E514–20.
- Churchward-Venne, TA, Pinckaers, PJM, Smeets, JSJ, et al. Dose-response effects of dietary protein on muscle protein synthesis during recovery from endurance exercise in young men: a double-blind randomized trial. Am J Clin Nutr. 2020 Aug 1;112(2):303–317. doi: 10.1093/ajcn/nqaa073
- Power, O, Hallihan, A, Jakeman, P. Human insulinotropic response to oral ingestion of native and hydrolysed whey protein. Amino Acids. 2009 Jul;37(2):333–339. doi: 10.1007/s00726-008-0156-0
- Impey, SG, Smith, D, Robinson, AL, et al. Leucine-enriched protein feeding does not impair exercise-induced free fatty acid availability and lipid oxidation: beneficial implications for training in carbohydrate-restricted states. Amino Acids. 2015 Feb;47(2):407–416. doi: 10.1007/s00726-014-1876-y
- Rothschild, JA, Kilding, AE, Broome, SC, et al. Pre-exercise carbohydrate or protein ingestion influences substrate oxidation but not performance or hunger compared with cycling in the fasted state. Nutrients. 2021 Apr 14; 13(4):1291. doi: 10.3390/nu13041291
- Gieske, BT, Stecker, RA, Smith, CR, et al. Metabolic impact of protein feeding prior to moderate-intensity treadmill exercise in a fasted state: a pilot study. J Int Soc Sports Nutr. 2018 Nov 29;15(1):56. doi: 10.1186/s12970-018-0263-6
- Taylor, C, Bartlett, JD, van de Graaf, CS, et al. Protein ingestion does not impair exercise-induced AMPK signalling when in a glycogen-depleted state: implications for train-low compete-high. Eur J Appl Physiol. 2013 Jun;113(6):1457–1468. doi: 10.1007/s00421-012-2574-7
- Frampton, J, Edinburgh, RM, Ogden, HB, et al. The acute effect of fasted exercise on energy intake, energy expenditure, subjective hunger and gastrointestinal hormone release compared to fed exercise in healthy individuals: a systematic review and network meta-analysis. Int J Obes (Lond). 2022 Feb;46(2):255–268. doi: 10.1038/s41366-021-00993-1
- Wingfield, HL, Smith-Ryan, AE, Melvin, MN, et al. The acute effect of exercise modality and nutrition manipulations on post-exercise resting energy expenditure and respiratory exchange ratio in women: a randomized trial. Sports Med Open. 2015 Jun 2; 1:11. doi:10.1186/s40798-015-0010-3
- Ratliff, KM, Kerksick, CM, Moon, JM, et al. Metabolic impact of feeding prior to a 60-min bout of moderate-intensity exercise in females in a fasted state. Front Sports Act Living. 2022;4:1070477. doi: 10.3389/fspor.2022.1070477
- American, College of Sports Medicine. ACSM’s guidelines for exercise testing and prescription. 10th. Philadelphia: Wolters Kluwer Philadelphia;2018. eng.
- Compher, C, Frankenfield, D, Keim, N, et al. Best practice methods to apply to measurement of resting metabolic rate in adults: a systematic review. J Am Diet Assoc. 2006 Jun;106(6):881–903. doi: 10.1016/j.jada.2006.02.009
- Fallaize, R, Zenun Franco, R, Pasang, J, et al. Popular Nutrition-related Mobile apps: an agreement assessment against a UK reference method. JMIR mHealth uHealth. 2019 Feb 20;7(2):e9838. doi: 10.2196/mhealth.9838
- Weir, JB. New methods for calculating metabolic rate with special reference to protein metabolism. J Physiol. 1949 Aug;109(1–2):1–9. doi: 10.1113/jphysiol.1949.sp004363
- Jeukendrup, AE, Wallis, GA. Measurement of substrate oxidation during exercise by means of gas exchange measurements. Int J Sports Med. 2005 Feb;26(Suppl 1):S28–37.
- Cohen, J. Statistical power analysis for the behavioral sciences. 2nd ed. New York: Academic press; 2013.
- Witard, OC, Jackman, SR, Breen, L, et al. Myofibrillar muscle protein synthesis rates subsequent to a meal in response to increasing doses of whey protein at rest and after resistance exercise. Am J Clin Nutr. 2014 Jan;99(1):86–95.
- Macnaughton, LS, Wardle, SL, Witard, OC, et al. The response of muscle protein synthesis following whole-body resistance exercise is greater following 40 g than 20 g of ingested whey protein. Physiol Rep. 2016 Aug;4(15). doi: 10.14814/phy2.12893
- Pennings, B, Boirie, Y, Senden, JM, et al. Whey protein stimulates postprandial muscle protein accretion more effectively than do casein and casein hydrolysate in older men. Am J Clin Nutr. 2011 May;93(5):997–1005. doi: 10.3945/ajcn.110.008102
- Koopman, R, Crombach, N, Gijsen, AP, et al. Ingestion of a protein hydrolysate is accompanied by an accelerated in vivo digestion and absorption rate when compared with its intact protein. Am J Clin Nutr. 2009 Jul;90(1):106–115. doi: 10.3945/ajcn.2009.27474
- Boirie, Y, Dangin, M, Gachon, P, et al. Slow and fast dietary proteins differently modulate postprandial protein accretion. Proc Natl Acad Sci, USA. 1997 Dec 23;94(26):14930–14935. doi: 10.1073/pnas.94.26.14930
- Hunter, GR, Moellering, DR, Carter, SJ, et al. Potential causes of elevated REE after high-intensity exercise. Med Sci Sports Exerc. 2017 Dec;49(12):2414–2421. doi: 10.1249/MSS.0000000000001386
- Pilegaard, H, Keller, C, Steensberg, A, et al. Influence of pre-exercise muscle glycogen content on exercise-induced transcriptional regulation of metabolic genes. J Physiol. 2002 May 15;541(Pt 1):261–271. doi: 10.1113/jphysiol.2002.016832
- Wojtaszewski, JF, MacDonald, C, Nielsen, JN, et al. Regulation of 5‘AMP-activated protein kinase activity and substrate utilization in exercising human skeletal muscle. Am J Physiol Endocrinol Metab. 2003 Apr;284(4):E813–22.
- Tipton, KD, Rasmussen, BB, Miller, SL, et al. Timing of amino acid-carbohydrate ingestion alters anabolic response of muscle to resistance exercise. Am J Physiol Endocrinol Metab. 2001 Aug;281(2):E197–206.
- Veasey, RC, Haskell-Ramsay, CF, Kennedy, DO, et al. The effect of breakfast prior to morning exercise on cognitive performance, mood and appetite later in the Day in habitually active women. Nutrients. 2015 Jul 14;7(7):5712–5732.
- Bachman, JL, Deitrick, RW, Hillman, AR. Exercising in the fasted state reduced 24-hour energy intake in active male adults. J Nutr Metab. 2016;2016:1984198. doi: 10.1155/2016/1984198
- Hill, RJ, Davies, PS. The validity of self-reported energy intake as determined using the doubly labelled water technique. Br J Nutr. 2001 Apr;85(4):415–430. doi: 10.1079/BJN2000281
- Aird, TP, Farquharson, AJ, Bermingham, KM, et al. Divergent serum metabolomic, skeletal muscle signaling, transcriptomic, and performance adaptations to fasted versus whey protein-fed sprint interval training. Am J Physiol Endocrinol Metab. 2021 Dec 1;321(6):E802–E820. doi: 10.1152/ajpendo.00265.2021