ABSTRACT
Macroautophagy/autophagy is a conserved degradation pathway in eukaryotes that is required for recycling unwanted intracellular components, maintaining homeostasis, and coping with biotic and abiotic stresses. Pathogens have evolved to subvert autophagic machinery by secreting host cell-entering effector proteins. Here, we provided evidence that an apple autophagy-related gene MdATG8i, activated autophagy and contributed to resistance against Valsa canker caused by Valsa Mali (Vm) when being overexpressed in apple. MdATG8i interacted with a plastid elongation factor Tu (MdEF-Tu) which became insoluble and aggregated during Vm infection and was degraded through the autophagy pathway. Intriguingly, we identified a highly-induced effector secreted from Vm, Vm1G-1794, which competitively interacted with MdATG8i, suppressed autophagy, and depleted MdEF-Tu out of MdATG8i complexes. The formation of stable MdEF-Tu aggregates caused by Vm1G-1794 promoted the susceptibility of apple to Vm. Overall, our study demonstrated that MdATG8i contributed to Vm resistance by targeting and degrading MdEF-Tu, and Vm1G-1794 competed with MdEF-Tu to target MdATG8i and prevent MdEF-Tu degradation, thus favoring infection.
Abbreviations: 35S: cauliflower mosaic virus 35S promoter; AIM: ATG8-interacting motif; ATG8–PE: ATG8 conjugated with phosphatidylethanolamine; BiFC: biomolecular fluorescence complementation; Con A: concanamycin A; Co-IP: co-immunoprecipitation; DEPs: differentially expressed proteins; DMSO: dimethyl sulfoxide; GFP: green fluorescent protein; hpt: hours post-treatment; LCI: luciferase complementation imaging; MdATG8i: autophagy-related protein 8i in Malus domestica; MDC: monodansylcadaverine; MdEF-Tu: elongation factor Tu in Malus domestica; MdNBR1: neighbor of BRCA1 in Malus domestica; N. benthamiana: Nicotiana benthamiana; OE: overexpression; PAMP: pathogen-associated molecular pattern; PTI: pattern-triggered immunity; qRT-PCR: quantitative reverse transcription PCR; RFP: red fluorescent protein; RNAi: RNA interference; ROS: reactive oxygen species; Ub: ubiquitin; V. Mali: Valsa Mali; WT: wild-type plant; YFP: yellow fluorescent protein
Introduction
Autophagy is a conserved process in eukaryotes that plays an important role in maintaining cellular homeostasis and the recycling of cytoplasmic components engulfed in double-membraned autophagosomes [Citation1–3]. Stimulated autophagy facilitates adaptation to diverse types of cellular stress, including starvation, aging and pathogen infection [Citation4–6]. The major steps in the process from autophagosome generation to maturation rely on a complex set of membrane trafficking factors or chaperone proteins [Citation7–9], and the autophagy machinery comprises several conserved autophagy-related (ATG) proteins [Citation10]. The ubiquitin-like conjugation complex ATG8/ATG8–PE (phosphatidylethanolamine) is a critical component in this process, as levels of its lipidated form contribute to the formation of autophagosomes [Citation11,Citation12]. ATG8–PE anchored into the phagophore membranes serves as a docking site for the selective recruitment of cargo through selective autophagy receptors, which can specifically target and degrade various cellular structures ranging from misfolded or ubiquitinated protein aggregates to damaged organelles and invading microbes [Citation13–15]. The discovery of a wide array of specialized autophagic receptor proteins, which function as sorting platforms that specifically interact with ATG8 proteins and recruit the selected cargo into the developing autophagosomes, such as NBR1/Joka2, has highlighted the selective nature of autophagy pathways in substrate degradation [Citation16–18].
In plants, several studies have shown that the role of autophagy in disease resistance depends on the lifestyle of the invading pathogens [Citation19,Citation20]. Autophagy positively regulates plant defense in response to necrotrophic pathogens [Citation21,Citation22]. Manipulation of autophagy by necrotrophic fungus benefits the uptake of nutrients from dead host cells, which is accompanied by bursts of reactive oxygen species (ROS), changes in endogenous hormone levels, endoplasmic reticulum (ER) stress, and the accumulation of ubiquitinated proteins [Citation23]. Autophagosome membrane expansion and vesicular trafficking are responsible for the delivery of immune-relevant compounds to cope with cellular stress and are regulated by secretory pathways [Citation24,Citation25]. Recent studies have shed light on the function of autophagy-related defense during compatible interactions of plants with oomycetes, fungi, and viruses, which involves the recruitment of specific substrates by receptor proteins, such as ATG8 and NBR1, that guide the cargos to phagophores [Citation26,Citation27]. Arabidopsis ORM1 and ORM2 negatively regulate FLS2-mediated plant immunity and serve as selective autophagy receptors to modulate the accumulation of FLS2 [Citation28]. NbP3IP may act as a selective cargo receptor that interacts with NbATG8 and degrades p3, an RNA silencing suppressor protein of RSV [Citation29].
To interrupt plant resistance and counteract pattern-triggered immunity (PTI), pathogens deliver virulence proteins termed effectors into host cells, which not only interfere with and suppress plant defense responses but also mediate the acquisition of nutrients [Citation30–33]. Adapted pathogens have evolved diverse strategies to suppress or stimulate the autophagic degradation of essential host components to support their pathogenicity [Citation34–37]. A conserved RxLR effector PexRD54 from Phytophthora infestans antagonizes Joka2-mediated autophagy by depleting Joka2 out of ATG8 complexes [Citation38]. Recent studies have systematically analyzed and validated the interaction of Arabidopsis ATG proteins with a subset of effectors from various pathogens [Citation39]. For instance, the Legionella effector RavZ targets Atg8-family proteins and cleaves the C-terminus of these proteins, thereby inhibiting phagophore expansion and suppressing autophagy [Citation40]. BSMV-γb inhibits the interaction between ATG7 and ATG8 to suppress autophagy and promote viral infection [Citation41]. TuMV VPg and 6K2 proteins inhibit NBR1-dependent autophagy by blocking the degradation of viral protein [Citation42]. However, the function and molecular mechanism in which the interaction between pathogen effectors and host ATG proteins modulate autophagy during pathogenesis remain unclear.
Plastid elongation factor EF-Tu has been documented to play an important role in protein synthesis by carrying aminoacyl-tRNAs to ribosomes in protein biosynthesis [Citation43]. In addition, EF-Tu acts as a protein chaperone independent of GTP or GDP that promotes the refolding of several denatured proteins [Citation44–46]. EF-Tu is induced by abiotic stress and plays an important role in heat tolerance in plants. The partial loss of chloroplast EF-Tu activities in Arabidopsis, maize, and wheat results in reduced heat tolerance [Citation47–49]. Arabidopsis heat-sensitive plastid EF-Tu was one of the most abundant protein aggregates derived from heat-denatured proteins [Citation50,Citation51]. Recently, plastid EF-Tu shown to rapidly form protein aggregates both in vitro and in vivo during heat stress [Citation49].
Apple Valsa canker caused by Valsa Mali fungus is a destructive disease that causes severe losses of apple in Eastern Asia [Citation52,Citation53]. Analysis of the V. Mali genome has identified 193 secreted proteins with no known annotation, and several candidate effectors might suppress BAX-induced cell death in Nicotiana benthamiana or contribute to the virulence and development of V. Mali [Citation54–57]. Here, we show that a small cysteine-rich secreted protein Vm1G-1794 from V. Mali, subverted autophagy-mediated defense responses by disrupting the interaction of MdEF-Tu and MdATG8i in a competitive manner, suppressing autophagic flux and attenuating the autophagy-mediated ubiquitination and degradation of MdEF-Tu aggregates. Overall, this study provided new insights into the substrates for autophagy upon V. Mali infection as well as the mechanism by which Vm1G-1794 exploits autophagy to undermine plant defense.
Results
MdATG8i positively regulated apple resistance against V. Mali by activating autophagy.
Previous studies have demonstrated that autophagy plays an important role in the resistance of plants to pathogens [Citation4]. Our previous work has revealed that MdATG8i, a key autophagy gene in apple, enhances resistance to abiotic stresses by activating the autophagy pathway when it is overexpressed [Citation58,Citation59]. Here, we found that the transcription of MdATG8i was induced significantly after 24 hpt (hours post-treatment) upon V. Mali infection compared with the control (). Next, two Malus germplasms with contrasting susceptibilities to V. Mali, ZD1 and ZH16 [Citation60], were selected for further analysis. RT-qPCR confirmed that the transcription of MdATG8i was induced rapidly after 24 hpt in ZH16 (resistant), and its transcription level was higher compared with that in ZD1 (susceptible) (Fig. S1A and S1B).
Figure 1. Overexpression of MdATG8i induced autophagy and autophagosome formation, which positively regulates resistance against V. Mali. (A) The relative transcript levels of MdATG8i in GL-3 at different stages during V. Mali infection were determined by RT-qPCR. MdEF1a was used for normalization. Control, sterile potato dextrose agar (PDA) medium; Treatment, V. Mali-infected leaves. (B and C) Representative disease symptoms of detached leaves and stems in GL-3 (WT) and transgenic lines overexpressing MdATG8i (OE#1 and OE#7) infected with V. Mali and photographed at 3 d and 7 d, respectively. (D and E) Statistical analysis of the relative fungal biomass of diseased leaves in (B) and the length of the necrotic regions of stems in (C) based on three independent biological replicates with at least 15 infected leaves or stems per replicate. (F) Representative transmission electron microscope (TEM) images of autophagic structures in leaves of WT and MdATG8i OE plants at 24 h post-treatment under control or treatment conditions. Arrowheads indicate the ultrastructure of autophagic bodies inside the vacuoles. Scale bar: 2 μm. (G) MDC-stained autophagosomes in leaves of WT and MdATG8i OE plants at 24 h post-treatment under control or treatment conditions visualized by fluorescence confocal microscopy. Scale bars: 20 μm. (H and I) Quantification analysis of the numbers of autophagic structure and punctate MDC-stained spots per section described in (F and G) with at least 10 individual images acquired from different leaf areas. Data represent means ± SD, n = 3. Different letters above each bar indicate significant differences at P < 0.05 based on one-way ANOVA followed by Tukey’s test. (J) Immunoblotting of the ATG8 protein level in total proteins extracted from transgenic MdATG8i OE#1, OE#7, and WT leaves infected by V. Mali. The non-lipidated and lipidated forms of ATG8 are indicated by ATG8 and ATG8–PE, respectively; hpt, hours post-treatment. Ponceau S staining of the membrane is shown as a loading control.
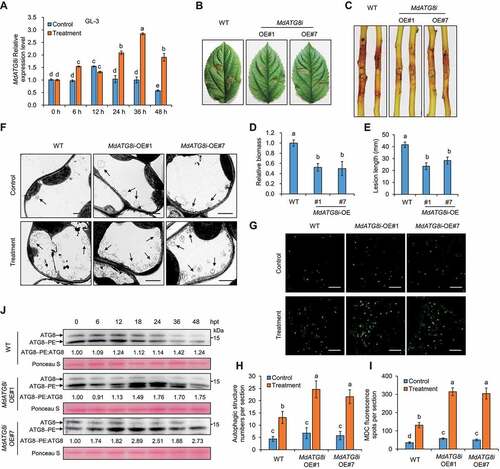
To investigate the function of MdATG8i in apple defense against V. Mali, we used two 35S::MdATG8i lines (OE#1 and OE#7) in a “GL-3” background, and “GL-3” was used as the wild-type (WT) (Fig. S1E and S1J). The specificity of the MdATG8i antibody was demonstrated by analysis with or without PLD (phospholipase D) (Fig. S2A and S2B). The 35S::MdATG8i lines showed higher V. Mali resistance than wild-type (WT) plants as indicated by the smaller necrotic regions on infected leaves and stems (). The autophagy induction by MdATG8i overexpression was monitored by transmission electron microscopy (TEM). Compared with WT plants, more autophagic structures were present in the vacuoles of 35S::MdATG8i lines after treatment (). MDC staining results revealed that V. Mali infection resulted in increased autophagosomes in 35S::MdATG8i lines compared with WT (). Furthermore, the conversion of ATG8 to ATG8–PE conjugates is considered an indicator of autophagy activation, which is consistent with the finding that the faster-migrating bands of ATG8–PE were more abundant in OE lines after 18 hpt than in WT ().
MdATG8i interacted with elongation factor MdEF-Tu and altered its subcellular localization
To identify potential MdATG8i-interacting proteins, we performed yeast two-hybrid (Y2H) screening with MdATG8i as bait (Table S1) and obtained MdEF-Tu, a relatively conserved translation elongation factor Tu in prokaryotes and eukaryotic plastids, as a target. The interaction between MdATG8i and MdEF-Tu was confirmed by Y2H (), in vitro GST pull-down, in vivo luciferase complementation imaging (LCI), and Co-IP analyses (). In addition, a web-based analysis (http://repeat.biol.ucy.ac.cy/iLIR) identified a potential ATG8-interaction motif (AIM) in MdEF-Tu, and the interaction between MdEF-Tu with MdATG8i depended on the AIM motif (Fig. S2C-S2E).
Figure 2. MdATG8i interacts with MdEF-Tu and altered the subcellular localization of MdEF-Tu. (A) Interaction between pGBKT7-MdATG8i and pGADT7-MdEF-Tu in the Y2H analysis. pGBKT7-53/pGADT7-RecT was used as the positive control. (B) Interaction between HA-MdATG8i and MdEF-Tu-GFP in the co-IP assay; empty vector was used as the negative control. Arrowheads indicate nonspecific bands; the protein loading control was stained with Ponceau S. (C) Interaction between cLUC-MdATG8i and MdEF-Tu-nLUC in the LCI assay. (D) Interaction between GST-MdATG8i and His-MdEF-Tu in the GST affinity-isolation assay; GST was used as a negative control. (E) Confocal microscopy images of single optical sections showing the localization of MdEF-Tu-GFP or MdEF-Tu-GFP with RFP-MdATG8i in N. benthamiana. Chloroplast autofluorescence was in blue. Scale bars: 20 μm. (F) MdEF-Tu-GFP and (G) MdEF-Tu-GFP co-expressed with RFP-MdATG8i were detected by immunoblotting in different cell fractionations extracted from N. benthamiana. Rbcl, UGPase, and H+-ATPase were detected as markers for the Rubisco large subunit, cytoplasm, and plasma membrane, respectively. T, total extract; Chl, chloroplasts; S, soluble fraction; M, membrane fraction. (H) Representative immunohistochemical staining of the protein localization of MdEF-Tu in apple leaves. Scale bars: 20 μm.
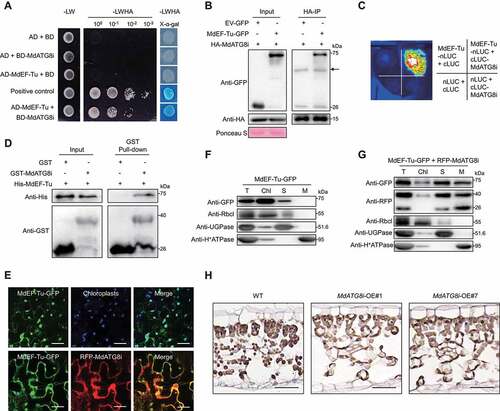
Previous studies have revealed that EF-Tu contains a chloroplast transport peptide in its N terminus and is located in the chloroplasts of wheat and Arabidopsis [Citation44,Citation48]. We hypothesized that interaction with MdATG8i might alter the localization of MdEF-Tu. To test this, we co-expressed MdEF-Tu-GFP and RFP-MdATG8i in N. benthamiana by agroinfiltration. When expressed alone, MdEF-Tu-GFP was mainly distributed in the chloroplasts (); however, when co-expressed with RFP-MdATG8i, a substantial amount of the GFP signal was detected in the cytoplasm and plasma membrane and colocalized with RFP-MdATG8i ( and Fig. S2F). Cell fractionation and immunoblotting analyses further confirmed that MdEF-Tu protein was mainly detected in the chloroplasts (). MdEF-Tu was less visible in the chloroplasts but accumulated in the soluble and membrane fractions when co-expressed with MdATG8i (). In addition, the effect of MdATG8i on the localization of MdEF-Tu was further verified by immunohistochemical staining in apple leaves (), which showed that the MdEF-Tu accumulated less in chloroplasts when MdATG8i was overexpressed.
MdEF-Tu negatively regulates apple resistance to V. Mali and was degraded during the infection process.
We examined the transcription of MdEF-Tu in “GL-3”; In comparison with the control, MdEF-Tu was down-regulated upon V. Mali infection, which was more evident between 12 to 36 hpt (). Similarly, the transcription of MdEF-Tu remained unchanged during infection but was induced under control conditions in both ZD1 and ZH16 (Fig. S1C and S1D). We generated 35S::MdEF-Tu transgenic apple plants (OE#1 and OE#3) (Fig. S1F and S1K). Leaves and stems from 35S::MdEF-Tu plants were more susceptible to V. Mali than WT (). By contrast, RNA interference (RNAi) transgenic calli of MdEF-Tu (RNAi#3 and RNAi#5) (Fig. S1G) showed reduced sensitivity to V. Mali than WT (). To elucidate how MdEF-Tu negatively regulates apple defense against V. Mali, total proteins were extracted from the infected leaves of WT or 35S::MdEF-Tu lines and purified by immunoprecipitation with anti-MdEF-Tu. The obtained proteins were trypsinized, separated and identified using LC-MS/MS (Table S2). In total, 43 differentially expressed proteins (DEPs) were identified, among which 38 were up-regulated and 5 were down-regulated (Fig. S3A and S3B). Based on Kyoto Encyclopedia of Genes and Genomes (KEGG) annotation, most of the up-regulated DEPs were linked with metabolism and concentrated in carbon metabolism or biosynthesis of amino acids (Fig. S3C). Clusters of Orthologous Groups (COG) annotation indicated that the up-regulated DEPs were mainly involved in carbohydrate transport and metabolism, translation, ribosomal structure and biogenesis, or posttranslational modification, protein turnover, chaperones (Fig. S3D). Enrichment analysis for Gene Ontology (GO) terms associated with ribosome, cytoplasm and integral component of membrane (Fig. S3E).
Figure 3. MdEF-Tu negatively regulates V. Mali resistance and is ubiquitinated and degradated upon infection. (A) The relative transcript levels of MdEF-Tu in GL-3 at different stages during V. Mali infection. (B and C) Representative disease symptoms of detached leaves and stems in GL-3 (WT) and transgenic lines overexpressing MdEF-Tu (OE#1 and OE#3) infected with V. Mali and photographed at 3 d and 7 d, respectively. (D and E) Statistical analysis of the relative fungal biomass of diseased leaves in (B) and the length of the necrotic regions of stems in (C). (F) Representative disease symptoms of WT and its transgenic calli with MdEF-Tu silenced (RNAi#3 and RNAi#5) under V. Mali infection; photographs were taken at 3 d. (G) Relative fungal biomass of V. Mali was determined by RT–qPCR in infected calli. Data represent means ± SD, n = 3. Different letters indicate significant differences at P < 0.05 based on one-way ANOVA followed by Tukey’s test. (H) MdEF-Tu overexpression suppressed chitin-induced ROS burst. Data represent means ± SD, n = 6. (I) MdEF-Tu overexpression suppressed chitin-induced MAPK activation. (J) Immunoblotting of MdEF-Tu stability at the indicated times under control or treatment conditions in MdEF-Tu overexpressing leaves. (K) Ubiquitination of MdEF-Tu in vivo upon V. Mali infection. The Ubiquitination of MdEF-Tu protein in both WT and MdEF-Tu OE#1 line during V. Mali infection was detected by immunoprecipitation analysis with anti-Ub and anti-MdEF-Tu antibodies. (L) Ubiquitination of MdEF-Tu in vitro. Purified recombinant protein His-MdEF-Tu was incubated with or without equal amounts of protein extracts from GL-3-infected leaves and was pulled down using a His-Select Nickel Affinity Gel.
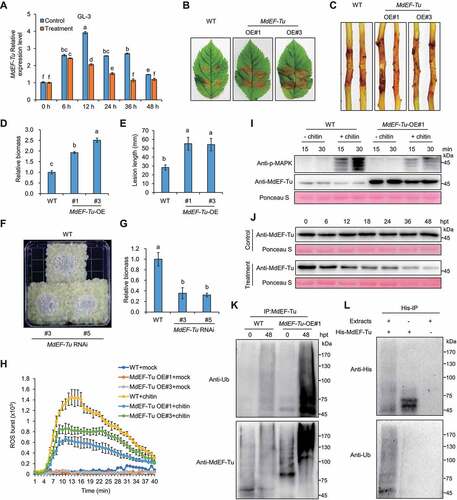
MdEF-Tu homologous protein in Arabidopsis thaliana, AtEF-Tu was prone to inactivation via heat-induced protein aggregation [Citation49]. In this study, total proteins were extracted from 35S::MdEF-Tu leaves infected with V. Mali at 24 hpt and separated into soluble and insoluble fractions, and MdEF-Tu aggregates were detected in the pellets (Fig. S4A). In addition, OE lines showed much higher levels of ubiquitinated proteins in the soluble and insoluble fraction of the total protein compared with WT during infection (Fig. S4B). Upon fungal cell-wall chitin oligomers elicitation, 35S::MdEF-Tu leaves demonstrated remarkably attenuated ROS burst and MAPK (mitogen-activated protein kinase) activation compared with WT (). Next, we found that MdEF-Tu was degraded gradually in V. Mali-infected leaves of 35S::MdEF-Tu lines; however, the degradation of MdEF-Tu was not observed under control conditions (). MdEF-Tu protein was more ubiquitinated in both WT and 35S::MdEF-Tu apple leaves infected by V. Mali at 48 hpt compared with 0 hpt, and the ubiquitination of MdEF-Tu was stronger in 35S::MdEF-Tu lines (). The interaction between MdEF-Tu and polyubiquitin protein (Ubi) was confirmed by the Co-IP assay (Fig. S4C), suggesting that MdEF-Tu was ubiquitinated and degraded during V. Mali infection as an immune-sensitive factor. Consistent with these findings, recombinant His-MdEF-Tu proteins purified from E. coli were co-incubated with or without total proteins extracted from leaves infected by V. Mali, and the ubiquitination of MdEF-Tu was also observed ( and Fig. S4D).
Ubiquitinated MdEF-Tu was degraded via autophagy
Immunoblots showed that the degradation of MdEF-Tu was accelerated in 35S::MdATG8i lines compared with WT upon infection (, lower panel), however, the slight accumulation of MdEF-Tu was observed in WT and 35S::MdATG8i lines under control conditions (, upper panel), which likely stemmed from the transcriptional upregulation of MdEF-Tu in the control compared with the treatment (, Fig. S1C and S1D). NEIGHBOR OF BRCA1 (NBR1) homolog in Arabidopsis can recruit ATG8-labeled autophagosomes as a cargo receptor, which mediates the selective autophagosomal degradation of ubiquitinated protein aggregates [Citation61]. NBR1 protein turnover has been used to indicate autophagic flux in plants because it is degraded in the vacuole in response to increases in autophagy activity. We found that the degradation of MdNBR1 was faster in 35S::MdATG8i lines than in WT upon V. Mali infection, which is similar to the pattern of MdEF-Tu degradation (). The ubiquitination of total protein from 35S::MdATG8i lines was similar to that of the WT during infection; however, more MdEF-Tu was ubiquitinated upon infection in 35S::MdATG8i lines compared with WT (). In addition, the degradation of MdEF-Tu and MdNBR1 extracted from bark was consistent with that from leaves (Fig. S4E). RT-qPCR revealed that the transcription profile of MdEF-Tu was similar in both WT and 35S::MdATG8i lines, suggesting that MdATG8i did not regulate MdEF-Tu at the transcriptional level during V. Mali infection (Fig. S4F). Furthermore, knocking down MdEF-Tu expression in “GL-3” leaves by agroinfiltration resulted in decreased sensitivity to V. Mali compared to the control (Fig. S4G and S4H).
Figure 4. Autophagy is required for the degradation of ubiquitinated MdEF-Tu. (A) Immunoblot analysis of endogenous MdEF-Tu and MdNBR1 in WT and MdATG8i OE#1 leaves upon infection. Sterile PDA medium was used as a control. (B) Ubiquitination of MdEF-Tu was detected by immunoprecipitation analysis. (C) Immunoblot analysis of endogenous MdEF-Tu and MdNBR1 in MdEF-Tu OE#1 leaves upon infection after inhibitor treatment. MG132, 26S proteasome inhibitor; Con A, 3-MA, and E64d, autophagy inhibitors; DMSO was used as a control. (D and E) Immunoblot analysis of endogenous MdEF-Tu and MdNBR1 in WT and MdATG5 RNAi#2 (D) or MdATG10 RNAi#1 (E) transgenic calli upon infection. Actin protein was used as the internal control. (F) Colocalization analysis of RFP-MdATG8i with MdEF-Tu-GFP in apple leaves upon infection with or without Con A treatment. Scale bars: 20 μm. (G) Co-immunoprecipitation analysis of interaction between MdATG8i and ubiquitinated MdEF-Tu in apple leaves upon infection with or without Con A treatment. Sterile PDA medium was used as a control. The asterisk indicates a nonspecific band that might represent an unidentified degradation product of MdNBR1. Ponceau S staining of the membrane is shown as a loading control.
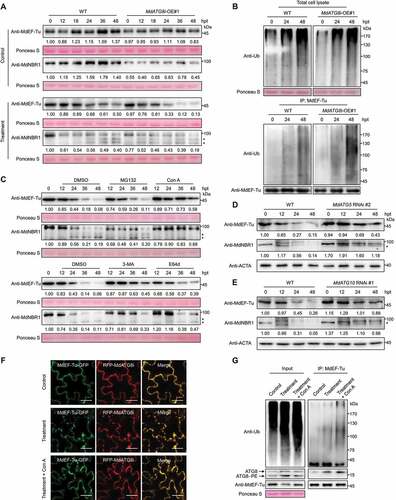
Autophagy and the ubiquitin-proteasome system are the two major protein degradation pathways involved in monitoring the steady-state level of ubiquitinated protein aggregates [Citation18]. We treated 35S::MdEF-Tu lines with MG132, concanamycin A (Con A), 3-methyladenine (3-MA), and cysteine protease inhibitor (E64d) and examined the levels of MdEF-Tu and MdNBR1 upon V. Mali infection. Immunoblots showed that MdEF-Tu and MdNBR1 were stabilized by autophagy inhibitors but not by MG132 (). Furthermore, the protein contents of MdEF-Tu and MdNBR1 were higher in MdATG5 and MdATG10 RNAi lines (Fig. S1H and S1I) compared with WT upon infection (). Consistent with the inhibition of MdEF-Tu degradation, the ubiquitination levels of both total proteins and co-immunoprecipitated MdEF-Tu were further enhanced by the application of autophagy inhibitors compared with DMSO or MG132 treatment after infection for 48 h (Fig. S4I). In addition, knockdown of MdATG5 and MdATG10 reduced the resistance of calli to V. Mali (Fig. S5A-S5D). Similarly, leaves with reduced MdATG5 and MdATG10 expression also had enhanced sensitivity to V. Mali compared to the control (Fig. S5E and S5F).
Confocal microscopy analysis indicated that RFP-MdATG8i and MdEF-Tu-GFP were colocalized throughout the cytoplasm and plasma membrane in apple leaves, however, more fluorescent puncta were observed upon V. Mali infection under treatment with or without the vacuolar-type ATPase inhibitor Con A (). Consistent with this, Co-IP assay showed that a stronger interaction between MdEF-Tu and MdATG8i, accompanied with more ubiquitination of MdEF-Tu in apple leaves, upon infection under treatment with or without Con A (). Furthermore, apple leaves with MdATG5 and MdEF-Tu simultaneously knocked down showed less sensitivity to V. Mali compared to knocking down MdATG5 alone (Fig. S5G and S5H), suggesting that MdEF-Tu aggregation is a key component of increased susceptibility to V. Mali.
V. Mali potential effectors directly targeted MdATG8i.
Considering that many pathogen effectors are known to overcome plant defenses by targeting certain immune components and MdATG8i improved apple V. Mali resistance via autophagy-mediated MdEF-Tu degradation, we asked whether there are V. Mali effectors that target MdATG8i and disturb its function. We previously documented that the AIM motif in MdEF-Tu was critical for MdEF-Tu and MdATG8i interaction (Fig. S2C-S2E). After analyzing a total of 193 candidate effector proteins from the V. Mali genome [Citation54], Vm1G-1794, Vm1G-8682, and Vm1G-11723 were found to contain potential AIM motifs and might target MdATG8i. The transcription levels of the three potential effectors were up-regulated upon V. Mali infection, which was highly induced at late stages of infection in ZD1 compared with “GL-3” (, Fig. S5I and S5J). The interaction between these effectors and MdATG8i was confirmed by Y2H and LCI analysis (). However, mutants without the AIM motif, Vm1G-1794ΔAIM and Vm1G-8682ΔAIM, did not interact with MdATG8i except for Vm1G-11723ΔAIM (Fig. S5K and S5L), suggesting that Vm1G-1794 and Vm1G-8682 interacted with MdATG8i depending on the AIM motif.
Figure 5. Identification and functional assay of the effectors in V. Mali that may target MdATG8i. (A) The relative transcript levels of Vm1G-1794 in GL-3 and ZD1 at different stages during V. Mali infection. VmG6PDH was used for normalization. ZD1, V. Mali-susceptible germplasm. Data represent means ± SD, n = 3. Different letters indicate significant differences at P < 0.05 based on one-way ANOVA followed by Tukey’s test. (B) Y2H analysis to detect the interaction of pGBKT7-MdATG8i with pGADT7-Vm1G-1794, pGADT7-Vm1G-8682, and pGADT7-Vm1G-11723. (C) LCI assays showed that cLUC-MdATG8i interacts with nLUC-tagged Vm1G-1794, Vm1G-8682, and Vm1G-11723 lacking its signal peptide. (D) The function of the signal peptides of Vm1G-1794 and Vm1G-8682 was identified using the yeast mutant YTK12. Avr1b was used as the positive control. Enzymatic activity of invertase was detected in TTC medium. (E) Immunoblot analysis of apoplastic fluid from N. benthamiana to validate the apoplastic location of full-length SP-Vm1G-1794. (F) ROS accumulation and callose deposition in N. benthamiana leaves were detected by DAB and aniline blue staining. Bars: 500 μm. (G) GST-MdATG8i interacts with MBP-Vm1G-1794 but not with MBP-Vm1G-1794ΔAIM in the GST affinity-isolation assay. GST was used as a negative control. (H) HA-MdATG8i interacted with Vm1G-1794-GFP but not with Vm1G-1794ΔAIM-GFP in the co-IP assay. GFP was used as the negative control. (I) Colocalization analysis of RFP-MdATG8i puncta with Vm1G-1794-GFP in N. benthamiana. The dotted square region focuses on RFP-MdATG8i-labeled puncta. Arrowheads point to punctate structures. Scale bars: 20 μm; scale bar in inset: 2 μm.
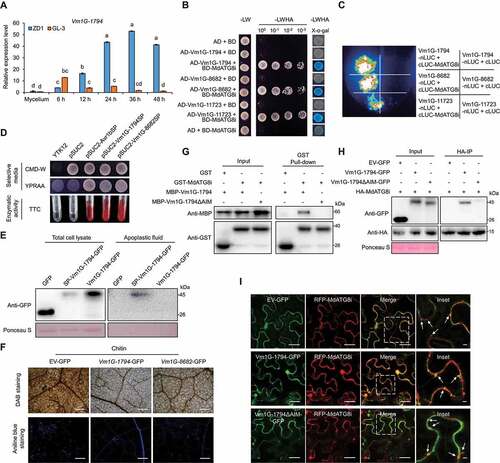
The signal peptides (SPs) of Vm1G-1794 and Vm1G-8682 were cloned into pSUC2 and transformed into YTK12, a yeast strain lacking a secreted invertase. The results illustrated that SP was sufficient for the secretion of invertase activity in yeast, and the enzyme activity was also detected by the TTC assay (). Vm1G-1794 and Vm1G-8682 suppressed chitin-induced ROS accumulation and callose deposition in N. benthamiana leaves ( and Fig. S5M). We selected Vm1G-1794, which had a higher transcription level during infection (), for further examination. The full-length SP-Vm1G-1794 was detected in apoplastic fluid of N. benthamiana leaves, but not Vm1G-1794 (). Moreover, confocal microscopy revealed that full-length SP-Vm1G-1794 was observed in the apoplast of N. benthamiana leaves after plasmolysis (Fig. S6A), further confirming the extracellular location of SP-Vm1G-1794.
Vm1G-1794 competitively bound with MdATG8i and interfered with the interaction between MdATG8i and MdEF-Tu
GST affinity-isolation and co-IP assays showed that MdATG8i directly interacted with Vm1G-1794 in vitro and in vivo but not with the AIM deletion mutant Vm1G-1794ΔAIM (). We found that Vm1G-1794 also interacted with five other ATG8 homologs in apple by Y2H, suggesting that the interaction was conserved (Fig. S6B). Confocal microscopy analysis indicated that RFP-MdATG8i and Vm1G-1794-GFP were colocalized in the cytoplasm and the punctate MdATG8i-labeled autophagosomes in N. benthamiana ().
Given that both MdEF-Tu and Vm1G-1794 interacted with MdATG8i via their AIM motifs, and Vm1G-1794 did not interact with MdEF-Tu (Fig. S6C and S6D), we hypothesized that the interaction with Vm1G-1794 would affect the MdEF-Tu-MdATG8i complex. A yeast three-hybrid assay demonstrated that the interaction of MdEF-Tu-MdATG8i was disrupted by Vm1G-1794 (). A BiFC assay revealed that the YFP signal was weaker when MdATG8i-nYFP and MdEF-Tu-cYFP were co-expressed with RFP-Vm1G-1794 compared with RFP-Vm1G-1794ΔAIM (). GST affinity-isolation and co-IP assays between MdEF-Tu and MdATG8i in the presence or absence of Vm1G-1794 confirmed that the enrichment of MdEF-Tu was notably decreased in MdATG8i immunoprecipitates with increased Vm1G-1794 relative to VM1G-1794ΔAIM (). LCI and co-IP assays indicated that not only Vm1G-1794, but also Vm1G-8682 decreased the association between MdEF-Tu and MdATG8i in a competitive manner (Fig. S6E and S6F).
Figure 6. Vm1G-1794 disrupts the MdATG8i-MdEF-Tu interaction as a competitive antagonist and suppressed MdATG8i-labeled autophagic flux. (A) Yeast three-hybrid analysis to detect the growth of yeast co-transformed with the MdATG8i-Vm1G-1794-MdEF-Tu complex. (B) Detection of YFP signals was interfered with by RFP-Vm1G-1794 in the BiFC colocalization assays. Scale bars: 20 μm. (C) Vm1G-1794 interferes with the association of MdEF-Tu with MdATG8i in vivo in the co-IP assay. (D) Vm1G-1794 competes with MdEF-Tu to bind to MdATG8i in vitro according to a competitive GST affinity-isolation assay. MBP was used as a negative control. The gradient indicates increasing concentrations of Vm1G-1794 or Vm1G-1794ΔAIM. (E) Co-expression of Vm1G-1794ΔAIM-GFP, but not Vm1G-1794-GFP, significantly increased the number of RFP-MdATG8i-labeled autophagosomes in N. benthamiana treated with Con A. Maximum projections of images represent independent Z-stacks with 30 individual images each. Scale bars: 50 μm. (F) Quantification analysis of the numbers of MdATG8i labeled autophagosomes per section described in (E) with at least 10 individual images acquired from different leaf areas. Data represent means ± SD, n = 3. Different letters indicate significant differences at P < 0.05 based on one-way ANOVA followed by Tukey’s test. (G) Immunoblot analysis of the processing of RFP-MdATG8i co-expressed with Vm1G-1794-GFP or Vm1G-1794ΔAIM-GFP in N. benthamiana leaves after E64d or Con A treatment. (H) Immunoblot analysis of the processing of GFP-MdATG8i co-expressed with RFP-Vm1G-1794 or RFP-Vm1G-1794ΔAIM in apple leaves upon infection with or without Con A treatment.
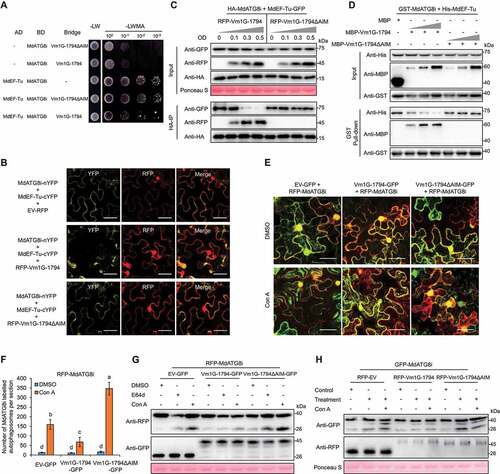
Vm1G-1794 inhibited autophagic flux and stabilized MdEF-Tu
An increase in ATG8-labeled puncta is widely used as a marker of autophagic activity. We examined the maximum projections of images consisting of Z-stacks with 30 independent images, in which RFP-MdATG8i was co-expressed with EV-GFP, Vm1G-1794-GFP, and Vm1G-1794ΔAIM-GFP in N. benthamiana leaves under treatment with the specific vacuolar ATPase inhibitor Con A. The puncta number of MdATG8i-labeled autophagosomes was decreased under expression with Vm1G-1794-GFP, but dramatically increased under expression with Vm1G-1794ΔAIM-GFP compared with EV-GFP ( and Fig. S7A). RFP-MdATG8i was degraded into free RFP, which reflects the induction level of autophagy. We further analyzed RFP-MdATG8i processing to confirm the inhibition of autophagic flux by Vm1G-1794. Immunoblot analysis showed that free RFP accumulated under the expression of Vm1G-1794ΔAIM but weakly in Vm1G-1794 after E64d or Con A treatment (). Furthermore, immunoblot analysis revealed higher autophagy activity after V. Mali infection under treatment with or without Con A in apple leaves expressing Vm1G-1794ΔAIM compared with Vm1G-1794 based on the level of released free GFP from GFP-MdATG8i cleavage ().
Pathogen-associated molecular patterns (PAMPs) recognized by pattern recognition receptors trigger PTI in host defense, and chitin, which activates PTI, is the best known PAMPs in oomycetes and fungi [Citation62]. Ubiquitination of MdEF-Tu was induced upon V. Mali infection. Previous data showed that the ubiquitinated MdEF-Tu was degraded via MdATG8i-mediated autophagy. We tested whether ubiquitination of MdEF-Tu also occurred in N. benthamiana leaves co-expressing HA-MdEF-Tu and RFP-MdATG8i treated with chitin. Immunoblot analysis revealed that autophagic flux was stimulated after chitin treatment based on the ratio of free RFP to RFP-MdATG8i, and co-precipitated MdEF-Tu was more ubiquitinated (Fig. S7G). To determine the involvement of Vm1G-1794 in MdATG8i-mediated MdEF-Tu degradation, we co-expressed Vm1G-1794 or Vm1G-1794ΔAIM with MdATG8i and MdEF-Tu and found that Vm1G-1794 reduced the ratio of free RFP to RFP-MdATG8i and stabilized MdEF-Tu after chitin treatment (Fig. S7H).
Vm1G-1794 contributed to V. Mali pathogenicity and suppressed the basal immune response.
To test the function of Vm1G-1794 during plant–pathogen interaction, we transiently expressed Vm1G-1794 in N. benthamiana and then infected leaves with P. capsici LT263. Larger water-soaked lesions were observed in leaves expressing Vm1G-1794 than in leaves expressing GFP, but no difference was observed between leaves expressing Vm1G-1794ΔAIM and GFP (Fig. S7B-S7D). We also transiently overexpressed Vm1G-1794 in apple leaves and observed increased sensitivity to V. Mali compared to the control (Fig. S7E and S7F). Consistent with these findings, transgenic apple “Orin” calli were generated for overexpressing Vm1G-1794 (OE#3 and OE#7) (Fig. S8A); larger disease lesions and greater biomass were observed in 35S::Vm1G-1794 transgenic calli after V. Mali infection than in GFP control calli (). Overexpression of Vm1G-1794 significantly suppressed the formation of autophagic structures in calli upon V. Mali infection compared with GFP control calli, as monitored by transmission electron microscopy (TEM) (). In addition, V. Mali infection resulted in decreased autophagosome numbers in 35S::Vm1G-1794 transgenic calli compared with the GFP control as evidenced by MDC staining (). Immunoblots revealed that the abundance of processing of ATG8 to ATG8–PE was lower in 35S::Vm1G-1794 calli and barely detected at 48 h after V. Mali infection compared with the GFP control; however, accumulation of MdEF-Tu or MdNBR1 was stable in transgenic calli (), which is consistent with findings in N. benthamiana (Fig. S7H). We found that levels of Vm1G-1794-GFP protein decreased considerably during infection compared with GFP protein (). RT-qPCR analyses indicated that the transcription of MdATG8i and MdEF-Tu was similar in both transgenic and control calli under infection (Fig. S8B and S8C), suggesting that Vm1G-1794 enhanced the susceptibility of apple calli to V. Mali but did not regulate MdATG8i and MdEF-Tu at the transcriptional level.
Figure 7. Vm1G-1794 is a virulence effector and counteracts the resistance against V. Mali conferred by MdATG8i-mediated autophagy. (A) Representative disease symptoms in transgenic calli overexpressing Vm1G-1794-GFP (OE#3 and OE#7) and infected with V. Mali; photographs were taken at 3 d. Overexpression of GFP empty vector was used as a control. (B) Relative fungal biomass of V. Mali in infected calli described in (A). (C) Representative transmission electron microscope (TEM) images of autophagic structures in transgenic calli of EV-GFP and Vm1G-1794-GFP OE#3 at 24 h post-treatment under control or treatment conditions. Arrowheads indicate the ultrastructure of autophagic bodies inside the vacuoles. Scale bar: 2 μm. (D) MDC-stained autophagosomes in the transgenic calli of EV-GFP and Vm1G-1794-GFP OE#3 at 24 h post-treatment under control or treatment conditions. Scale bars: 20 μm. (E and F) Quantification analysis of the numbers of autophagic structure and punctate MDC-stained spots per section described in (C and D) with at least 10 individual images acquired from different leaf areas. (G) ATG8 protein levels and stability of MdEF-Tu and MdNBR1 in calli overexpressing Vm1G-1794-GFP OE#3 or GFP empty vector upon V. Mali infection. Actin protein was used as the internal control. (H and I) Representative disease symptoms of detached stems and leaves in GL-3 infected with V. Mali WT strain 03–8 or Vm1G-1794 deletion mutant (#14 and #22) and photographed at 7 d and 3 d, respectively. (J and K) Statistical analysis of the length of the necrotic regions of stems in (H) and relative fungal biomass of diseased leaves in (I) based on three independent biological replicates with at least 15 infected leaves or stems per replicate. (L) ROS accumulation and callose deposition in GL-3 leaves infected with V. Mali WT strain 03–8 or Vm1G-1794 deletion mutant were detected by DAB and aniline blue staining. Bars: 500 μm. (M) Immunoblot analysis of the processing of GFP-MdATG8i in apple leaves infected with V. Mali WT strain 03–8 or Vm1G-1794 #14 deletion mutant with or without Con A treatment. Sterile PDA medium was used as a control. (N) Confocal microscopy analysis of the number of GFP-MdATG8i-labeled puncta in apple leaves infected with V. Mali WT strain 03–8 or Vm1G-1794 #14 deletion mutant with or without Con A treatment. Maximum projections of images represent independent Z-stacks with 30 individual images each. Scale bars: 50 μm. (O) Quantification analysis of the numbers of MdATG8i labeled puncta per section described in (N) with at least 10 individual images acquired from different leaf areas. (P) Stability of ATG8 and MdEF-Tu protein levels in GL-3 leaves infected with V. Mali WT strain 03–8 or Vm1G-1794 #14 deletion mutant. Ponceau S staining is shown as a loading control.
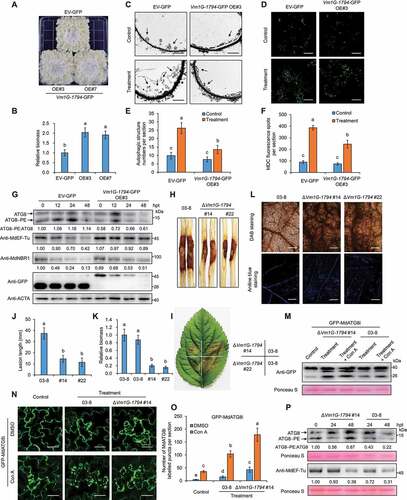
To evaluate the contribution of Vm1G-1794 to the virulence of V. Mali, we generated two Vm1G-1794 deletion mutants (#14 and #22) (Fig. S8D and S8E). Vm1G-1794 contributed to the virulence of V. Mali, as indicated by smaller necrotic regions on leaves and stems and lower biomass in tissues infected with Vm1G-1794 deletion mutants () which also had reduced pathogenicity to calli (Fig. S8G and S8H). However, obvious vegetative growth defects were observed during filamentous growth on PDA medium in Vm1G-1794 deletion mutants compared with WT strain 03–8 (Fig. S8F), suggesting that Vm1G-1794 is required not only for full virulence but also for the growth and development of V. Mali. Furthermore, ROS accumulation and extensive callose deposition were detected in apple leaves inoculated with Vm1G-1794 deletion mutants, indicating that the mutants failed to suppress PTI responses compared with WT strain 03–8 (). Immunoblot analysis revealed higher autophagy activity in apple leaves after Vm1G-1794 deletion mutant infection under treatment with or without Con A based on the cleavage of GFP-MdATG8i (). We examined the puncta of GFP-MdATG8i labeled autophagosomes in apple leaves by maximum projections of images consisting of Z-stacks, the puncta number was dramatically increased after Vm1G-1794 deletion mutant infection compared to WT strain 03–8 under treatment with Con A (). Furthermore, immunoblots revealed that the processing of ATG8 to ATG8–PE accumulated to a higher level in leaves infected with Vm1G-1794 deletion mutants than in leaves with 03–8, especially at 48 hpt; however, the accumulation of MdEF-Tu was similar in both infected leaves ().
Discussion
Ubiquitin modification is one of the main signals of protein degradation in biological cells. Ubiquitinated proteins are degraded by three biological pathways, 26S proteasome, autophagy, and vesicle transport, and selective autophagy is the classical process of autophagy-specific degradation of ubiquitinated proteins and protein aggregates for maintaining intracellular homeostasis. The elongation factor Tu (EF-Tu), an abundant protein in bacteria, was originally identified as a typical PAMP that triggers the PTI response in plants. Current studies of EF-Tu have been focused on examining the translation and synthesis of ribosomal proteins in eukaryotes. EF-Tu also acts as a molecular switch or protein chaperone to regulate many cellular processes [Citation44,Citation45,Citation47]. AtEF-Tu has been reported to play a role in plant heat tolerance, and it tends to form protein aggregates under heat stress [Citation49]. The CHIP-mediated 26S proteasome system and NBR1-mediated selective autophagy have been reported to degrade protein aggregates to alleviate heat stress [Citation50,Citation51]. Thus, EF-Tu aggregates might be targeted by certain protein degradation pathways under stressed conditions. As expected, we found that MdEF-Tu was induced by V. Mali to form protein aggregates (Fig. S4A), which was followed by the ubiquitination and degradation of MdEF-Tu (). In addition, more ubiquitinated proteins were accumulated in MdEF-Tu overexpressed lines upon infection (Fig. S4B). Previous studies have shown that the uncontrolled spread of cell death observed in autophagy-deficient mutants upon pathogen infection stems from the inappropriate accumulation of ubiquitinated protein aggregates and increased ER stress [Citation23]. Aggregated and deactivated proteins interact nonspecifically with other active proteins, promoting their misfolding and interfering with their functions [Citation49,Citation50], which makes host cells more susceptible to pathogens. Furthermore, a comparative proteomic analysis showed that carbon transport and metabolism, translation and protein turnover pathways were enriched in those up-regulated DEPs (Fig. S3). Previous studies reported that carbohydrate metabolism and proteolysis were utilized directly as an infection strategy by fungi in plant [Citation60,Citation63]. Therefore, overexpression of MdEF-Tu might affect resistance against V. Mali by promoting sugar derivatives and amino acid metabolism which will benefit V. Mali infection in apple.
Autophagic cargo receptor NBR1 is degraded along with polyubiquitinated cargos in the growing phagophore for selective clearance and thus acts as a selective autophagic substrate [Citation16]. We demonstrated that ubiquitin-modified MdEF-Tu aggregates were a substrate for autophagy-mediated degradation during infection (). We cannot rule out the possibility that MdEF-Tu is degraded directly via autophagy upon V. Mali infection given that it interacts with MdATG8i, similar to MdNBR1. In addition, the degradation dynamic of MdNBR1 was comparable to MdEF-Tu, suggesting that the autophagic degradation of MdEF-Tu and MdNBR1 might be relatively independent (). Furthermore, since MdEF-Tu did not interact with MdNBR1 (Fig. S6G and S6H), thus we hypothesized that MdNBR1 did not function as a cargo receptor in the degradation of ubiquitinated MdEF-Tu aggregates. However, the reason for elongation factor MdEF-Tu protein degradation via autophagy upon V. Mali infection remains unclear.
Like many other plant pathogenic fungi, V. Mali employs effectors to suppress plant defense responses [Citation52,Citation54]. Vm1G-1794 suppressed PTI responses, including chitin-induced ROS accumulation and callose deposition in N. benthamiana (), and blocked autophagic flux by interaction with MdATG8i via the AIM motif (), indicating that suppressing host autophagy benefits V. Mali. However, we have no evidence demonstrating whether Vm1G-1794-mediated autophagy inhibition is involved in cell death, and this often provides nutrients for necrotrophic fungi during the establishment of infection. Furthermore, we found that Vm1G-1794 interacted with at least five MdATG8 homologs (MdATG8a, MdATG8b, MdATG8d, MdATG8e, and MdATG8f) (Fig. S6B), suggesting that Vm1G-1794 might extensively suppress autophagy. In addition, overexpression of Vm1G-1794 reduced the resistance of apple calli to V. Mali () and N. benthamiana to P. capsici (Fig. S7B), suggesting that Vm1G-1794 possesses a conserved function in suppressing autophagy during plant defense. Pathogens have evolved various ways to hijack autophagy, which contributes to the accumulation of negative immune factors and/or substrates. Here, we described a similar mechanism during the apple-V. Mali interaction wherein the effector Vm1G-1794 competes with MdEF-Tu to bind MdATG8i (), which disrupts the degradation of MdEF-Tu aggregates in apple calli. As a consequence, the abundant MdEF-Tu aggregates result in increased susceptibility to V. Mali infection ().
Host autophagy can also target virulence effectors for degradation to strengthen defense. Tomato yellow leaf curl virus (TYLCV) induces protein aggregation in plants, and ATG8 co-exists in these aggregates to degrade viral proteins [Citation64]. NbP3IP-mediated defense against RSV is achieved by targeting RSV p3 for degradation via the autophagy pathway [Citation29]. CaMV P6 protein has been reported to protect viral capsid particle inclusions from autophagic degradation [Citation26]. In this study, we found that Vm1G-1794 was degraded gradually in the stable genetic transformation of apple calli upon infection (), and the degradation of Vm1G-1794ΔAIM also occurs under the transient transformation of N. benthamiana with or without chitin treatment (Fig. S6F and S7H). We speculated that Vm1G-1794 or Vm1G-1794ΔAIM might be degraded independently of autophagy given that Vm1G-1794ΔAIM did not interact with MdATG8i.
Vm1G-1794 deletion mutants had significantly decreased pathogenicity (), which part of that might stem from severe vegetative growth defects (Fig. S8F). Compared with WT strain 03–8, infection with Vm1G-1794 deletion mutants showed greater autophagy flux (), which was supposed to trigger the severe degradation of MdEF-Tu; however, we did not observe any difference in the abundance of MdEF-Tu when apple leaves were infected with Vm1G-1794 deletion mutants and the WT strain (). Thus, we cannot rule out the possibility that other effectors in V. Mali also interfere with the interaction between MdATG8i and MdEF-Tu, such as Vm1G-8682, which also interacted with MdATG8i in a manner similar to Vm1G-1794 (Fig. S6E and S6F).
In sum, V. Mali delivers Vm1G-1794 into the apple cells to suppress autophagy and promote pathogen virulence (). Identification of the core autophagy proteins could shed light on the specific roles of defense-related autophagy machinery in plant–pathogen interactions. Furthermore, the effector-directed strategy could be used to regulate the stability of host components and disturb complex cellular processes for the benefit of pathogens.
Figure 8. A working model of how Vm1G-1794 reduces autophagy-associated resistance by interfering with the interaction of MdATG8i-MdEF-Tu. Localization of MdEF-Tu is partially transferred out of chloroplast via interaction with MdATG8i under non-infection conditions. However, wheather the functional MdEF-Tu involved in autophagy is unknown. During V. Mali infection, autophagic degradation of ubiquitinated MdEF-Tu aggregates to improve resistance. However, V. Mali produces the cytoplasmic effector Vm1G-1794, which competitively combines with MdATG8i and blocks autophagic flux, resulting in the accumulation of MdEF-Tu aggregates and an increase in the susceptibility to V. Mali.
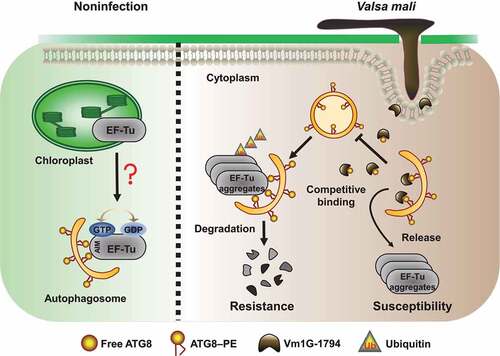
Materials and methods
Plant materials and growth conditions
All tissue culture plantlets of apple (Malus domestica) WT and transgenic lines used in this study were in a “Royal Gala” background, apple calli formation was induced from “Orin” cultivar (M. domestica Borkh), and Nicotiana benthamiana plants were used for transient gene expression. One-month-old seedlings and apple calli from transgenic and WT apple were harvested and mixed to eliminate genetic differences and used for RNA extraction and immunoblotting analysis. One-year-old leaves and stems of the same size from GL-3, transgenic plantlets, and two Malus germplasms, ZD1 and ZH16, were collected from the Horticultural Experimental Station of Northwest A&F University, Yangling (34°20 N, 108°24 E), China, and were used in the V. Mali resistance analysis and other assays.
The apple plantlets were incubated on Murashige and Skoog (MS) medium (Sigma-Aldrich, M5519) containing 0.5 mg L−1 indole-3-acetic acid (YEASEN, 41005ES25) and 1.5 mg L−1 6-benzylaminopurine (MedChemExpress, HY-B0941) with 2% sucrose (w:v; MedChemExpress, HY-B1779) and 0.8% agar (w:v; Sigma-Aldrich, A1296) at 22–25°C under 80% relative humidity and a 16-h light/8-h dark photoperiod with artificial fluorescent light for 30 days; the seedlings were then transferred to nutritive soil for further growth in an incubator. N. benthamiana plants were grown under the same conditions for transient expression assays, and apple calli were cultured on MS agar medium at room temperature under continuous darkness. The oomycete pathogen P. capsici strain LT263 used for inoculation in N. benthamiana was routinely cultured on 10% (v/v) V8 medium, and the V. Mali WT strain 03–8 and transformants were grown on PDA medium at 25°C in the dark.
Plasmid construction and plant transformation
Primer sequences with 20-bp extensions homologous to the corresponding plasmids used in this study are listed (Table S3). The full-length cDNA coding fragments of MdEF-Tu, MdNBR1, MdATG8i, and its homologous genes, or Vm1G-1794, Vm1G-8682, and Vm1G-11723, and its AIM deletion mutants lacking SPs were amplified from the genomic DNA of apple GL-3 or V. Mali strain 03–8, respectively. All ORFs were introduced into binary expression vectors under the control of the CaMV 35S promoter and the NOS terminator with or without protein tags. RNAi mediated gene silencing of MdEF-Tu, MdATG5 and MdATG10 were performed by infiltrating apple leaves using Agrobacterium strain EHA105 carrying pK7WIWG2D derived vectors, as described previously [Citation65].
Plasmids used for transient expression in N. benthamiana and apple leaves were constructed to generate GFP, RFP, HA, nLUC and cLUC, nYFP, and cYFP-tagged fusion vectors by Gateway BP/LR recombination reaction. The coding sequences used for stable genetic transformation in apple materials of MdEF-Tu, MdATG8i, or Vm1G-1794 were cloned into a modified pCambia2300 vector for overexpression or into the RNAi vector pK7GWIWG2 for gene-silencing. The recombinant vectors were transformed into Agrobacterium strain EHA105 and subsequently introduced into “Royal Gala” or “Orin” by Agrobacterium-medium transformation following a previously described protocol [Citation60]. The indicated genes were amplified and inserted into pGADT7 (Clontech, HG-VJC0483) and pGBKT7 (Clontech, HG-VJC0485) vectors, which were used to make the constructs for Y2H experiments. Genes for prokaryotic expression in vitro were individually cloned into the pGEX-4 T-1 (Sigma-Aldrich, GE28-9545-49), pMAL-c5x (New England Biolabs Inc, HG-VYN0870), or pET32a (Novagen, HG-VYN0176) vectors to express GST, MBP, or His-tagged fusion proteins in E. coli. All of these vectors were verified by sequencing.
V. Mali infection and generation of gene deletion mutants.
The WT and transgenic lines were infected using the stab inoculation and hole puncher wounding method as described previously [Citation60]. Briefly, cultures of the V. Mali strain 03–8 and deletion mutants were incubated at room temperature on potato dextrose agar medium for 3 d of darkness before use. Wounds were made using a sterile syringe in the fully expanded leaves from one-month-old culture seedlings or one-year-old plantlets and infected by mycelial discs using stab inoculation (leaves) and the hole puncher wounding method (stems) [Citation66]. Tissues were kept in a sealed container with high humidity for disease development; a sterile PDA disc was placed over the wound as a negative control. Lesion area phenotypes were evaluated and photographed at 3– 7 days after infection; relative fungal biomass was calculated using DNA-based RT-qPCR as described previously [Citation67].
Fragments of G418, a resistance gene used for screening, were fused with specific upstream and downstream sequences of the target genes by double-joint PCR to generate deletion cassettes as described previously [Citation56]. V. Mali transformation was performed to generate gene deletion mutants as described above, and amplified fragments were transformed into the protoplasts of strain 03–8 by a PEG (MedChemExpress, HY-Y0873A)-mediated approach as described previously [Citation68]. At least two independent mutants were generated, and four PCR primers were used to confirm that the target gene was indeed replaced.
Protein extraction and immunoblotting analysis
Total proteins were extracted from the leaves or stems and homogenized in ice-cold extraction buffer (50 mM Tris-HCl, pH 8.0, 200 mM NaCl, 1 mM EDTA, 1% Triton X-100 [MedChemExpress, HY-Y1883A], 5 mM DTT, 1 mM PMSF [MedChemExpress, HY-B0496], 10% glycerol) supplemented with a protease inhibitor cocktail tablet (MedChemExpress, HY-K0010). The samples were placed on ice for 30 min and centrifuged at 4°C at 12,000 × g for 10 min, and the protein concentrations were determined by a BCA protein assay kit (Sigma-Aldrich, FP0010). For immunoblot analysis, protein extracts were mixed with SDS loading buffer; boiled for 10 min, subjected to SDS-PAGE, and transferred to PVDF membrane; blocked with 5% skimmed milk in TBST buffer (MedChemExpress, HY-K1025) at room temperature; and incubated with specific anti-HA (Proteintech, 51,064-2-AP), anti-GFP (Proteintech, 66,002-1-lg), anti-RFP (Proteintech, 67,378-1-lg), anti-His (Proteintech, 25,940-1-AP), anti-GST (Proteintech, 66,001-2-lg), anti-MBP (Proteintech, 66,003-1-lg), anti-Ub (Proteintech, 10,201-2-AP), and anti-ACTA/actin (Proteintech, 23,660-1-AP) monoclonal antibodies. In addition, anti-MdEF-Tu, anti-ATG8, and anti-MdNBR1 polyclonal antibodies were produced in rabbit by Genscript, used in the immunoblotting analysis, and diluted in TBST buffer, followed by three washes. Membranes were incubated with appropriate secondary antibodies coupled with horseradish peroxidase (HRP) before the proteins were visualized using ECL kits (Abcam, ab133409).
Cell fractionation assays
The fresh leaves were frozen in liquid nitrogen and ground to a fine powder with a mortar and pestle; different cell fractions were then extracted using a plasma membrane extraction kit (Abcam, ab65400). Total proteins of MdEF-Tu-GFP and RFP-MdATG8i were separated into chloroplast protein, membrane protein, and soluble protein fractions, and anti-Rbcl (Solarbio Life Sciences, K900001P), anti-UGPase (Abcam, ab235828), and anti-H+-ATPase (Agrisera, AS07260) antibodies were used for immunoblot analysis.
Recombinant protein expression and purification
His, GST, and MBP tag-fused recombinant proteins were produced using E. coli strain BL21 (DE3) (Sangon Biotechnology, B528415-0010). Cells containing the prokaryotic expression constructs were incubated by adding 0.5 mM IPTG (Sangon Biotechnology, A600168-0025) to induce protein expression for 16 h at 20°C and suspended in protein extraction buffer supplemented with lysozyme and a cocktail tablet, followed by sonication and centrifugation at 10,000 × g for 10 min at 4°C. Crude proteins were extracted from cell lysate, and His fusion protein was purified using His-Select Nickel Affinity resin (QIAGEN, 30,210); GST and MBP fusion proteins were eluted with washing buffer (50 mM Tris-HCl at pH 8.0, 200 mM NaCl, 1 mM DTT, 1 mM PMSF) containing glutathione Sepharose (GenScript, L00206) or maltose resin (Abcam, ab270538) according to the manufacturer’s instructions. After purification, the purity of proteins was determined by SDS–PAGE and immunoblotting.
Y2H, LCI, and BiFC assays
The yeast strain AH109 was cultured at 30°C to an OD600 of 0.6. The cells were collected and mixed with the bait vector pGBKT7 and the prey vector pGADT7; these constructs were then transformed into yeast using the PEG lithium acetate method per the manufacturer’s protocol. The transformed cells were plated on synthetic defined (SD) medium (Coolaber, PM2040) without Leu and Trp and grown for 3 days at 30°C; they were then cultured on selection medium (SD-Leu-Trp-His-Ade) to detect the interaction.
The DNA fragments described above were fused to the N terminus of luciferase (nLUC) and the C terminus of luciferase (cLUC). The Agrobacterium strain EHA105 harboring the nLUC or cLUC fusion constructs was equally mixed and co-infiltrated into N. benthamiana leaves. Leaves were incubated with 100 mM d-luciferin potassium (MedChemExpress, HY-12591B) at 48 h after infiltration, and the luciferase luminescence was detected using a cooled CCD imaging system.
For the BiFC assay, the indicated plasmids were used to generate nYFP and cYFP fusion constructs that were transformed into Agrobacterium strain EHA105 and co-expressed in N. benthamiana leaves. For BiFC colocalization analysis, Agrobacterium carrying RFP-Vm1G-1794 construct was mixed with nYFP and cYFP combinations and infiltrated into N. benthamiana as described above. The YFP and RFP signals were detected by confocal laser scanning microscopy 48 h after infiltration.
GST affinity-isolation and co-IP assay
GST and GST-MdATG8i fusion protein were expressed in the BL21 strain and were immobilized with glutathione Sepharose at 4°C for 1 h, followed by three washes with PBS and mixing with E. coli crude extract containing the His-MdEF-Tu or MBP-Vm1G-1794 proteins at 4°C for 2 h with gentle shaking. The agarose was harvested and washed five times with cold washing buffer and then was boiled with SDS sample-loading buffer for 10 min for immunoblot analysis.
For the co-IP assay, MdEF-Tu-GFP and Vm1G-1794-GFP plasmids were co-expressed with HA-MdATG8i or GFP-MdATG8i into N. benthamiana and apple leaves. For agro-infiltration in apple leaves, 1-month-old tissue cultured apple plantlets were put in 10 mL syringe containing 2 mL agrobacteria suspension, and vacuum infiltrated for 5 min. The total proteins were extracted and lysed in IP buffer (100 mM Tris-HCl, pH 8.0, 150 mM NaCl, 1 mM EDTA, 0.5% Triton X-100, 1 mM DTT, 1 mM PMSF) at 48 h after infiltration and incubated with 25 µL of prewashed anti-HA or anti-MdEF-Tu affinity A/G PLUS-Agarose (Santa Cruz Biotechnology, sc-2003) at 4°C for 4 h with gentle shaking. The precipitated beads were then washed three times with cold washing buffer, followed by boiling with SDS sample-loading buffer for 10 min for immunoblot analysis.
Confocal laser scanning microscopy analyses
For colocalization experiments, the different GFP, YFP, and RFP fusion constructs driven by the CaMV 35S promoter were co-transformed into Agrobacterium strain EHA105, and then equal volumes of EHA105 cultures were infiltrated into N. benthamiana and apple leaves. The fluorescence signals were observed using sequential multi-channel scanning under a confocal laser-scanning microscope 48 h post-infiltration. The number of punctate structures in images obtained from independent Z-stacks consisting of 30 multi-layered images was determined using ImageJ.
To visualize the MDC-labeled autophagosomes, plant materials were immediately vacuum infiltrated with 100 μM MDC solution (Beyotime, C3018S) for 30 min in the dark until they were completely saturated and washed three times with PBS buffer. The fluorescent signals were monitored under a confocal laser scanning microscope excited by a wavelength of 405 nm and detected at 400 to 580 nm.
Identification of differential proteins by liquid chromatography/mass spectrometry
Denatured protein samples buffer (8 M urea [MedChemExpress, HY-Y0271], 100 mM TEAB [Sigma-Aldrich, 140,023], pH 8.5) were mixed with trypsin (MedChemExpress, HY-P71773) and CaCl2 solution overnight at 37°C for digestion as described previously [Citation63]. In brief, desalting protein was obtained and the supernatant was rinsed several times, then the filtrate was collected and lyophilized after dilution with 70% acetonitrile, then mixed with mobile phase solution (water containing 0.1% formic acid) to dissolve the lyophilized powder. Biomarker and BMKCloud was responsible for the LC/MS analysis and differential protein identification.
Interaction assays of two proteins binding to MdATG8i
For the yeast three-hybrid assay, MdATG8i was recombined into a pBridge vector fused with Vm1G-1794 or Vm1G-1794ΔAIM and co-transformed into yeast strain AH109 with pGADT7-MdEF-Tu as described above. Positive clones were grown on the synthetic defined (SD) medium without Leu and Trp for 3 days at 30°C and then cultured on selection medium (SD/-Leu-Trp-Met-Ade) to detect the Interaction.
For the competitive GST affinity-isolation assay, immobilized GST-MdATG8i was incubated with E. coli crude extract containing His-MdEF-Tu and subsequently mixed with increasing amounts of purified MBP-Vm1G-1794 or MBP-Vm1G-1794ΔAIM. The mixtures were then incubated at 4°C for 2 h with gentle shaking and washed five times as described above. The affinity-isolation components were boiled with SDS sample-loading buffer for 10 min for immunoblot analysis.
Protein ubiquitination and degradation assays
For the in vivo ubiquitination assay, MdATG8i or MdEF-Tu-overexpressing plants were used, and HA-MdEF-Tu was co-expressed with RFP-MdATG8i in N. benthamiana leaves; total proteins were extracted and lysed in native protein extraction buffer, and supernatants were incubated with anti-HA or anti-MdEF-Tu antibodies for 2 h at 4°C with gentle shaking. The reactions were then incubated with 30 µL of protein A/G PLUS-Agarose for 4 h at 4°C before immunoblot analysis. The ubiquitination patterns of MdEF-Tu in the immune complex were detected by anti-Ub antibody. The in vivo degradation assays were conducted as described above. Total proteins from WT or transgenic lines with or without inhibitor treatment were extracted at the indicated time points and then subjected to immunoblotting with the appropriate antibodies.
ROS burst and MAPK activation assays
Chitin-induced MAPK activation was visualized by immunoblotting using anti-p-MAPK/ERK (Proteintech, 11,257-1-AP) antibody. Chitin-induced oxidative burst was quantified as described previously [Citation62]. In brief, apple leaf disks were floated overnight on sterile water, added reaction buffer (0.2 mM regular luminol [YEASEN, 36207ES03], 10 µg/mL horseradish peroxidase [Santa Cruz Biotechnology, sc-391,122]) in a 96-well plate, and 50 mg/L chitin. Sterile water was used as mock. ROS burst in the form of chemiluminescence was immediately recorded in a time-course manner using a microplate reader.
RNA extraction and RT-qPCR analysis
Total RNA was extracted from various tissues using a Plant RNA Isolation Kit (Sangon Biotechnology, B518631-0050) per the manufacturer’s instructions. Approximately 2 µg of total RNA was used for cDNA synthesis with the RevertAid First Strand cDNA Synthesis Kit (Thermo Fisher Scientific, K1622). Using diluted cDNA as the template, RT-qPCR was performed in a 20-µL reaction volume with gene-specific primers (Table S2) using a LightCycler 96 real-time PCR machine and ChamQ SYBR qPCR Master Mix kit (Best Enzymes, EG20117 M) following the manufacturer’s protocol. Fold change was calculated by the comparative Ct method, and relative expression levels were calculated using the 2−ΔΔCt method based on three technical replications. The mRNA expression levels were normalized using the housekeeping genes MdEF1a and VmG6PDH as internal references.
Chemical inhibitors and chitin treatment
For inhibitor treatments, detached leaves of 30-day-old tissue culture plantlets grown on regular MS solid medium were transferred to half-strength MS liquid medium containing DMSO (MedChemExpress, HY-Y0320), 5 mM 3-MA (MedChemExpress, HY-19312), 50 µM E64d (MedChemExpress, HY-100229), 1 µM Con A (MedChemExpress, HY-N1724), or 50 µM MG132 (MedChemExpress, HY-13259), followed by soaking incubation in darkness at 25°C for 12 h. The detached leaves were examined for V. Mali infection after chemical inhibitor treatment. For N. benthamiana and apple, leaves transiently expressing constructs were treated with E64d or Con A in the dark at 48 hpi and collected for observation of autophagic activity. Total proteins were extracted, and MdEF-Tu and MdNBR1 abundance was determined by immunoblotting at the indicated time points.
For chitin treatments, N. benthamiana leaves transiently expressing indicated recombinant plasmids for 48 h were infiltrated with 100 µM chitin into leaves 12 h before leaves were harvested for immunoblotting and confocal microscopy analyses; sterile water was infiltrated as a control.
Trypan blue, DAB, and aniline blue staining
The three-month-old apple leaves were infected with V. Mali for 24 h. For trypan blue staining, infected leaves of N. benthamiana and apple were harvested and boiled in fresh trypan blue solution (YEASEN, 40207ES20) for 10 min and incubated for 24 h at room temperature. Leaves were destained in chloral hydrate and then submerged in 60% glycerol solution for microscopy. For DAB staining, infected leaves were submerged in DAB staining solution (YEASEN, 36201ES03) for 10 h in the dark, transferred to 90% ethanol at 65°C to remove chlorophyll until the leaves became transparent, and observed by bright-field microscopy.
For observation of callose deposits, infected leaves were decolorized in destaining solution (absolute ethyl alcohol:acetic acid, 1:1 v:v) and then immersed overnight in chloral hydrate to eliminate chlorophyll. The leaves were washed twice with 70% ethanol and distilled water, and the transparent leaves were stained with 0.5% aniline blue solution (Solarbio Life Sciences, A9540) overnight in the dark. Callose deposition was visualized by fluorescent microscopy using a DAPI filter.
Transmission electron microscopy and immunohistochemical staining
Apple leaves and calli were immediately cut into small pieces after V. Mali infection and then vacuum infiltrated with 2.5% glutaraldehyde solution for immobilization. Autophagic structures were observed by transmission electron microscopy as described previously [Citation58].
The pieces of immobilized leaf tissues were embedded in paraffin medium and prepared in semithin sections; these sections were then treated with anti-MdEF-Tu antibody. The specific procedures were based on those described in a previous study [Citation69].
SP function and apoplastic fluid extraction
The predicted SPs were introduced into the pSUC2 vector to generate SP-SUC2 fusion constructs and transformed into the invertase negative yeast strain YTK12. YTK12 and the transformants were assayed for growth on CMD-W plates (0.67% yeast nitrogen base without amino acids [Solarbio Life Sciences, Y8040], 0.075% tryptophan dropout supplement [Elife-Media, M394-01], 2% sucrose, 0.1% glucose [MedChemExpress, HY-B0389], and 2% agar) and YPRAA medium (1% yeast extract [Sigma-Aldrich, Y1625], 2% peptone [Millipore, P0521], 2% raffinose [MedChemExpress, HY-N7088], and 2 mg/ml antimycin A) to detect invertase secretion. Invertase enzymatic activity was detected by the reduction of 2,3,5-triphenyltetrazolium chloride (TTC) to insoluble red-colored 1,3,5-triphenylformazan (TPF) as described previously [Citation70]. The apoplastic fluid was extracted by the infiltration-centrifugation method in N. benthamiana leaves expressed with Vm1G-1794 and SP-Vm1G-1794 as described [Citation57].
Statistical analysis
Data reported in this study were usually calculated from three independent replicates unless otherwise indicated, and bars represent means ± SD. Statistical significance of the differences between groups was determined by one-way ANOVA followed by Tukey’s test. P-values < 0.05 were considered significant.
Accession numbers
Sequence data from this article can be found in the Malus domestica genome (GDDH13 v1.1) database, and GenBank database under the following accession numbers: MdEF-Tu (MD06 G1165400), MdATG5 (MD15 G1436000), MdATG10 (MD05 G1153700), MdATG8i (MD16 G1138400), MdATG8a (MD11 G1216100), MdATG8b (MD07 G1116100), MdATG8d (MD00G1114800), MdATG8e (MD09G1293100), MdATG8f (MD05 G1347700), MdNBR1 (MD14 G1171800), Vm1G-1794 (KUI65994.1), Vm1G-8682 (KUI73405.1), and Vm1G-11723 (KUI70449.1).
Data availability
Data supporting the major findings of this work are available within the manuscript and its Supplementary Information files. Any other supporting data is available from the corresponding authors upon request. Source data are provided with this paper.
Supplemental Material
Download MS Word (8.8 MB)Acknowledgments
We thank Dr. Chunlei Tang (Northwest A&F University) for providing pSUC2 vector, Prof. Yu Du (Northwest A&F University) for fruitful discussions. We are grateful to Dr. Yangyang Yuan and Minrong Luo (Horticulture Science Research Center, Northwest A&F University, Yangling, China) for providing professional technical assistance.
Disclosure statement
The authors declare that they have no competing interests.
Supplementary Material
Supplemental data for this article can be accessed online at https://doi.org/10.1080/15548627.2022.2153573
Additional information
Funding
References
- Boya P, Reggiori F, Codogno P. Emerging regulation and functions of autophagy. Nat Cell Bio. 2013;15(7):713–720.
- Galluzzi L, Pietrocola F, Levine B, et al. Metabolic control of autophagy. Cell. 2014;159(6):1263–1276.
- Yang X, Bassham DC. New insight into the mechanism and function of autophagy in plant cells. Int. Rev. Cell. Mol Biol. 2015;320:1–40.
- Hofius D, Li L, Hafrén A, et al. Autophagy as an emerging arena for plant-pathogen interactions. Curr Opin Plant Biol. 2017;38:117–123.
- Üstün S, Hafrén A, Hofius D. Autophagy as a mediator of life and death in plants. Curr Opin Plant Biol. 2017;40:122–130.
- Wang P, Mugume Y, Bassham DC. New advances in autophagy in plants: regulation, selectivity and function. Semin Cell Dev Biol. 2018;80:113–122.
- Reggiori F, Ungermann C. Autophagy pathway: cellular and molecular mechanisms. J Mol Biol. 2017;429(4):486–496.
- Yu L, Chen Y, Tooze SA. Autophagy pathway: cellular and molecular mechanisms. Autophagy. 2018;14(2):207–215.
- Yoshimoto K, Ohsumi Y. Unveiling the molecular mechanisms of plant autophagy-from autophagosomes to vacuoles in plants. Plant Cell Physiol. 2018;59(7):1337–1344.
- Mizushima N, Yoshimori T, Ohsumi Y. The role of Atg proteins in autophagosome formation. Ann Rev Cell Dev Biol. 2011;27(1):107–132.
- Noda NN, Ohsumi Y, Inagaki F. Atg8-family interacting motif crucial for selective autophagy. FEBS Lett. 2010;584(7):1379–1385.
- Slobodkin MR, Elazar Z, Lane JD. The Atg8 family: multifunctional ubiquitin-like key regulators of autophagy. Essays Biochem. 2013;55:51–64.
- Zaffagnini G, Martens S. Mechanisms of selective autophagy. J Mol Biol. 2016;428(9):1714–1724.
- Ryabovol VV, Minibayeva FV. Molecular mechanisms of autophagy in plants: role of ATG8 proteins in formation and functioning of autophagosomes. Biochemistry. 2016;81(4):348–363.
- Kellner R, De la Concepcion JC, Maqbool A, et al. ATG8 expansion: a driver of selective autophagy diversification? Trends Plant Sci. 2017;22(3):204–214.
- Svenning S, Lamark T, Krause K, et al. Plant NBR1 is a selective autophagy substrate and a functional hybrid of the mammalian autophagic adapters NBR1 and p62/SQSTM1. Autophagy. 2011;7(9):993–1010.
- Stolz A, Ernst A, Dikic I. Cargo recognition and trafficking in selective autophagy. Nat Cell Bio. 2014;16(6):495–501.
- Gatica D, Lahiri V, Klionsky DJ. Cargo recognition and degradation by selective autophagy. Nat Cell Bio. 2018;20(3):233–242.
- Lenz HD, Haller E, Melzer E, et al. Autophagy differentially controls plant basal immunity to biotrophic and necrotrophic pathogens. Plant J. 2011;66(5):818–830.
- Lenz HD, Haller E, Melzer E, et al. Autophagy controls plant basal immunity in a pathogenic lifestyle-dependent manner. Autophagy. 2011;7(7):773–774.
- Van Kan JA. Licensed to kill: the lifestyle of a necrotrophic plant pathogen. Trends Plant Sci. 2006;11(5):247–253.
- Lai Z, Wang F, Zheng Z, et al. A critical role of autophagy in plant resistance to necrotrophic fungal pathogens. Plant J. 2011;66(6):953–968.
- Munch D, Rodriguez E, Bressendorff S, et al. Autophagy deficiency leads to accumulation of ubiquitinated proteins, ER stress, and cell death in Arabidopsis. Autophagy. 2014;10(9):1579–1587.
- Ao X, Zou L, Wu Y. Regulation of autophagy by the Rab GTPase network. Cell Death Differ. 2014;21(3):348–358.
- Wang WM, Liu PQ, Xu YJ, et al. Protein trafficking during plant innate immunity. J Integr Plant Biol. 2016;58(4):284–298.
- Hafrén A, Macia JL, Love AJ, et al. Selective autophagy limits cauliflower mosaic virus infection by NBR1-mediated targeting of viral capsid protein and particles. Proc Natl Acad Sci USA. 2017;114(10):E2026–E2035.
- Jung H, Lee HN, Marshall RS, et al. Arabidopsis cargo receptor NBR1 mediates selective autophagy of defective proteins. J Exp Bot. 2020;71(1):73–89.
- Yang F, Kimberlin AN, Elowsky CG, et al. A plant immune receptor degraded by selective autophagy. Mol Plant. 2019;12(1):113–123.
- Jiang L, Lu Y, Zheng X, et al. The plant protein NbP3IP directs degradation of Rice stripe virus p3 silencing suppressor protein to limit virus infection through interaction with the autophagy-related protein NbATG8. New Phytol. 2021;229(2):1036–1051.
- Donofrio NM, Raman V. Roles and delivery mechanisms of fungal effectors during infection development: common threads and new directions. Curr Opin Microbiol. 2012;15(6):692–698.
- Giraldo MC, Valent B. Filamentous plant pathogen effectors in action. Nat Rev Microbiol. 2013;11(11):800–814.
- Wang X, Jiang N, Liu J, et al. The role of effectors and host immunity in plant-necrotrophic fungal interactions. Virulence. 2014;5(7):722–732.
- Fevre RL, Evangelisti E, Rey T, et al. Modulation of host cell biology by plant pathogenic microbes. Ann Rev Cell Dev Biol. 2015;31(1):201–229.
- Cheng X, Wang A. The potyvirus silencing suppressor protein VPg mediates degradation of SGS3 via ubiquitination and autophagy pathways. J Virol. 2016;91(1):e01478–e01416.
- Li F, Zhao N, Li Z, et al. A calmodulin-like protein suppresses RNA silencing and promotes geminivirus infection by degrading SGS3 via the autophagy pathway in Nicotiana benthamiana. PLoS Pathog. 2017;13(2):e1006213.
- Üstün S, Hafrén A, Liu Q, et al. Bacteria exploit autophagy for proteasome degradation and enhanced virulence in plants. Plant Cell. 2018;30:668–685.
- Ismayil A, Yang M, Haxim Y, et al. Cotton leaf curl Multan virus βC1 Protein Induces Autophagy by Disrupting the Interaction of Autophagy-Related Protein 3 with Glyceraldehyde-3-Phosphate Dehydrogenases[OPEN]. Plant Cell. 2020;32(4):1124–1135.
- Dagdas YF, Belhaj K, Maqbool A, et al. An effector of the Irish potato famine pathogen antagonizes a host autophagy cargo receptor. eLife. 2016;5:e10856.
- Lal NK, Thanasuwat B, Huang PJ, et al. Phytopathogen effectors use multiple mechanisms to manipulate plant autophagy. Cell Host Microbe. 2020;28(4):558–571.
- Choy A, Dancourt J, Mugo B, et al. The Legionella effector RavZ inhibits host autophagy through irreversible Atg8 deconjugation. Science. 2012;338(6110):1072–1076.
- Yang M, Zhang Y, Xie X, et al. Barley stripe mosaic virus γb protein subverts autophagy to promote viral infection by disrupting the ATG7-ATG8 Interaction. Plant Cell. 2018;30(7):1582–1595.
- Hafrén A, Üstün S, Hochmuth A, et al. Turnip mosaic virus counteracts selective autophagy of the viral silencing suppressor HCpro. Plant Physiol. 2018;176(1):649–662.
- Morse JC, Girodat D, Burnett BJ, et al. Elongation factor-Tu can repetitively engage aminoacyl-tRNA within the ribosome during the proofreading stage of tRNA selection. Proc Natl Acad Sci USA. 2020;117:3610–3620.
- Rao D, Momcilovic I, Kobayashi S, et al. Chaperone activity of recombinant maize chloroplast protein synthesis elongation factor, EF-Tu. Eur J Biochem. 2004;271(18):3684–3692.
- Ristic Z, Momcilović I, Fu J, et al. Chloroplast protein synthesis elongation factor, EF-Tu, reduces thermal aggregation of rubisco Activase. J Plant Physiol. 2007;164(12):1564–1571.
- Suzuki H, Ueda T, Taguchi H, et al. Chaperone properties of mammalian mitochondrial translation elongation factor Tu. J Biol Chem. 2007;282(6):4076–4084.
- Fu J, Momcilović I, Clemente TE, et al. Heterologous expression of a plastid EF-Tu reduces protein thermal aggregation and enhances CO2 fixation in wheat (Triticum aestivum) following heat stress. Plant Mol Biol. 2008;68(3):277–288.
- Ristic Z, Bukovnik U, Momcilović I, et al. Heat-induced accumulation of chloroplast protein synthesis elongation factor, EF-Tu, in winter wheat. J Plant Physiol. 2008;165(2):192–202.
- Li X, Cai C, Wang Z, et al. Plastid translation elongation factor Tu is prone to heat-induced aggregation despite its critical role in plant heat tolerance. Plant Physiol. 2018;176(4):3027–3045.
- Zhou J, Wang J, Cheng Y, et al. NBR1-mediated selective autophagy targets insoluble ubiquitinated protein aggregates in plant stress responses. PLoS Genet. 2013;9(1):e1003196.
- Zhou J, Zhang Y, Qi J, et al. E3 ubiquitin ligase CHIP and NBR1-mediated selective autophagy protect additively against proteotoxicity in plant stress responses. PLoS Genet. 2014;10(1):e1004116.
- Yin Z, Liu H, Li Z, et al. Genome sequence of Valsa canker pathogens uncovers a potential adaptation of colonization of woody bark. New Phytol. 2015;208(4):1202–1216.
- Yin Z, Zhu B, Feng H, et al. Horizontal gene transfer drives adaptive colonization of apple trees by the fungal pathogen Valsa Mali. Sci Rep. 2016b;6(1):33129.
- Li Z, Yin Z, Fan Y, et al. Candidate effector proteins of the necrotrophic apple canker pathogen Valsa Mali can suppress BAX-induced PCD. Frontiers in Plant Science. 2015;6:579.
- Zhang M, Feng H, Zhao Y, et al. Valsa Mali pathogenic effector VmPxE1 contributes to full virulence and interacts with the host peroxidase MdAPX1 as a potential target. Front Microbiol. 2018;9:821.
- Zhang M, Xie S, Zhao Y, et al. Hce2 domain-containing effectors contribute to the full virulence of Valsa Mali in a redundant manner. Mol Plant Pathol. 2019;20(6):843–856.
- Nie J, Yin Z, Li Z, et al. A small cysteine-rich protein from two kingdoms of microbes is recognized as a novel pathogen-associated molecular pattern. New Phytol. 2019;222(2):995–1011.
- Jia X, Mao K, Wang P, et al. Overexpression of MdATG8i improves water use efficiency in transgenic apple by modulating photosynthesis, osmotic balance, and autophagic activity under moderate water deficit. Hortic. Res. 2021;8(1):81.
- Huo L, Guo Z, Wang P, et al. MdHARBI1, a MdATG8i-interacting protein, plays a positive role in plant thermotolerance. Plant Sci. 2021;306:110850.
- Zhou K, Hu L, Li Y, et al. MdUGT88F1-Mediated Phloridzin Biosynthesis Regulates Apple Development and Valsa Canker Resistance. Plant Physiol. 2019;180(4):2290–2305.
- Zhang Y, Chen Z. Broad and complex roles of NBR1-mediated selective autophagy in plant stress responses. Cells. 2020;9(12):2562.
- Yang Q, Guo J, Zeng H, et al. The receptor-like cytoplasmic kinase CDG1 negatively regulates Arabidopsis pattern-triggered immunity and is involved in AvrRpm1-induced RIN4 phosphorylation. Plant Cell. 2021;33(4):1341–1360.
- Elagamey E, Abdellatef MAE, Arafat MY. Proteomic insights of chitosan mediated inhibition of Fusarium oxysporum f. sp. cucumerinum. J Proteomics. 2022;260:104560.
- Gorovits R, Fridman L, Kolot M, et al. Tomato yellow leaf curl virus confronts host degradation by sheltering in small/midsized protein aggregates. Virus Res. 2016;213:304–313.
- Zhang Q, Ma C, Zhang Y, et al. A single-nucleotide polymorphism in the promoter of a hairpin RNA contributes to alternaria alternata leaf spot resistance in apple (Malus × domestica). Plant Cell. 2018;30(8):1924–1942.
- Wei J, Huang L, Gao Z, et al. Laboratory evaluation methods of apple Valsa canker disease caused by Valsa ceratosperma sensu Kobayashi. Acta Phytopathologica Sinica. 2010;40:14–20.
- Yu X, Tang J, Wang Q, et al. The RxLR effector Avh241 from Phytophthora sojae requires plasma membrane localization to induce plant cell death. New Phytol. 2012;196(1):247–260.
- Gao J, Li Y, Ke X, et al. [Development of genetic transformation system of Valsa Mali of apple mediated by PEG]. Acta Microbiologica Sinica. 2011;51(9):1194–1199.
- Mochizuki T, Ohki ST. Detection of plant virus in meristem by immunohistochemistry and in situ hybridization. Methods Mol Biol. 2015;1236:275–287.
- Jiang C, Hei R, Yang Y, et al. An orphan protein of Fusarium graminearum modulates host immunity by mediating proteasomal degradation of TaSnRK1α. Nat Commun. 2019;11(1):4382.