ABSTRACT
Long non-coding RNAs (lncRNAs) are involved in the process of muscle cell differentiation and play an important role. Previous studies have shown that lncRNA-MEG3 promotes the differentiation of porcine skeletal muscle satellite cells (PSCs), but the regulatory mechanism of MEG3 interaction with target protein has not been well studied. We demonstrated that MEG3 can bind dihydrolipoamide succinyltransferase (DLST) by RNA pull down and RIP-qPCR. Subsequently, knockdown and overexpression experiments showed that DLST promotes PSCs differentiation. Rescue experiments showed that the expression of DLST protein was significantly increased with MEG3 overexpression and decreased with MEG3 knockdown, while its mRNA expression was not changed. Furthermore, we have successfully predicted and validated that the transcription factor myogenic differentiation (MYOD) binds to the MEG3 core promoter though utilizing chromatin immunoprecipitation (CHIP) and luciferase reporter assays. The results indicated that MYOD acts as a transcription factor of MEG3 to promote MEG3 transcription. Knockdown of MEG3 in vivo indicated that MEG3 is involved in skeletal muscle regeneration. It is concluded that MYOD acts as a transcription factor to induce MEG3 expression. MEG3 acts as a molecular scaffold to bind and promote DLST protein expression. This paper provides a new molecular mechanism for MEG3 to promote the differentiation of PSCs.
KEYWORDS:
Introduction
The development of mammalian skeletal muscle is a complex and orderly process, which is usually regulated by some key myogenic genes. In the process of early myogenic directed differentiation regulation, the up-regulation of myogenic factor 5 (MYF5) and MYOD enables myocytes to start the myogenic differentiation programme smoothly. Finally, myogenin (MyoG) and muscle regulatory factor (MRF4) participate in and mediate the expression of terminal differentiation genes, and promote the fusion of myocytes to form multinucleated myotubes [Citation1]. An increasing number of studies have shown that lncRNAs play vital roles in muscle cell proliferation, differentiation, apoptosis, and migration by some different ways.
At present, lncRNAs such as linc-YY1 [Citation2], lnc-SYISL [Citation3], lnc-IRS1 [Citation4], lnc-KCNQLOT1 [Citation5], lnc-MGPF [Citation6], lnc-RAM [Citation7], lnc-MG [Citation8], lnc-MALAT1 [Citation9] and other lncRNAs have been identified to affect myogenic differentiation, and they are mainly used as decoys, signals, scaffolds and recruitment and other forms to function. Lnc-Gm10561 upregulates the expression of myocyte enhancer factor 2C (MEF2C) and E2F transcription factor 3 (E2F3) by acting as a molecular sponge for miR-432 [Citation10], during which the lncRNA acts as a decoy. The expression of Lnc-MyoD can increase the chromatin accessibility of its binding region to MYOD [Citation11]. Studies have shown that lnc-H19 acts as a scaffold to bind to drebrin 1, and can recruit TAR DNA binding protein 43 transcription factor to promote the expression of MYOD [Citation12,Citation13]. Moreover, lnc-AK045171 prevents cardiac hypertrophy by promoting the expression of mitsugumin 53 as well as the phosphorylation of β-catenin, thereby reducing the expression of hypertrophic marker genes [Citation14]. These lncRNAs regulate skeletal muscle myogenesis and regeneration through different mechanisms, indicating the significance of lncRNAs in myogenic differentiation.
MEG3, one of the best known long non-coding RNA, is involved in many biological processes and especially exhibits important functions during myogenic differentiation [Citation15–18]. For example, MEG3 acts as a molecular sponge for miR-135 and promotes bovine myoblast differentiation by up-regulating MEF2C expression [Citation19]. Previous research have proposed that MEG3 upregulates the expression of serum response factor by adsorbing miR-423-5p [Citation20]. In addition, MEG3 exists in multiple single nucleotide loci in pigs and is significantly associated with meat production traits [Citation21,Citation22]. Therefore, it is of great significance to study the effect of MEG3 on myogenic differentiation. To further investigate the molecular mechanism of MEG3 that promotes PSCs differentiation, we used RNA pull down to find the binding protein DLST of MEG3, and explored the effect of DLST on PSCs differentiation. Subsequently, it was confirmed that transcription of MEG3 can be activated by MYOD. This study enriches the regulatory network of MEG3 during myogenic differentiation.
Result
MEG3 directly combined with DSLT
A large number of studies have shown that long non-coding RNAs play the functions of scaffolding, decoy, and signalling in many biological processes. We performed a FISH experiment to detect MEG3 subcellular localization in porcine skeletal muscle satellite cells. The results showed that MEG3 was expressed in both the nuclear and cytoplasmic of myoblast and myotube (). Therefore, to explore MEG3-bound proteins, we performed RNA-binding protein experiments (pull-down) in vitro. We synthesized biotin-labelled MEG3 sense and antisense strand probes, and then used magnetic beads to bind the probes and incubated with 48 h-differentiated satellite cell lysate. The resulting RNA–protein complexes were subjected to SDS-PAGE gel electrophoresis followed by silver staining. Compared with the antisense strand, MEG3 has obvious binding proteins around 45 kDa, and by mass spectrometry analysis, there is a 49-kDa protein – DLST (). To verify the mass spectrometry results, we performed a Western blot experiment, which showed the presence of DLST protein in the sense strand pull-down samples, while the antisense strand did not bind to the DLST protein (). To further confirm the interaction between MEG3 and DLST, we performed reverse validation using RNA immunoprecipitation assay, and the results showed that MEG interacted with DLST in the anti-DLST group compared with the IgG negative control group (). Furthermore, quantitative results showed that DLST was significantly enriched for MEG3 (). The CatRAPID fragment, which is based on the interactions between individual polypeptides and nucleotide fragments, was utilized to make prediction (). Then, a series of truncated mutants of MEG3 were synthesized to perform RNA pull down (). Consistent with the predicted results, three truncated mutants of MEG3 were capable of pulling down DLST (). The above experimental results indicate that MEG3 can bind to DLST protein.
Figure 1. DLST serves as a MEG3 binding protein. (a) Confocal FISH images showing subcellular localization of MEG3 in PSCs. (b) in vitro RNA pull-down assay. After pull down, proteins were subjected to SDS-PAGE and stained by silver. Mass spectrometry analysis of the band circled by the ellipse. (c) Western blotting results showed that sense MEG3 specifically binds to DLST protein, but not with β-tubulin protein(negative control). (d) RNA immunoprecipitation (RIP) results indicate that DLST protein binds MEG3. PKNOX2 protein and lnc-TCONS_00323213 was used as negative control. (e) RIP-qPCR results indicated that MEG3 was significantly enriched by DLST proteins. (f) CatRAPID fragment module prediction of the interaction profile for MEG3 and DLST. The x- and the y-axes represent the indexes of the RNA sequence and the interaction score, respectively. (g) Schematic representation of truncated mutants of MEG3. (h) The interaction of truncated MEG3 and DLST was determined by RNA pull down. Error bars are the mean ± standard error of the mean (SEM) of three biological replicates. Statistical significance of differences was assessed by t-test. *p < 0.05, **p < 0.01.
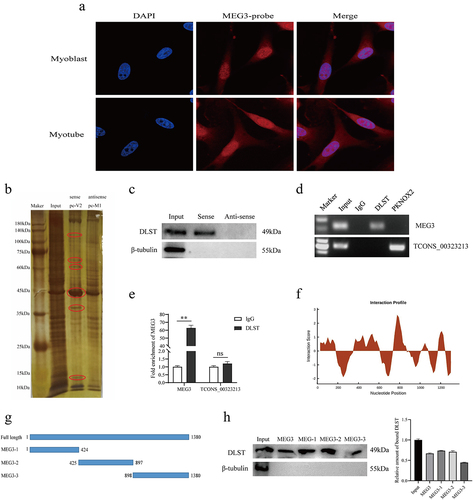
DLST promotes satellite cell differentiation
The PAX7 gene is an important marker for the identification of skeletal muscle satellite cells. The immunofluorescence results showed that the accuracy of purified porcine satellite cells was high, and the purity reached more than 95% (). The immunofluorescence results of satellite cells at the early stage of differentiation showed that the expression of MTOD+ reached 86% (). Based on the binding relationship between MEG3 and DLST protein, we explored the important role of DLST in satellite cell differentiation. First, we analysed the expression profile of DLST in the process of satellite cell proliferation and differentiation. The results showed that the expressions of DLST mRNA did not change significantly during the proliferation phase, but increased significantly from 12 h of differentiation, indicating that DLST may affect satellite cells differentiation (). Subsequently, we designed two interfering RNAs to transfect satellite cells, and the RNA and protein were collected after 48 h of induction differentiation. The results showed that the knockdown efficiency of si-DLST-1 was better and was used in subsequent experiments (). After knockdown of DLST, the mRNA expressions of the key genes MYOD, MYOG, and MYHC in muscle differentiation were significantly lower than those in the control group, while the change in MEG3 expression was not significant (). The protein expression level of the knockdown group was also significantly decreased (). To further verify its function, we transfected DLST overexpression vector into satellite cells, and it did not affect the expression of MEG3 (). The results consistently showed that DLST overexpression significantly increased the mRNA and protein expression levels of MYOD, MYOG, and MYHC ().
Figure 2. DLST promotes porcine satellite cell differentiation. (a) Purification detection of porcine satellite cells. The scales of immunofluorescence pictures are all 100 μm. (b) Identification results of porcine satellite cells during differentiation. The scales of immunofluorescence pictures are all 100 μm. (c) The expression profile of DLST mRNA in the proliferation and differentiation stages of satellite cells, with an interval of 12 h between each stage. (d) Efficiency assay of DLST interfering RNA. (e) The mRNA expressions of MYOD, MYHC, and MYOG were decreased after DLST knockdown, while the expression of MEG3 was not affected. (f) Knockdown of DLST decreased the protein expressions of MYOD, MYHC, and MYOG. (g) Quantitative analysis of western blot results after knockdown of DLST. (h) Efficiency assay of DLST overexpression vector, and DLST overexpression does not affect MEG3 expression. (i) Overexpression of DLST upregulated the mRNA expression levels of MYOD, MYOG, and MYHC. (j) Overexpression of DLST enhanced the protein expression levels of MYOD, MYOG, and MYHC. (k) Quantitative analysis of western blot results after overexpression of DLST. The relative mRNA expression were normalized to those of the control 18S rRNA. Error bars are the mean ± SEM of three biological replicates. Statistical significance of differences was assessed by t-test. *p < 0.05, **p < 0.01.
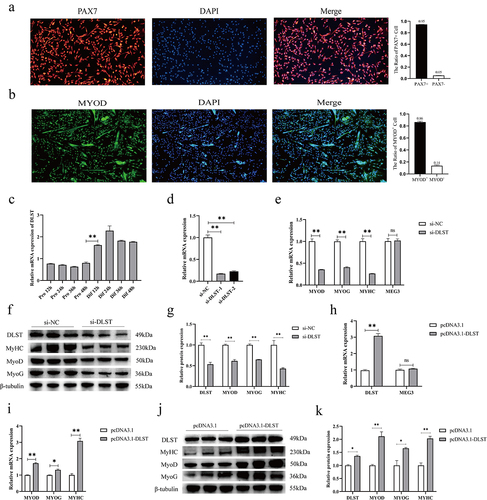
Further, we also verified DLST function in skeletal muscle cell differentiation at the immunofluorescence level. The results showed that DLST affects the formation of myotubes, and can significantly increase the number of myotubes and the number of nuclei in myotubes (). After overexpression of DSLT-, MYOD-, and MYOG-positive cells were significantly increased. Conversely, it was significantly decreased after knockdown of DLST (). Taken together, the results suggest that DLST has a promoting role during porcine skeletal muscle satellite cell differentiation and has no effect on MEG3 expression.
Figure 3. Detection of DLST promotes differentiation at the immunofluorescence level. (a) Myotubes become smaller and less numerous after knockdown of DLST. (b) The number of myotubes and of nuclei within myotubes increased after overexpression of DLST. (c, e) Knockdown of DLST reduced MYOG- (c) and MYOD- (e) positive porcine satellite cells. (d, f) Overexpression of DLST increased MYOG- (d) and MYOD- (f) positive porcine satellite cells. Merge is represented as the proportion of MYHC+, MYOD+, and MYOG+ nuclei relative to total nuclei in one field of view. The scales are all 100 μm. Error bars are the mean ± SEM of three biological replicates. Statistical significance of differences was assessed by t-test. *p < 0.05, **p < 0.01.
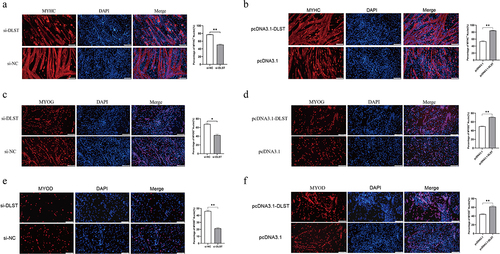
MEG3 affects satellite cell differentiation via DLST protein
In order to further determine the interaction between MEG3 and DLST proteins in regulating satellite cell differentiation, we designed rescue experiments of pcDNA3.1-MEG3+si-DLST co-transfection. The results showed that the mRNA expressions of DLST did not change after overexpression of MEG3 (). Interestingly, overexpression of MEG3 significantly increased the expressions of DLST protein (). qRT-PCR and Western blot results showed that overexpression of MEG3 promoted satellite cell differentiation, while the mRNA and protein expressions of MYOD, MYOG, and MYHC in the pcDNA3.1-MEG3+si-DLST co-transfected group were significantly lower than those in the overexpression of MEG3 group (). The results indicated that knockdown of DLST in satellite cells inhibited the promotion effect of overexpression of MEG3.
Figure 4. MEG3 affects satellite cell differentiation by promoting expression of DLST protein. (a) The results of qRT-PCR showed that the expression of MEG3 was increased and the mRNA expression of DLST was decreased in the pcDNA3.1-MEG3+si-DLST co-transfection group. (b) The results of qRT-PCR showed that the mRNA expressions of marker gene in the MEG3 overexpression group were higher than those in the pcDNA3.1-MEG3+si-DLST group. (c, d) Quantitative analysis of Western blot results, the protein expression levels of marker gene in the MEG3 overexpression group were higher than those in the pcDNA3.1-MEG3 +si-DLST group, and the expression DLST protein in the MEG3 overexpression group was higher than that in the blank control group. (e) The results of qRT-PCR showed that the expression of MEG3 was decreased and the mRNA expression of DLST was increased in the ASO-MEG3+ pcDNA3.1-DLST co-transfection group. (f) The results of qRT-PCR showed that the mRNA expressions of marker gene in the MEG3 knockdown group were lower than those in the ASO-MEG3+pcDNA3.1-DLST group. (g, h) Quantitative analysis of Western blot results, the protein expression levels of marker gene in the MEG3 knockdown group were lower than those in the ASO-MEG3+pcDNA3.1-DLST group, and the DLST protein expression in the MEG3 knockdown group was lower than that in the blank control group. The relative mRNA expression were normalized to those of the control 18S rRNA. Error bars are the mean ± SEM of three biological replicates. Statistical significance of differences was assessed by t-test. *p < 0.05, **p < 0.01.
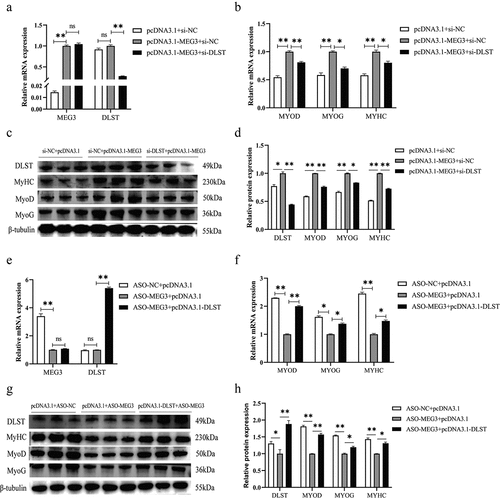
Subsequently, we also performed ASO-MEG3+pcDNA3.1-DLST co-transfection for verification, and the results showed that knockdown of MEG3 did not affect the mRNA expression of DLST (). The mRNA and protein expressions of the three muscle differentiation marker genes in the ASO-MEG3+pcDNA3.1-DLST co-transfected groups were significantly higher than those in the knockdown of MEG3 group. Knockdown of MEG3 also suppressed the expression of DLST protein (). This indicates that overexpression of DLST gene in satellite cells can rescue the inhibitory effect after knockdown of MEG3. This experiment further showed that the effect of DLST on satellite cells and MEG3 can promote the expression of DLST protein. Therefore, we speculate that MEG3 binds DLST protein in satellite cells and promotes its expression to affect the differentiation of porcine skeletal muscle satellite cells.
Transcription factor MYOD regulates the expression of MEG3
We predicted that the transcription factor MYOD has two binding sites E1 and E2 in the MEG3 core promoter region by utilizing JASPAR 2022 database and Animal TFDB 3.0 online tools (). ChIP-qPCR results showed that the enrichment of two sites of MEG3 core promoter was significantly higher in the MYOD group than in the IgG group (). To further explore whether MYOD directly binds to the MEG3 core promoter region, we constructed wild-type and mutant luciferase reporter vectors containing two binding sites (). Results showed that the transfection of pcDNA3.1-MYOD significantly increased the luciferase activity of the MEG3 core promoter (), while the activity was significantly decreased after transfection of MYOD siRNA (). Furthermore, overexpression of MYOD in PSCs significantly increased MEG3 expressions, whereas knockdown of MYOD suppressed MEG3 expressions (). Moreover, we found that the increase of MYOD expressions significantly increased the mRNA and protein expression levels of MEF2C, MYOG, and MYHC (). In contrast, after MYOD knockdown, the expression levels of MEF2C, MYOG, and MYHC were all significantly lower than those in the control group (). The above results indicate that MYOD may promote MEG3 transcription by binding to the region of MEG3 cope promoter, thereby regulating the differentiation of PSCs.
Figure 5. MEG3 is regulated by MYOD to promote satellite cell differentiation. (a) The prediction results of JASPAR online software show that MEG3 core promoter has two MYOD binding sites E1 and E2. (b) CHIP-qPCR results showed that MYOD was bound to the MEG3 cope promoter. (c) Wild-type and mutant core promoter vector construction of MEG3. (d, e) MEG3 cope promoter luciferase assay. Overexpression of MYOD significantly increased the luciferase activity of the MEG3 core promoter, and knockdown of MEG3 inhibited its luciferase activity. However, MYOD had no effect on mutant promoter activity. (f, g) Real-time quantitative PCR results showed that when MYOD was down-regulated, MEG3 expression decreased; when MYOD was up-regulated, MEG3 expression increased. (h) the mRNA expression levels of MYOG, MYHC, and MEF2C after overexpression of MYOD were detected by qRT-PCR. (I) the protein expression levels of MYOG, MYHC and MEF2C after Overexpression of MYOD were detected by western blotting. (j) Quantitative analysis of western blot results after overexpression of MYOD. (k) the mRNA expression levels of MYOG, MYHC, and MEF2C after knockdown of MYOD were detected by qRT-PCR. (l) the protein expression levels of MYOG, MYHC, and MEF2C after knockdown of MYOD were detected by western blotting. (m) Quantitative analysis of Western blot results after knockdown of MYOD. The relative mRNA expression were normalized to those of the control 18S rRNA. Error bars are the mean ± SEM of three biological replicates. Statistical significance of differences was assessed by t-test. *p < 0.05, **p < 0.01.
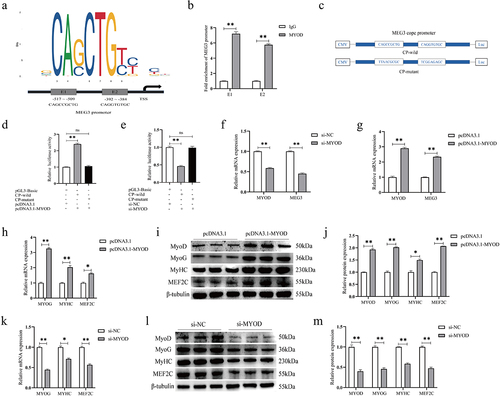
Loss of MEG3 delayed CTX-induced muscle repair in vivo
To further verify whether MEG3 also plays an important role in myogenic differentiation in vivo, we injected ASO oligonucleotides into injured muscles to reduce MEG3 expression in mice. We found high expression in skeletal muscle and lung of mice by tissue expression profiling (). As illustrated in , we injected ASO-MEG3 and ASO-NC on d 1/2, 2, and 4 after CTX injection in the tibialis anterior muscle, and collected muscles on d 6 for follow-up experiments. The qRT-PCR results showed that the expression of MEG3 was significantly decreased on the sixth day (), and the mRNA expression of MYOD, MYOG, and MYHC was significantly lower than that of the control group (). Western blot results showed that MEG3 knockdown inhibited the protein expressions of MYOG, MYOD, and MYHC (). The protein expression of DLST was significantly decreased after ASO-MEG3 injection (). Taken together, the above results suggest that loss of MEG3 may have an inhibitory effect on muscle regeneration in mice.
Figure 6. Knockdown of MEG3 in vivo impairs repair of injured muscle. (a) ASO-MEG3 and ASO-NC were injected into the tibialis anterior muscle injured by CTX. 5 mice per group. (b) Tissue expression profile of MEG3 in mice. (c) MEG3 expression levels after three injections of ASO-MEG3 in the tibialis anterior muscle. (d–f) Real-time quantitative PCR and Western blotting for the mRNA and protein expression levels of MYOD, MYOG, and MYHC were performed on the injected muscles on the sixth day. The relative mRNA expression were normalized to those of the control 18S rRNA. Error bars are the mean ± SEM of three biological replicates. Statistical significance of differences was assessed by t-test. *p < 0.05, **p < 0.01.
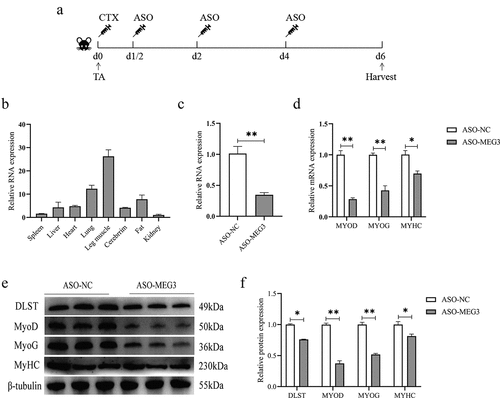
Discussion
MEG3 is located on pig chromosome 7, mouse chromosome 12 and human chromosome 14, which is highly conserved in pigs, mice, and humans [Citation23]. MEG3 is highly expressed in the mesoderm and exhibits differential expression during embryonic development [Citation24]. Previous studies have shown that MEG3 can regulate myoblast differentiation in mice, bovine, and porcine [Citation15,Citation19,Citation21]. Despite published studies, the molecular mechanism by which MEG3 regulates PSCs differentiation remains incomplete. In this study, we revealed a novel molecular mechanism that MEG3 regulates the differentiation of porcine satellite cells ().
Porcine skeletal muscle satellite cells are a type of myoblast. Myoblast differentiation are key steps to skeletal muscle development, and they determine the quantity and quality of meat produced. Therefore, it is particularly essential to understand the underlying mechanisms which lncRNAs regulate myogenesis. The common lncRNA regulatory pattern is that lncRNAs compete with miRNAs to promote the expression of target genes, thus achieving the purpose of regulating biological processes [Citation25]. For example, lnc-SYISL induces muscle atrophy by adsorbing miRNAs such as miR-23a-3p [Citation26]; lnc-MD and H19 also have ceRNA mechanisms to regulate myogenesis [Citation27,Citation28]. In addition, the interaction of lncRNAs with proteins to regulate biological processes is also a vital way for lncRNAs to function. However, depending on the subcellular localization, the regulatory mechanisms of lncRNAs in the nucleus and cytoplasm are different. In the nucleus, lncRNA-Neat1 recruits zeste homolog 2 to the promoter of cell-cycle inhibitor gene P21, leading to the occurrence of H3k27me3 in the promoter of P21 [Citation29]. In the cytoplasm, lncRNA-MGPF interacting with HUR enhances the binding ability of HUR to MYOD and MYOG mRNA, thereby slowing down the degradation rate of MYOD and MYOG mRNA [Citation6]. Previous studies have demonstrated that MEG3 regulates PSCs differentiation through a ceRNA mechanism. In this study, the proteins bound to MEG3 were analysed by RNA pull down and mass spectrometry experiments. The results of the gel map showed that there were proteins specifically bound to MEG3 (). We screened the target protein DLST and performed RIP verification. The results indicate that MEG3 binds to DLST. In addition, the results show that MEG3 also has specific binding to other proteins, how they interact and impact on PSCs needs to be studied in the future.
The DLST is located on chromosome 7 of pigs, and its encoded protein is dihydrolipoate succinyltransferase, which is a core component of the α-ketoglutarate dehydrogenase complex (KGDHC). While KGDHC is the rate-limiting enzyme of the tricarboxylic acid cycle in the mitochondria of cells [Citation30], therefore, the expression of DLST directly affects cellular energy metabolism. Bone marrow mesenchymal cell differentiation is affected by glycolysis [Citation31,Citation32]. Stem cells in the resting state have low energy requirements, while the activation of satellite cells requires a large amount of energy [Citation33]. Therefore, it is speculated that overexpression of DLST can affect the energy metabolism of muscle cells and promote satellite cells differentiation. DLST is less studied in muscle cells. Overexpression of DLST in mouse heart inhibits cardiac hypertrophy and reduces cardiomyocyte cross-sectional area, whereas miR-146a targets the 3´UTR region of DLST mRNA [Citation34]. Studies have also shown that miR-331-3p targets DLST to affect preadipocyte differentiation [Citation35]. In this study, we detect that the mRNA expression level of DLST in PSCs was significantly higher in the differentiation stage than in the proliferation stage, indicating that it was involved in differentiation process. Gain-of-function and loss-of-function experiments demonstrated that DLST can promote differentiation. In the rescue experiment, we found that MEG3 can promote the expression of DLST protein, and DLST overexpression can rescue the differentiation inhibited by knockdown of MEG3. We suspect that there are also miRNAs targeting DLST in PSCs, thereby affecting the expression of DLST protein. In conclusion, the results indicate that DSLT is a downstream regulatory protein of MEG3, and MEG3 promotes PSCs differentiation via promoting the expression of DLST. However, the mechanisms of MEG3 binding to DLST to regulate PSCs differentiation remains to be further studied. In the future, we will aim to thoroughly understand other distinctive functional mechanisms by which MEG3 and DLST regulate PSCs differentiation.
For the upstream of the MEG3, we used JASPAR to predict that MEG3 core promoter has two MYOD binding sites. MYOD has been widely studied as a key factor in muscle differentiation and is also a transcription factor that regulates the expression of myogenic factors [Citation36,Citation37]. Studies have shown that MYOD positively regulates the expression of lncRNA-AK017263 in C2C12 cells, while overexpression of lnc-AK017263 promotes the expression of MYOD, MYOG, and MYHC. Lnc-AK143003 inhibits myocyte differentiation and is negatively regulated by MYOD [Citation38]. Therefore, MYOD and lncRNA may have feedback regulation during muscle cell differentiation. In this paper, we demonstrate that MYOD binds directly to the MEG3 core promoter region and enhances MEG3 transcriptional capacity via CHIP-qPCR and luciferase reporter. Overexpression experiments showed that MYOD could promotes the expression of MEG3, thereby promoting PSCs differentiation. Combined with the results of Chen et al. [Citation20], there is a positive regulatory relationship between MEG3 and MYOD. It was further shown that MEG3 is a key factor affecting muscle myogenic differentiation.
MEG3 is known to affect myoblast differentiation in vitro. Therefore, we further investigated the function of MEG in vivo. The results of muscle injury experiments in mice showed that the mRNA and protein expressions of MYOD, MYOG, and MYHC were significantly decreased in the tibialis anterior muscle after ASO-MEG3 treatment, and indicating that MEG3 knockdown inhibited the repair of muscle injury. Interestingly, the protein expression of DLST is also regulated by MEG3 in vivo, demonstrating the accuracy of vitro experiments on PSCs. These results suggest that MEG3 may be involved in skeletal muscle regeneration. However, the delayed muscle repair caused by MEG3 knockdown is not due to MEG3 inhibiting satellite cell activation [Citation15].
Materials and methods
Ethics statement
Animal care and experiments in this study have been performed in accordance with the National Laboratory Animal Research Council Guidelines for the Care and Use of Laboratory Animals. All experimental protocols were approved by the Animal Care and Use Committee of Huazhong Agricultural University, Wuhan, China (licence HZAUSW2015–0003).
PSCs isolation
All the PSCs were isolated from less than 1-week-old Yorkshire male pigs. The muscle of extremities (including triceps, biceps femoris, semitendinosus, semimembranosus, and gastrocnemius) were collected and kept in PBS supplement with 1% antibiotic-antimycotic. The tissues were dissected and digested with collagenase II (Gibco, Life Technologies, United States, Cat#17101–015, 1 mg/mL) in a 37°C water bath shaking table for 2 h. Digestion was followed by termination with DMEM, including 10% FBS. The mixture was filtered through a 100-μm cell strainer and centrifuged at 2500 r/min for 15 min. Then, we removed the supernatant, and the precipitation was resuspended by PBS and centrifuged at 2200 r/min for 12 min. Next, 70- and 40-μm cell strainers were continued and the supernatant similarly removed and then resuspended with RPMI 1640 (Gibco, United States, Cat#A10491–01) and centrifuged at 2000 r/min for 10 min. Last, the cells were suspended with 20% FBS (Wenren Biotechnology Co., Ltd, Shanghai, Cat#FBS-AUS050) and transferred to the culture dish. After 2.5 h, the cell suspension was transferred to the new culture dishes, which were coated with Matrigel (Corning, BD, United States, Cat# 356234); the cells left in the original cell culture dishes are fibroblasts.
PSCs culture and differentiation
Isolated PSCs were cultured on Matrigel (BD, Cat# 356234) coated 10-cm plates (Corning Cat#430167) in PM + medium (PM + medium containing 76.5% RPMI 1640 (Life, Cat#A10491), 20% FBS (Wenren Biotechnology Co., Ltd, Shanghai, Cat#FBS-AUS050), 0.5% chicken embryo extract (GEMINI, Cat#100-163P), 1% GlutaMax (Gibco, Life Technologies, United States, Cat#35050–061), 1% non-essential amino acids (Gibco, Life Technologies, United States, Cat#11140–050), 1% antibiotic-antimycotic (Gibco, Life Technologies, United States, Cat#15240–062), and 2.5 ng/mL human recombinant basic fibroblast growth factor (Gibco, Invitrogen, United States, Cat#13256029). When cells grow to 70%, they can be inoculated into six-hole plates or new culture dishes for subsequent experiments.
To observe the formation of myotubes, PSCs were differentiated in DMEM containing 5% horse serum (HyClone, Cat#SH30074.02) for different time points, changing the culture medium every day.
Animal experiment
Six-week-old adult C57BL/6 mice were purchased from the Laboratory Animal Center of Huazhong Agricultural University. Fifty microlitres of cardiotoxin (10 µM, CTX; MCE, Shanghai, China, Cat#HY-P1902A) was injected into the tibialis anterior muscle of mice to build a muscle injury model. According to the instructions of Entranster™ in vivo transfection reagent (Engreen Biosystem Co., Ltd.), the experimental group and the control group were respectively injected with 15 µg of antisense oligonucleotide (ASO) against MEG3 (ASO-MEG3; Sangon Biotech, China) and antisense oligonucleotide negative control (ASO-NC; Sangon Biotech, Shanghai, China) on d 1/2, 2, and 4. On the sixth day, mice were sacrificed to collect tibialis anterior muscle and extract total RNA and protein for real-time quantitative PCR (qRT-PCR) and Western blot analysis. Five mice per group were collected and used for the next experiments.
Plasmid construction, RNA oligonucleotide, and transfection
For the construction of MEG3, MYOD, and DLST overexpression vectors, T4 DNA ligase (Takara, Japan, Cat# 6022Q) was used to clone the sequence of MEG3, MYOD, and DLST, then the sequences were inserted into pcDNA3.1 vector. For the MEG3 cope promoter vector, the cope promoter region of MEG3 was cloned into the PGL3 vector. The aforementioned primers are shown in Table S1. The small interfering RNA (siRNA) of MYOD and DLST were purchased from Sangon Biotech (Shanghai, China). The siRNA and the antisense oligonucleotide against MEG3 sequences used are shown in Table S2. For cell transfection, the relevant plasmids or siRNA were conducted with jetPRIME® transfection reagent (USA, Cat#101000046) as advised by the manufacturer’s protocol.
RNA extraction and qPCR assay
We were extract total RNA from PSCs by used Trizol reagent (Takara, Otsu, Japan) to according to the manufacturer’s instructions. Then, we used the ABScriptII cDNA First-Strand Synthesis Kit (Abclonal, Wuhan, China, Cat#RK20400) to carry out cDNA synthesis for mRNA. Analysis of mRNA expression was carried out with 2× Universal SYBR Green FastqPCR Mix (Abclonal, Wuhan, China, Cat#RK21203). The primers associated with qRT-PCR are shown in Tables S1. As previously described, the 2-∆∆CT method was used to analyse the qPCR data.
Western blotting analysis
Total protein was extracted from PSCs and lysed in RIPA lysis buffer with 1% PMSF. The protein concentration was detected with Pierce BCA Protein Assay Reagent (Thermo-Fisher, Waltham, MA, USA). The primary antibodies used were anti-DLST (1:1000, Abclonal, Wuhan, China, Cat#A13297), anti-MYOG (1:1000, Abclonal, Wuhan, China, Cat#A17427), anti-MYOD (1:1000, Proteintech, Wuhan, China, Cat#18943–1-AP), anti-myosin heavy chain (MYHC; 1:3000, Millipore, Darmstadt, Germany, Cat#05–716), and anti-β-tubulin (1:3000, Proteintech, Wuhan, China, Cat#10068–1-AP). The HRP conjugated secondary antibodies (1:4000) HRP-labelled goat anti-mouse IgG (Servicebio, Wuhan, China, Cat#GB23301) and HRP-labelled goat anti-rabbit IgG (Servicebio, Wuhan, China, Cat#GB23303) were used as secondary antibodies.
RNA fluorescent in situ hybridization (FISH)
Cells were briefly rinsed in PBS and fixed in 4% formaldehyde in PBS for 10 min at room temperature. Then, the cells were permeabilized in PBS containing 0.5% Triton X-100.The experiments were performed using a fluorescent in situ hybridization (FISH) Kit (Guangzhou RiboBio Co. Ltd., Guangzhou, China) according to the manufacturer’s instructions in the following steps. Briefly, the cells were incubated with the RNA probes in a hybridization buffer overnight at 37°C. The cells were washed three times with different concentrations of SSC buffer, stained with 4',6-diamidino-2-phenylindole (DAPI) for 10 min at room temperature, and then rinsed in PBS and, finally, examined using a confocal laser-scanning microscope.
RNA pull-down assay
After linearization of the plasmids, T7 RNA polymerase (Roche, Basel, Switzerland, Cat#10881767001) and biotin RNA labelling mix (Roche, Cat#11685597910) were used to synthesize transcripts of the MEG3 v2 full-length [Citation20]. Then, the transcripts were treated with DNase I and EDTA. Meanwhile, proteins were extracted and lysed from PSCs. In vitro biotinylated RNAs (2 µg) were incubated with streptavidin beads overnight, and then pulled down the proteins by complex. The beads were washed five times with wash buffer. Then, the protein complexes associated with beads were analysed by mass spectrometry and western blot. The mass spectrometry results of the sense strand and antisense strand were compared to find the protein that specifically binds to MEG3.
RNA immunoprecipitation (RIP) assay
According to the manufacturer’s instructions, EZ-Magna RIP Kit (Millipore, Boston, USA) was used to carry out RIP assays. Briefly, RIP lysis buffer was used to lyse 107 PSCs, and the lysates were incubated with 10 μg DLST antibody (Abclonal, Wuhan, China, Cat#A13297) at 4°C overnight. Then we added the protein A/G beads to pull down the RNA–protein complex. Reverse-transcription polymerase chain reactions were carried out to detect RNAs.
Immunofluorescence assay
The PSCs were incubated in anti-MYHC (1:500; Milipore, Darmstadt, Germany, Cat#05–716), anti-MYOG (1:500; Abclonal, Wuhan, China, Cat#A17427) and anti-MYOD (1:500; Proteintech, Wuhan, China, Cat#18943–1-AP) overnight at 4°C. Following three washes in PBS, the PSCs were incubated with anti-mouse IgG antibodies (Abclonal, Wuhan, China, Cat#AS008) or anti-rabbit IgG antibodies (Abclonal, Wuhan, China, Cat#AS007) for 60 min. Lastly, the cells were stained with 4',6-diamidino-2-phenyl indole (DAPI) for 10 min and washed thrice with PBS. The images were captured by microscope and dealt with ImageJ software (version 1.48, National Institutes of Health, Bethesda, MD, USA).
Chromatin immunoprecipitation (CHIP)
Chromatin Immunoprecipitation assays were conducted using a ChIP Assay Kit (Beyotime, Shanghai, China, P2078) according to the manufacturer’s instructions. Briefly, the cells were fixed with 37% formaldehyde, neutralized with glycine solution, and then washed thrice with PBS containing 1 mM phenylmethylsulfonyl fluoride (PMSF). Next, the cells were centrifuged and resuspended in SDS lysis buffer containing 1 mM PMSF. After ultrasonic crushing treatment, the samples were resuspended in ChIP dilution buffer containing 1 mM PMSF. Each ChIP reaction was incubated with 10 μg of antibodies against MYOD (Proteintech, Wuhan, China, Cat#18943–1-AP), or IgG (negative control) overnight at 4°C. The next day, 60 μL of protein A + G agarose/salmon sperm DNA was added and the mixture was incubated for 1 h at 4°C. Then, the pulled down DNA was eluted and purified. Fold enrichment was quantified by qPCR.
Dual-luciferase reporter assay
Animal TFDB 3.0 (http://bioinfo.life.hust.edu.cn/AnimalTFDB/#!/) was used to predict the potential TF in the MEG3 promoter. The MYOD-binding motif in the MEG3 promoter was identified using the JASPAR database (https://jaspar.genereg.net/).
When cell confluence reached about 80%, the wild-type and mutant dual-luciferase reporter vectors of MEG3 cope promoter and pcDNA3.1-MYOD plasmids were separately co-transfected into C2C12 cells with pRL-TK normalizing reporter plasmid. After incubation for 36 h, the cells were harvested. The firefly and renilla luciferase activities were measured using Dual-Luciferase Reporter Assay System (Promega, Fitchburg, WI, USA)®.
Statistical analysis
Generally, results are presented as the means ± standard error of the mean (SEM). Statistical comparison between two different groups were assessed by two-tailed Student’s t-test. The p value <0.05 was considered to be statistically significant.
Author contributions
CL conceived and designed the experiments and explained the data. QL, ML, SX, and CT analysed the main content of the data with the help of CL. QL, ML, and SX performed the experiment with the help of LC. QL wrote the manuscript with the help of CL. All authors contributed to the article and approved the submitted version
Supplemental Material
Download Zip (20.5 KB)Acknowledgments
We are grateful for the generous help of ZJL and WJZ in data analysis. In addition, we are also grateful for the funding of the National Natural Science Foundation of China (NSFC, 32172707 and NSFC, 31872322).
Disclosure statement
No potential conflict of interest was reported by the author(s).
Data availability statement
Datasets used in this study are available from the corresponding author upon reasonable request.
Supplementary material
Supplemental data for this article can be accessed online at https://doi.org/10.1080/15592294.2023.2237789.
Correction Statement
This article has been republished with minor changes. These changes do not impact the academic content of the article.
Additional information
Funding
References
- Bentzinger CF, Wang YX, Rudnicki MA. Building muscle: molecular regulation of myogenesis. Cold Spring Harb Perspect Biol. 2012 Feb 1;4(2):a008342. PMID: 22300977; PMCID: PMC3281568. doi: 10.1101/cshperspect.a008342
- Zhou L, Sun K, Zhao Y, et al. Linc-YY1 promotes myogenic differentiation and muscle regeneration through an interaction with the transcription factor YY1. Nat Commun. 2015 Dec 11;6:10026. PMID: 26658965 doi: 10.1038/ncomms10026
- Jin JJ, Lv W, Xia P, et al. Long noncoding RNA SYISL regulates myogenesis by interacting with polycomb repressive complex 2. Proc Natl Acad Sci U S A. 2018 Oct 16;115(42):E9802–E9811. Epub 2018 Oct 2. PMID: 30279181; PMCID: PMC6196504. doi: 10.1073/pnas.1801471115
- Li Z, Cai B, Abdalla BA, et al. LncIRS1 controls muscle atrophy via sponging miR-15 family to activate IGF1-PI3K/AKT pathway. J Cachexia Sarcopenia Muscle. 2019 Apr;10(2):391–16. Epub 2019 Jan 30. PMID: 30701698; PMCID: PMC6463472. doi: 10.1002/jcsm.12374
- Andresini O, Rossi MN, Matteini F, et al. The long non-coding RNA Kcnq1ot1 controls maternal p57 expression in muscle cells by promoting H3K27me3 accumulation to an intragenic MyoD-binding region. Epigenet Chromatin. 2019 Jan 16;12(1):8. PMID: 30651140; PMCID: PMC6334472. doi: 10.1186/s13072-019-0253-1
- Lv W, Jin J, Xu Z, et al. lncMGPF is a novel positive regulator of muscle growth and regeneration. J Cachexia Sarcopenia Muscle. 2020 Dec;11(6):1723–1746. Epub 2020 Sep 20. PMID: 32954689; PMCID: PMC7749533. doi: 10.1002/jcsm.12623
- Zhao Y, Cao F, Yu X, et al. Linc-RAM is required for FGF2 function in regulating myogenic cell differentiation. RNA Biol. 2018 Mar 4;15(3):404–412. Epub 2018 Feb 9. PMID: 29364044; PMCID: PMC5927723. doi: 10.1080/15476286.2018.1431494
- Zhu M, Liu J, Xiao J, et al. Lnc-mg is a long non-coding RNA that promotes myogenesis. Nat Commun. 2017 Mar 10;8:14718. PMID: 28281528; PMCID: PMC5353601 doi: 10.1038/ncomms14718
- Chen X, He L, Zhao Y, et al. Malat1 regulates myogenic differentiation and muscle regeneration through modulating MyoD transcriptional activity. Cell Discov. 2017 Mar 14;3:17002. PMID: 28326190; PMCID: PMC5348715 doi: 10.1038/celldisc.2017.2
- Wang S, Tan B, Xiao L, et al. Long non-coding RNA Gm10561 promotes myogenesis by sponging miR-432. Epigenetics. 2022 Jul;31:1–17. Epub ahead of print. PMID: 35899799. doi: 10.1080/15592294.2022.2105052
- Dong A, Preusch CB, So WK, et al. A long noncoding RNA, LncMyoD, modulates chromatin accessibility to regulate muscle stem cell myogenic lineage progression. Proc Natl Acad Sci U S A. 2020 Dec 22;117(51):32464–32475. Epub 2020 Dec 8. PMID: 33293420; PMCID: PMC7768704. doi: 10.1073/pnas.2005868117
- Li J, Su T, Zou C, et al. Long non-coding RNA H19 Regulates porcine satellite cell differentiation through miR-140-5p/SOX4 and DBN1. Front Cell Dev Biol. 2020 Nov 25 PMID: 33324629; PMCID: PMC7723966;8:518724. doi: 10.3389/fcell.2020.518724
- Li J, Zhao W, Li Q, et al. Long non-coding RNA H19 promotes porcine satellite cell differentiation by interacting with TDP43. Genes (Basel). 2020 Feb 28;11(3):259. PMID: 32121115; PMCID: PMC7140797. doi: 10.3390/genes11030259
- Xu L, Wang H, Jiang F, et al. Correction for: LncRNA AK045171 protects the heart from cardiac hypertrophy by regulating the SP1/MG53 signalling pathway. Aging. 2020 Apr 3. Epub ahead of print. PMID: 32243257. doi: 10.18632/aging.102975
- Dill TL, Carroll A, Pinheiro A, et al. The long noncoding RNA Meg3 regulates myoblast plasticity and muscle regeneration through epithelial-mesenchymal transition. Development. 2021 Jan 15;148(2):dev194027. PMID: 33298462. doi: 10.1242/dev.194027
- Liu Y, Jia L, Min D, et al. Baicalin inhibits proliferation and promotes apoptosis of vascular smooth muscle cells by regulating the MEG3/p53 pathway following treatment with ox‑LDL. Int J Mol Med. 2019 Feb;43(2):901–913. Epub 2018 Nov 30. PMID: 30535498; PMCID: PMC6317676. doi: 10.3892/ijmm.2018.4009
- Bai Y, Zhang Q, Su Y, et al. Modulation of the proliferation/apoptosis balance of vascular smooth muscle cells in atherosclerosis by lncRNA-MEG3 via regulation of miR-26a/Smad1 axis. Int Heart J. 2019 Mar 20;60(2):444–450. Epub 2019 Feb 8. PMID: 30745534. doi: 10.1536/ihj.18-195
- Wang W, Gu MF, Wang ZF, et al. Let-7a-5p regulated by lncRNA-MEG3 promotes functional differentiation to Schwann cells from adipose derived stem cells via directly inhibiting RBPJ-mediating Notch pathway. Apoptosis. 2021 Oct;26(9–10):548–560. Epub 2021 Aug 18. PMID: 34409556. doi: 10.1007/s10495-021-01685-x
- Liu M, Li B, Peng W, et al. LncRNA-MEG3 promotes bovine myoblast differentiation by sponging miR-135. J Cell Physiol. 2019 Aug;234(10):18361–18370. Epub 2019 Mar 18. PMID: 30887511. doi: 10.1002/jcp.28469
- Cheng X, Li L, Shi G, et al. MEG3 promotes differentiation of porcine satellite cells by sponging miR-423-5p to relieve inhibiting effect on SRF. Cells. 2020 Feb 15;9(2):449. PMID: 32075310; PMCID: PMC7072828. doi: 10.3390/cells9020449
- Yang R, Liu Y, Cheng Y, et al. Effects and molecular mechanism of single-nucleotide polymorphisms of MEG3 on porcine skeletal muscle development. Front Genet. 2021 Feb 22 PMID: 33692824; PMCID: PMC7937967;12:607910. doi: 10.3389/fgene.2021.607910
- Yu X, Wang Z, Sun H, et al. Long non-coding MEG3 is a marker for skeletal muscle development and meat production traits in pigs. Anim Genet. 2018 Dec;49(6):571–578. Epub 2018 Oct 8. PMID: 30294799. doi: 10.1111/age.12712
- Li XP, Do KT, Kim JJ, et al. Molecular characteristics of the porcine DLK1 and MEG3 genes. Anim Genet. 2008 Apr;39(2):189–192. Epub 2008 Feb 15. PMID: 18279496. doi: 10.1111/j.1365-2052.2007.01693.x
- Schuster-Gossler K, Bilinski P, Sado T, et al. The mouse Gtl2 gene is differentially expressed during embryonic development, encodes multiple alternatively spliced transcripts, and may act as an RNA. Dev Dyn. 1998 Jun;212(2):214–228. PMID: 9626496. doi: 10.1002/(SICI)1097-0177199806212:2<214:AID-AJA6>3.0.CO;2-K
- Wang H, Zhong J, Zhang C, et al. The whole-transcriptome landscape of muscle and adipose tissues reveals the ceRNA regulation network related to intramuscular fat deposition in yak. BMC Genomics. 2020 May 7;21(1):347. PMID: 32381004; PMCID: PMC7203869. doi: 10.1186/s12864-020-6757-z
- Jin J, Du M, Wang J, et al. Conservative analysis of Synaptopodin-2 intron sense-overlapping lncRNA reveals its novel function in promoting muscle atrophy. J Cachexia Sarcopenia Muscle. 2022 Aug;13(4):2017–2030. Epub 2022 May 19. PMID: 35592920; PMCID: PMC9397560. doi: 10.1002/jcsm.13012
- Sun X, Li M, Sun Y, et al. The developmental transcriptome sequencing of bovine skeletal muscle reveals a long noncoding RNA, lncMD, promotes muscle differentiation by sponging miR-125b. Biochim Biophys Acta. 2016 Nov;1863(11):2835–2845. Epub 2016 Aug 31. PMID: 27589905. doi: 10.1016/j.bbamcr.2016.08.014
- Dey BK, Pfeifer K, Dutta A. The H19 long noncoding RNA gives rise to microRnas miR-675-3p and miR-675-5p to promote skeletal muscle differentiation and regeneration. Genes Dev. 2014 Mar 1;28(5):491–501. Epub 2014 Feb 14. PMID: 24532688; PMCID: PMC3950346. doi: 10.1101/gad.234419.113
- Wang S, Zuo H, Jin J, et al. Long noncoding RNA Neat1 modulates myogenesis by recruiting Ezh2. Cell Death Dis. 2019 Jun 26;10(7):505. PMID: 31243262; PMCID: PMC6594961. doi: 10.1038/s41419-019-1742-7
- Dumont M, Ho DJ, Calingasan NY, et al. Mitochondrial dihydrolipoyl succinyltransferase deficiency accelerates amyloid pathology and memory deficit in a transgenic mouse model of amyloid deposition. Free Radic Biol Med. 2009 Oct 1;47(7):1019–1027. Epub 2009 Jul 22. PMID: 19596066; PMCID: PMC2761144. doi: 10.1016/j.freeradbiomed.2009.07.008
- Lee SY, Long F. Notch signaling suppresses glucose metabolism in mesenchymal progenitors to restrict osteoblast differentiation. J Clin Invest. 2018 Dec 3;128(12):5573–5586. Epub 2018 Nov 12. PMID: 30284985; PMCID: PMC6264656. doi: 10.1172/JCI96221
- Jin Z, Kho J, Dawson B, et al. Nitric oxide modulates bone anabolism through regulation of osteoblast glycolysis and differentiation. J Clin Invest. 2021 Mar 1;131(5):e138935. PMID: 33373331; PMCID: PMC7919726. doi: 10.1172/JCI138935
- Ito K, Suda T. Metabolic requirements for the maintenance of self-renewing stem cells. Nat Rev Mol Cell Biol. 2014 Apr;15(4):243–256. PMID: 24651542; PMCID: PMC4095859. doi: 10.1038/nrm3772
- Heggermont WA, Papageorgiou AP, Quaegebeur A, et al. Inhibition of MicroRNA-146a and overexpression of its target dihydrolipoyl succinyltransferase protect against pressure overload-induced cardiac hypertrophy and dysfunction. Circulation. 2017 Aug 22;136(8):747–761. Epub 2017 Jun 13. PMID: 28611091. doi: 10.1161/CIRCULATIONAHA.116.024171
- Chen T, Cui J, Ma L, et al. The effect of MicroRNA-331-3p on preadipocytes proliferation and differentiation and fatty acid accumulation in laiwu pigs. Biomed Res Int PMID: 31886267; PMCID: PMC6914919. 2019 Dec 4; 2019;2019:1–13. doi: 10.1155/2019/9287804
- Peng XL, So KK, He L, et al. MyoD- and FoxO3-mediated hotspot interaction orchestrates super-enhancer activity during myogenic differentiation. Nucleic Acids Res. 2017 Sep 6;45(15):8785–8805. PMID: 28575289; PMCID: PMC5587775. doi: 10.1093/nar/gkx488
- Harford TJ, Kliment G, Shukla GC, et al. The muscle regulatory transcription factor MyoD participates with p53 to directly increase the expression of the pro-apoptotic Bcl2 family member PUMA. Apoptosis. 2017 Dec;22(12):1532–1542. PMID: 28918507; PMCID: PMC5693709. doi: 10.1007/s10495-017-1423-x
- Guo Y, Wang J, Zhu M, et al. Identification of MyoD-Responsive transcripts reveals a novel long non-coding RNA (lncRNA-AK143003) that negatively regulates myoblast differentiation. Sci Rep. 2017 Jun 6;7(1):2828. PMID: 28588232; PMCID: PMC5460278. doi: 10.1038/s41598-017-03071-7