ABSTRACT
This work uses elementary theoretical arguments to estimate whether softening of the surface could be used, along with surface texture and chemistry, to control superheat required for onset of nucleate boiling. For an ideal, smooth surface a mild decrease of the required superheat is predicted. In turn, an approximate closed-form model of vapor trapping and bubble seeding from soft surface with conical cavities shows linear dependence between the required superheat and the substrate’s shear modulus. Based on these results, considerations involved in implementing soft coatings for boiling applications and relevant outstanding fundamental questions are also briefly discussed.
Introduction
Surface engineering is often employed to manipulate interfacial thermofluidic phenomena to initiate boiling at temperatures closer to the saturation point. The difference between the surface temperature and the saturation point is referred to as the superheat required for the onset of nucleate boiling (ONB). Reduction of this difference significantly increases efficiency in many industrial applications such as power generation, thermal desalination, petroleum refining, and micro-electronic cooling [Citation1–Citation3]. Commercially available enhanced boiling surfaces that reduce the required superheat consist of either porous metal coatings or sub-millimeter metal fins with re-entrant geometries [Citation1]. In the last decade, the majority of the research in this area has focused on boiling enhancement using coatings with nano-to-microscale textures and/or chemical heterogeneities [Citation3–Citation6]. The goal of this work is to provide a first-order estimate of whether mechanical properties of the surface could provide a third design variable in addition to surface texture and chemistry for tuning superheat required for ONB. This goal is achieved by applying classical kinetic theory and by developing an approximate closed-form model to predict the superheat required for ONB on ideally smooth and textured soft surfaces, respectively.
While droplet wetting [Citation7–Citation15], condensation [Citation16–Citation19], freezing [Citation20], movement [Citation9–Citation12, Citation21–Citation28], and impact [Citation29–Citation36] on soft surfaces have been studied, vapor bubble interactions with soft materials have received little attention [Citation37]. Notably, Weijs et al. [Citation38] used molecular dynamics to quantify the deformation of a smooth, soft surface by a nanoscale vapor bubble. In current work, two bubble formation mechanisms are evaluated using elementary and approximate theoretical treatments to estimate the effect of surface softening on the superheat required for ONB. First, heterogeneous vapor bubble nucleation on smooth, soft surfaces is mapped in terms of the classical kinetic limit of the superheat required for vapor nucleation on liquid-solid and liquid-liquid interfaces. Second, augmentation of vapor trapping in surface cavities and superheat required to seed bubbles from the ensuing gas pockets induced by softening of the surface is evaluated using an approximate closed-form model. Finally, considerations involved in implementing soft coatings for boiling applications as well as relevant outstanding fundamental questions are briefly highlighted.
Results and discussion
From a kinetic point of view, sustained nucleate boiling occurs on an ideal smooth surface when the rate of formation of embryos with critical radius exceeds a threshold on the order of 1010 embryos per second per unit area [Citation2]. Given an expression for the nucleation rate, , one can estimate the limit of the superheat for sustained heterogeneous bubble nucleation. As recently emphasized in a review on elastocapillarity by Chen et al. [Citation37], currently there is no expression for the nucleation rate that accounts for mechanical properties of the substrate. Because of this limitation, the heterogeneous droplet nucleation on ideal smooth, soft solids has been assumed to be bound by the “hard” (i.e., liquid condensation on a hard solid surface) and “liquid” (i.e., liquid condensation on a liquid surface) limits [Citation16]. Here, this approach is adapted to estimate the effect of reducing the shear modulus of a smooth surface on the superheat required for sustained bubble nucleation. Specifically, the schematic in shows that, following the work on condensation by Eslami et al. [Citation16], bubble nucleation on soft solids can be charted in terms of limits imposed by boiling of liquid on hard solid surface and boiling of a liquid that is volatile in given conditions on surface of another liquid that is not volatile in given conditions.
Figure 1. Charting of the kinetic superheat limits versus spreading coefficient normalized by volatile liquid’s surface tension ( on soft substrates with wetting properties ranging from fully wetting to highly non-wetting in terms of the “hard solid” and “liquid” substrate limits. These limits are evaluated for (a) ethanol at 101 kPa with
of 351.5 K and in (b) water at 101 kPa and
of 373 K.
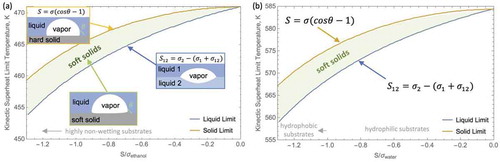
Experimentally validated expressions for exist for bubble nucleation from a volatile liquid on a hard solid surface [Citation2] as well as from a volatile liquid at the interface of two immiscible liquids [Citation39]. For the liquid on solid interface,
Where, the vapor embryo (subscript v) that is forming from the liquid (subscript l), with surface tension , has a contact angle of
when in contact with solid (subscript s).
and
are the temperature and pressure of the liquid respectively, while
and
are the saturation pressure and liquid specific volume at
. The number of liquid molecules immediately next to the surface,
is obtained from the liquid molecules density,
. Additionally,
is the mass of a molecule of the liquid,
is Boltzmann’s constant, and
is the gas constant. Lastly,
and
.
Correspondingly, the vapor embryo formation rate for interface of a volatile liquid (subscript 1) with liquid-vapor surface tension on a nonvolatile liquid (subscript 2) with liquid-vapor surface tension
is described by:
Jarvis et al. [Citation39] showed that bubble nucleation at the interface of the two liquids will only occur when the interfacial tension, , is greater than both
-
and
-
. If the first case is not satisfied, the volatile liquid spreads on the nonvolatile liquid and nucleation occurs entirely within the volatile liquid. On the other hand, if the second case is not satisfied, the nonvolatile liquid spreads on the volatile liquids, which leads to bubble formation within the nonvolatile liquid [Citation39].
One way to ensure that both of the conditions posed by Jarvis et al. [Citation39] are satisfied is to assume a hypothetical liquid substrate family with and varied
. On these liquids, nucleation will occur at the liquid-liquid interface (i.e., not entirely within either of the mediums [Citation39]) and will be mechanistically comparable to nucleation on liquid-solid interfaces. Furthermore, to make a direct comparison of the “hard” and “liquid” limits, the kinetic superheat limits are presented in terms of the spreading coefficient,
, non-dimensionalized by the surface tension of the volatile liquid (
). In the case of a liquid-solid interface,
. In turn,
for the case of the volatile liquid spreading on the nonvolatile liquid. A spreading coefficient approaching zero implies that the substrate is fully wetted by the volatile liquid. In turn, more negative values of the spreading coefficient imply more non-wetting behavior (e.g., for water surfaces with 0
-1 are hydrophilic and −1
are hydrophobic).
The plots in show that as the substrate softens from a “hard solid” to a “liquid” the superheat required for ONB of ethanol and water should decrease by, at most, 5 K and 8 K, respectively. Considering that the kinetic superheat limit is more than 100 K above the saturation point, a decrease of a few degrees is a minor improvement. It is imperative to note that kinetic superheat limits are only attained in highly controlled laboratory experiments [Citation39]. In more realistic conditions, the superheat required for ONB is significantly more moderate due to bubble seeding from vapor pockets trapped in surface cavities during the initial wetting of the substrate or later triple phase contact line (TPCL) movement [Citation2].
The volume of vapor trapped in surface cavities has been quantified using two approaches [Citation2, Citation40–Citation46]. The first approach, originally proposed by Bankoff [Citation43], is based on geometrical arguments. Specifically, as illustrated in ), it is thought that gas can be trapped when a liquid front with an advancing contact angle () greater than that of the groove or cone angle (2γ) passes over the cavity. Conversely, when 2γ>
the liquid floods the cavity, which significantly increases the superheat required to trigger nucleate boiling. The second approach, first studied by Johnson [Citation44] and significantly expanded by Wang [Citation47], is based on a thermodynamic criterion for the stability of the vapor pocket. Since the results of the second approach depend strongly on the interfacial areas within the cavities [Citation47], it is difficult to apply it to the cavities in soft materials, where the geometry will change with volume of the gas. Consequently, vapor trapping in soft material cavities is analyzed here using geometrical arguments. For further discussion on classical nucleation and boiling fundamentals, we refer the reader to Reference [Citation2].
Figure 2. Schematic of vapor trapping during liquid movement over a conical cavity in (a) a hard and (b) a soft material.
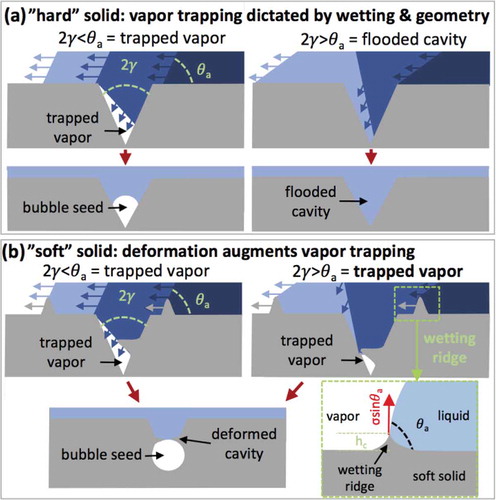
It is hypothesized here that softening of the substrate will augment ONB through a unique vapor trapping mechanism stemming from bridging of the cavities by microscopic deformation of the solid. This deformation, which is referred to here as the cavity bridge, is driven by the vertical component of the liquid’s surface tension acting on the substrate at the TPCL (see )). The height of the cavity bridge scales with the elastocapillary length (, where
is the substrate’s shear modulus [Citation10, Citation37]). For further discussion on elastocapillarity, we refer the reader to recent review by Chen et al. [Citation37]. In the case of growing water droplets, this length is on the order of 0.6 to 6 µm for soft, hydrophobic elastomers (
100 kPa to 10 kPa, respectively with
). Consequently, as illustrated in ), the cavity bridge is comparable to the size of the microscopic surface cavities; thus, it can trap the gas in the cavity. In the scenario where
<
, deformation of the cavity will have a minor effect on the volume of trapped gas. However, in the 2γ>
scenario, that is associated with cavity flooding on hard substrates (see right side of )), the bridging effect of soft substrates will continue to enable vapor trapping in the cavity (see right side of )).
As a first-order estimate, the bridging deformation can be assumed to be a plane that is locally normal to the surface of the cavity (i.e., the lateral extent of the deformation is assumed to have negligible thickness, see )). For simplicity, validity of this assumption for two-dimensional case, where the cavity represents a trough with a triangular cross-section, is discussed here. In two-dimensions, the assumed planar deformation reduces to a line that is perpendicular to the surface of the cavity and extends to the other side. The schematic in ) illustrates the difference between this assumption and cavity deformation simulated using finite element model (for details of the FEM simulation and benchmarking see Supporting Information). The plot in ) quantifies the volume occupied by trapped vapor obtained using the simulations and that predicted using the planar bridging deformation assumption. For the shear moduli of interest, where a sizeable quantity of gas is trapped, the difference between analytically predicted and simulated areas is below 25%. It is important to note that this error could be higher in the fully three-dimensional case of directional wetting of a conical cavity. This process is significantly more complex and could involve number of secondary cavity pinching modes. For the purposes of current work, however, these higher order deformations are neglected. In particular, the formulation of the problem is simplified here by treating the cavity bridge as a planar, tilted ellipse that crosses and caps the conical cavity.
Figure 3. (a) Schematic comparing the volume of gas trapped by the capillary deformation in a two-dimensional triangular trough obtained from finite element simulations (FEM) and that resulting from the planar bridging assumption and (b) corresponding plot quantifying fraction of the trapped cavity volume in this process obtained using simulations and that calculated using planar bridging assumption. Cavity with apex half angle of 40º and top opening width of 6 µm is considered here. Inset in (a) shows the FEM problem formulation.
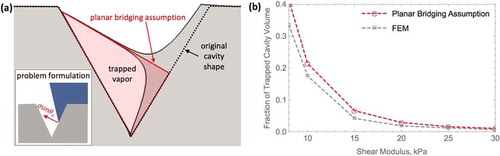
Using the planar bridging deformation assumption, the Lorenz solution [Citation46] can be adapted to approximate the volume of the trapped gas, (see Support Information for derivation details):
Since is below 0.7 for
of interest (see Support Information), the cavity bridge height plays a dominant role in determining the quantity of the entrapped gases. While
should not depend on velocity of the TPCL for an ideal elastic material, Karpitschka et al. [Citation26] recently showed that above a characteristic velocity,
, viscoelastic effects in realistic soft materials begin decreasing the
below the elastocapillary length on smooth, soft surfaces. For soft silicone gel,
is on the order of hundreds of micrometers per second [Citation26], which is slower than the TPCL velocity expected during surface flooding and boiling. However, this limitation may be mitigated by imperfections at the edge of the cavity pinning the TPCL, and thus allowing time for the wetting ridge to grow. While experimental results on the topic of TPCL movement across soft surfaces with cavities are scarce, recent work on wetting of superhydrophobic and omniphobic surfaces consisting of soft PDMS micro-pillars [Citation29, Citation48] implies that soft micro-textures do pin the TPCL. Additionally, these studies show that the advancing and receding contact angles are determined by the shape of the TPCL, which is distorted by pinning on the soft micro-pillars. Since droplets on the studied superhydrophobic surfaces sat on top of the PDMS pillars, the liquid temporarily pins at the edge of the micro-pillars as it steps from pillar to pillar. Because the edge of conical cavities provides a similar geometrical discontinuity (i.e., sharp step), it should also pin the TPCL. Consequently, for this analysis, the height of the cavity bridge is assumed to be independent of the TPCL velocity on the outside of the cavity and is equal to
.
Once trapped, vapor micro-pockets will deform the shape of the soft cavities due to the high surface tension forces at the TPCL and the Laplace pressure of the bubble. Since the latter depends on the radius of curvature of the liquid-gas interface, which is partially dictated by the solid geometry, determination of the vapor pocket shape is a complex thermo-mechanical problem. For the current work, this deformation is assumed to be approximately spherical. The error that this assumption introduces is estimated using FEM simulations and discussed in Support Information. Following Lorenz [Citation46], it is also assumed that volume change of the vapor during the trapping process is insignificant. Consequently, the radius of curvature of the spherical vapor pocket can be approximated as:
In boiling literature, it is assumed that the vapor filled cavity will actively seed bubbles when the cavity radius, is greater than the critical radius
[Citation2]:
Where ,
,
, and
, are respectively the saturation temperature at the given pressure, the latent heat of the fluid, the superheat, and the difference between vapor and liquid state specific volume of the fluid (the properties are calculated at the saturation temperature). By setting Eqs. 4 and 5 equal to each other and substituting the elastocapillary length for
, one obtains relationship between the superheat required to activate the cavity and the mechanical properties of the substrate:
Eq. 6 can be expressed as a product of terms consisting of the mechanical properties of the substrate, thermal properties of the fluid and operating conditions, and the geometry of the cavity. Most intriguingly, the superheat required for ONB on soft surfaces with conical cavities is linearly dependent on the shear modulus of the substrate. shows that low superheats (<5 K) can be achieved in the illustrative case of ethanol boiling on very soft silicone with a shear modulus less than 3 kPa. Since in this case, 2γ>
, “hard” surfaces with equivalent geometry would be flooded by ethanol and would require superheats approaching the high kinetic homogenous nucleation limit in order to induce vapor nucleation (see .
Figure 4. Plot of superheat required for onset of nucleate boiling of ethanol at 101 kPa on soft silicone surfaces (=30º [Citation58]) with conical cavities and varied mechanical properties.
![Figure 4. Plot of superheat required for onset of nucleate boiling of ethanol at 101 kPa on soft silicone surfaces (=30º [Citation58]) with conical cavities and varied mechanical properties.](/cms/asset/71d30dfe-d5fe-4693-b740-ca90a815733f/umte_a_1476634_f0004_oc.jpg)
Conclusions
The simplified theoretical arguments presented in this work show that while softening of a smooth surface would provide very mild benefits, the superheat required for the onset of nucleate boiling of liquids with 2γ> can be strongly influenced using soft substrates with vapor trapping cavities. This scenario, which is traditionally associated with flooding of cavities in hard substrates, occurs most frequently with low surface tension liquids. Thus, using soft textured coatings could potentially have significant impact in a variety of industrial applications where low surface tension liquids are utilized (e.g., refrigeration, petroleum refining, and microelectronics cooling). This reduction in the superheat stems from the substrate deformation, induced by surface tension forces at the moving TPCL, temporarily bridging the cavity and trapping vapor. The derived, simplified analytical expression predicts that, for the representative case of ethanol, nucleate boiling could become active at a superheat lower than 5 K on soft silicones with shear moduli lower than 3 kPa. It is important to point out that these soft properties are required at elevated boiling temperatures. Since silicones soften significantly with temperature [Citation49], the required coatings can be stiffer at room temperature than values predicted in . It is also important to note that even more impressive superheat reduction might be achieved with use of more complex cavity geometries than the simple conical geometry described here.
From a practical perspective, there are no evident obstacles to use of soft silicones coatings for enhanced boiling. In fact, their application might have several advantages over metallic surfaces. In particular, soft silicones are readily available, can withstand temperatures over 500 K [Citation49], and can be textured using variety of low cost microfabrication techniques [Citation50]. In addition, recent work has shown that silicones can be made as thermally conductive as 10 W/mK without significant change of mechanical properties of the polymer through addition of liquid metal nano/microscale inclusions [Citation51–Citation53]. In our recent work we showed that with addition of hybrid liquid-solid metal fillers we can achieve silicone composites with even higher thermal conductivity of up to 17 Wm−1K−1 [Citation54], and could adjust the liquid metal part of the particles to control electrical properties of such materials [Citation55]. Consequently, with heat fluxes of 103 to 104 Wm−2 typically observed for ONB [Citation2], filled silicone coatings with thickness of 10 to 100 µm and thermal conductivity on the order of 10 Wm−1K−1 would introduce negligible temperature drops of only a fraction of a degree Kelvin. Furthermore, silicones are already used in boilers for corrosion protection [Citation56] and, as in case of biofouling [Citation57], they could reduce fouling. Consequently, soft textured coatings could provide an attractive alternative to the current enhanced boiling surfaces.
Owing to absence of literature on this topic, the present work used a number of simplifying assumptions in order to provide an estimate of the effect of shear modulus on superheat required for ONB. Since the results indicate a favorable relationship between these two characteristics, there are several relevant fundamental questions that warrant further investigation. First, the microscale dynamics of solid deformation during movement and pinning of the TPCL across textured soft substrates needs to be further characterized. Second, the coupling between solid and bubble dynamics in soft cavities also needs to be studied. While not addressed in this work, bubble growth and detachment dynamics strongly impact nucleate boiling and their alteration through surface softening should also be investigated. Soft textured surfaces dynamic deformation could also disrupt other aspects of boiling, such as vapor film formation near critical heat flux. Thus, in summary, the current work provides motivation for further applied and fundamental investigation on the control of boiling through mechanical properties of the substrate.
Acknowledgments
KR wishes to sincerely thank Ms. Agata Renata Rykaczewski for thought provoking discussions that stimulated the principle idea behind this letter as well as Mr. Kenneth C. Manning and Prof. Jay Oswald from ASU for helpful discussions.
References
- R. L. Webb and N.-H. Kim, Principles of Enhanced Heat Transfer, New York, NY: Taylor Francis, 2007.
- V. P. Carey, Liquid–Vapor Phase-Change Phenomena: An Introduction to the Thermophysics of Vaporization and Condensation Process in Heat Transfer Equipment, 2nd ed. Bristol: Taylor and Francis, 2007.
- H. J. Cho, D. J. Preston, Y. Zhu, and E. N. Wang, “Nanoengineered materials for liquid–vapour phase-change heat transfer,” Nat. Rev. Mater., vol. 2, pp. 16092, 2016. DOI: 10.1038/natrevmats.2016.92.
- S. Bhavnani et al., “Boiling augmentation with micro/nanostructured surfaces: current status and research outlook,” Nanoscale Microscale Thermophys. Eng., vol. 18, no. 3, pp. 197–222, 2014.
- D. Attinger et al., “Surface engineering for phase change heat transfer: A review,” MRS Energy Sustain, vol. 1, pp. E4, 2014. DOI: 10.1557/mre.2014.9.
- N. A. Patankar, “Supernucleating surfaces for nucleate boiling and dropwise condensation heat transfer,” Soft Matter, vol. 6, no. 8, pp.1613, 2010. DOI: 10.1039/b923967g.
- R. Pericet-Cámara, A. Best, H. J. Butt, and E. Bonaccurso, “Effect of capillary pressure and surface tension on the deformation of elastic surfaces by sessile liquid microdrops: an experimental investigation,” Langmuir, vol. 24, no. 19, pp.10565–10568, 2008. DOI: 10.1021/la801862m.
- R. Pericet-Camara, et al., “Solid-supported thin elastomer films deformed by microdrops,” Soft Matter, vol. 5, no. 19, pp.3611, 2009. DOI: 10.1039/b907212h.
- R. W. Style and E. R. Dufresne, “Static wetting on deformable substrates, from liquids to soft solids,” Soft Matter, vol. 8, pp. 7177, 2012. DOI: 10.1039/c2sm25540e.
- R. W. Style, A. Jagota, C.-Y. Hui, and E. R. Dufresne, “Elastocapillarity: surface tension and the mechanics of soft solids,” Annu. Rev. Condens. Matter Phys., vol. 8, pp. 99–118, 2016. DOI: 10.1146/annurev-conmatphys-031016-025326.
- S. J. Park, et al., “Visualization of asymmetric wetting ridges on soft solids with X-ray microscopy,” Nat. Commun., vol. 5, pp. 4369, 2014. DOI: 10.1038/ncomms5369.
- L. Chen, G. K. Auernhammer, and E. Bonaccurso, “Short time wetting dynamics on soft surfaces,” Soft Matter, vol. 7, no. 19, pp.9084, 2011. DOI: 10.1039/c1sm05967j.
- A. Carlson, P. Kim, G. Amberg, and H. A. Stone, “Short and long time drop dynamics on lubricated substrates,” Europhys. Lett., vol. 104, no. 3, pp.34008, 2013. DOI: 10.1209/0295-5075/104/34008.
- Y.-S. Yu and Y.-P. Zhao, “Elastic deformation of soft membrane with finite thickness induced by a sessile liquid droplet,” J. Colloid Interface Sci., vol. 339, no. 2, pp.489–494, 2009. DOI: 10.1016/j.jcis.2009.08.001.
- D. Long, A. Ajdari, and L. Leibler, “Static and dynamic wetting properties of thin rubber films,” Langmuir, vol. 12, no. 21, pp.5221–5230, 1996. DOI: 10.1021/la9604700.
- F. Eslami and J. A. W. Elliott, “Thermodynamic investigation of the barrier for heterogeneous nucleation on a fluid surface in comparison with a rigid surface,” J. Phys. Chem. B, vol. 115, no. 36, pp.10646–10653, 2011. DOI: 10.1021/jp202018e.
- M. Sokuler, et al., “The softer the better: fast condensation on soft surfaces,” Langmuir, vol. 26, no. 3, pp.1544–1547, 2010. DOI: 10.1021/la903996j.
- A. Phadnis and K. Rykaczewski, “Dropwise condensation on soft hydrophobic coatings,” Langmuir, vol. 33, no. 43, pp.12095–12101, 2017. DOI: 10.1021/acs.langmuir.7b03141.
- R. Narhe et al., “Inverted Leidenfrost-like effect during condensation,” Langmuir, vol. 31, no. 19, pp. 5353–5363, 2015. DOI: 10.1021/la504850x.
- J. Petit and E. Bonaccurso, “General frost growth mechanism on solid substrates with different stiffness,” Langmuir, vol. 30, no. 4, pp.1160–1168, 2014. DOI: 10.1021/la404084m.
- R. W. Style, C. Hyland, R. Boltyanskiy, J. S. Wettlaufer, and E. R. Dufresne, “Surface tension and contact with soft elastic solids,” Nat. Com, vol. 4, pp. 2728, 2013.
- B. Andreotti et al., “Solid capillarity: when and how does surface tension deform soft solids?” Soft Matter, vol. 12, no. 12, pp. 2993–2996, 2016. DOI: 10.1039/C5SM03140K.
- A. Carré and M. E. R. Shanahan, “Viscoelastic braking of a running drop,” Langmuir, vol. 17, no. 10, pp.2982–2985, 2001. DOI: 10.1021/la001600e.
- A. Carré and M. E. R. Shanahan, “Viscoelastic dissipation in wetting, dewetting and adhesion phenomena,” Am. Chem. Soc. Polym. Prepr. Div. Polym. Chem., vol. 37, pp. 72–73, 1996.
- Y. Wang et al., “Flexible slippery surface to manipulate droplet coalescence and sliding, and its practicability in wind-resistant water collection,” ACS Appl. Mater. Interfaces, vol. 9, no. 29, pp. 24428–24432, 2017. DOI: 10.1021/acsami.7b06775.
- S. Karpitschka, et al., “Droplets move over viscoelastic substrates by surfing a ridge,” Nat. Commun., vol. 6, pp. 7891, 2015. DOI: 10.1038/ncomms8891.
- X. Yao, et al., “Adaptive fluid-infused porous films with tunable transparency and wettability,” Nat. Mater., vol. 12, no. 6, pp.529–534, 2013. DOI: 10.1038/nmat3598.
- W.-K. Lee, W.-B. Jung, S. R. Nagel, and T. W. Odom, “Stretchable superhydrophobicity from monolithic, three-dimensional hierarchical wrinkles,” Nano Lett., vol. 16, no. 6, pp.3774–3779, 2016. DOI: 10.1021/acs.nanolett.6b01169.
- M. Coux, C. Clanet, and D. Quéré, “Soft, elastic, water-repellent materials,” Appl. Phys. Lett., vol. 110, no. 25, pp.251605, 2017. DOI: 10.1063/1.4985011.
- T. Vasileiou, T. M. Schutzius, and D. Poulikakos, “Imparting icephobicity with substrate flexibility,” Langmuir, vol. 33, no. 27, pp.6708–6718, 2017. DOI: 10.1021/acs.langmuir.7b01412.
- P. B. Weisensee, J. Tian, N. Miljkovic, and W. P. King, “Springboard droplet bouncing on flexible superhydrophobic substrates,” J. Heat Transfer, vol. 139, no. 2, pp.20902, 2017. DOI: 10.1115/1.4035572.
- S. Gart, J. E. Mates, C. M. Megaridis, and S. Jung, “Droplet impacting a cantilever: A leaf-raindrop system,” Phys. Rev. Appl., vol. 3, no. 4, pp.44019, 2015. DOI: 10.1103/PhysRevApplied.3.044019.
- R. E. Pepper, L. Courbin, and H. A. Stone, “Splashing on elastic membranes: the importance of early-time dynamics,” Phys. Fluids, vol. 20, no. 8, pp.82103, 2008. DOI: 10.1063/1.2969755.
- S. Mangili, C. Antonini, M. Marengo, and A. Amirfazli, “Understanding the drop impact phenomenon on soft PDMS substrates,” Soft Matter, vol. 8, no. 39, pp.10045–10054, 2012. DOI: 10.1039/c2sm26049b.
- R. Rioboo et al., “Drop impact on soft surfaces: beyond the static contact angles,” Langmuir, vol. 26, no. 7, pp. 4873–4879, 2009. DOI: 10.1021/la9036953.
- P. B. Weisensee, J. Tian, N. Miljkovic, and W. P. King, “Water droplet impact on elastic superhydrophobic surfaces,” Sci. Rep., vol. 6, pp. 30328, 2016. DOI: 10.1038/srep30328.
- L. Chen, et al., “Static and dynamic wetting of soft substrates,” Curr. Opin. Colloid Interface Sci., vol. 36, pp. 46–57, 2017.
- J. H. Weijs, B. Andreotti, and J. H. Snoeijer, “Elasto-capillarity at the nanoscale: on the coupling between elasticity and surface energy in soft solids,” Soft Matter, vol. 9, no. 35, pp.8494–8503, 2013. DOI: 10.1039/c3sm50861g.
- T. J. Jarvis, M. D. Donohue, and J. L. Katz, “Bubble nucleation mechanisms of liquid droplets superheated in other liquids,” J. Colloid Interface Sci., vol. 50, no. 2, pp.359–368, 1975. DOI: 10.1016/0021-9797(75)90240-4.
- C. H. Wang and V. K. Dhir, “Effect of surface wettability on active nucleation site density during pool boiling of water on a vertical surface,” J. Heat Transfer, vol. 115, pp. 659, 1993. DOI: 10.1115/1.2910737.
- Y. Qi, Heterogeneous Nucleation and Influence of Surface Structure and Wettability, Gainesville, FL:University of Florida, 2005.
- Y. Qi and J. F. Klausner, “Heterogeneous nucleation with artificial cavities,” J. Heat Transfer, vol. 127, no. 11, pp.1189–1196, 2005. DOI: 10.1115/1.2039111.
- S. G. Bankoff, “Entrapment of gas in the spreading of a liquid over a rough surface,” AIChE J., vol. 4, no. 1, pp.24–26, 1958. DOI: 10.1002/(ISSN)1547-5905.
- R. E. Johnson Jr and R. H. Dettre, “Contact angle hysteresis. III. Study of an idealized heterogeneous surface,” J. Phys. Chem., vol. 68, no. 7, pp.1744–1750, 1964. DOI: 10.1021/j100789a012.
- J. J. Lorenz, B. B. Mikic, and W. M. Rohsenow, “Effect of surface conditions on boiling characteristics,” Heat Transfer, vol. 4, pp. 35–39, 1974.
- J. J. Lorenz, The Effects of Surface Conditions on Boiling Characteristics., Cambridge, MA: Massachusetts Institute of Technology, 1972.
- C. H. Wang and V. K. Dhir, “On the gas entrapment and nucleation site density during pool boiling of saturated water,” Trans. Soc. Mech. Eng. J. Heat Transf., vol. 115, pp. 670, 1993. DOI: 10.1115/1.2910738.
- R. Dufour, et al., “Engineering sticky superomniphobic surfaces on transparent and flexible PDMS substrate,” Langmuir, vol. 26, no. 22, pp.17242–17247, 2010. DOI: 10.1021/la103462z.
- M. Liu, J. Sun, and Q. Chen, “Influences of heating temperature on mechanical properties of polydimethylsiloxane,” Sensors Actuators A Phys., vol. 151, no. 1, pp.42–45, 2009. DOI: 10.1016/j.sna.2009.02.016.
- Y. Xia and G. M. Whitesides, “Soft Lithography,” Annu. Rev. Mater. Sci., vol. 28, no. 1, pp.153–184, 1998. DOI: 10.1146/annurev.matsci.28.1.153.
- S. H. Jeong et al., “Mechanically stretchable and electrically insulating thermal elastomer composite by liquid alloy droplet embedment,” Sci. Rep., vol. 5, pp. 18257, 2015. DOI: 10.1038/srep18257.
- M. D. Bartlett et al., “High thermal conductivity in soft elastomers with elongated liquid metal inclusions,” Proc. Natl. Acad. Sci., vol. 114, no. 9, pp. 2143–2148, 2017. DOI: 10.1073/pnas.1616377114.
- N. Kazem, T. Hellebrekers, and C. Majidi, “Soft multifunctional composites and emulsions with liquid metals,” Adv. Mater., vol. 29, no. 27, pp.1605985, 2017. DOI: 10.1002/adma.v29.27.
- M. I. Ralphs et al., “In situ alloying of thermally conductive polymer composites by combining liquid and solid metal microadditives,” ACS Appl. Mater. Interfaces, vol. 10, no. 2, 2018. DOI: 10.1021/acsami.7b15814.
- K. Rykaczewski and R. Y. Wang, “Probability of conductive bond formation in a percolating network of nanowires with fusible tips,” Appl. Phys. Lett., vol. 112, no. 13, pp. 131904, Mar. 2018. DOI: 10.1063/1.5026578.
- R. C. Hedlund, “Silicones,” Paint Varn. Prod., vol. 44, pp. 11, 1954.
- R. F. Brady Jr and I. L. Singer, “Mechanical factors favoring release from fouling release coatings,” Biofouling, vol. 15, no. 1–3, pp.73–81, 2000. DOI: 10.1080/08927010009386299.
- V. G. Damle et al., “‘Insensitive’ to touch: fabric-supported lubricant-swollen polymeric films for omniphobic personal protective gear,” ACS Appl. Mater. Interfaces, vol. 7, no. 7, pp. 4224–4232, 2015. DOI: 10.1021/am5085226.