ABSTRACT
Tri-methylation of Histone 3 lysine 4 (H3K4) is an important epigenetic modification whose deposition and removal can affect the chromatin at structural and functional levels. KDM5A is one of the four known H3K4-specific demethylases. It is a part of the KDM5 family, which is characterized by a catalytic Jumonji domain capable of removing H3K4 di- and tri-methylation marks. KDM5A has been found to be involved in multiple cellular processes such as differentiation, metabolism, cell cycle, and transcription. Its link to various diseases, including cancer, makes KDM5A an important target for drug development. However, despite several studies outlining its significance in various pathways, our lack of understanding of its recruitment and function at the target sites on the chromatin presents a challenge in creating effective and targeted treatments. Therefore, it is essential to understand the recruitment mechanism of KDM5A to chromatin, and its activity therein, to comprehend how various roles of KDM5A are regulated. In this review, we discuss how KDM5A functions in a context-dependent manner on the chromatin, either directly through its structural domain, or through various interacting partners, to bring about a diverse range of functions.
Introduction
The genetic material of a cell (or DNA) is wrapped tightly around the histone core and together this complex, called chromatin, drives various cellular machinery, and is affected by cellular as well as environmental cues. Multiple cell signalling pathways begin at the cell surface and culminate inside the nucleus, where they activate the transcription factors and other chromatin-modifying enzymes to alter chromatin function [Citation1]. One way to tailor chromatin function is through histone modification. Histones are a major part of chromatin, and modification of the tails of histone proteins can have a major effect on chromatin architecture, condensation, and transcription.
One such histone modification is the methylation of lysine residues. Unlike other modifications such as acetylation or phosphorylation, methylation of histone lysine residues was thought to be irreversible. This notion was changed by the discovery of LSD1 (Lysine-Specific Demethylase 1A or KDM1A). LSD1 can carry out the demethylation of H3K4 or H3K9 using flavin as a cofactor [Citation2,Citation3]. LSD1 was found to be capable of removing H3K4me1/me2 marks but not H3K4me3. Subsequently, another family of demethylases containing the JUMONJI domain was discovered, and these proteins were capable of removing H3K4me2/me3 marks [Citation4–8]. All members with JUMONJI domain in KDM family are 2-oxoglutarate and oxygen-dependent. Lysine (K)-specific demethylase 5 (KDM5) is a subfamily which falls under JUMONJI domain KDMs and contains KDM5A (RBP2/JARID1A), KDM5B (PLU-1/JARID1B), KDM5C (SMCX/JARID1C), and KDM5D (SMCY/JARID1D). KDM5A was initially discovered as the Retinoblastoma binding protein 2 (RBP2) [Citation9]. Almost two decades later, in 2007, its histone demethylase activity was discovered, and it was shown to be capable of removing di- and tri-methylation marks from the H3K4 [Citation6].
Even before the demethylase property of KDM5A/RBP2 was known, it was shown to play a role in various cellular processes. After the discovery of the demethylase capabilities of KDM5A, its involvement in diverse cellular processes, such as differentiation, senescence, development, cell cycle regulation, signalling, transcription, and chromatin remodelling, was uncovered [Citation10–17]. In addition, it has been observed that KDM5A plays a vital role in several diseases [Citation18–20]. KDM5A association with various cancers and its potential role in promoting drug resistance have made it a promising candidate for drug development [Citation21–23]. Nevertheless, significant challenges must be overcome to develop efficient and targeted therapeutic approaches involving KDM5A. Despite the emerging importance of KDM5A in various cellular processes and diseases, a mechanistic understanding of its activity, function, and regulation of KDM5A remains lacking. Even with several detailed studies, our appreciation of KDM5A’s engagement with chromatin at molecular level and its wide range of targets, remains limited. It is crucial to determine the recruitment mechanisms of KDM5A to the chromatin, and its activity therein to understand how diverse roles of KDM5A are regulated. KDM5A employs a range of domains/motifs and protein–protein interactions for its recruitment to specific target sites on the chromatin. Various targeting and regulatory interactions play a role in establishing the molecular context for KDM5A functioning. In this review, we have summarized the various structural domains and protein interactions that KDM5A employs for its chromatin recruitment.
Structure of KDM5A protein
KDM5A, a member of the KDM5 family, falls under the sub-group of JUMONJI domain-containing histone demethylases. This subfamily is unique because an AT-Rich Interacting domain (ARID) and Plant Homeodomain (PHD) domains are inserted into the JUMONJI domain, causing it to separate into two domains, JUMONJI N (JmJN) and JUMONJI C (JmJC) (). In addition to these two JUMONJI domains, KDM5A contains several other domains, including an ARID, PHD1, 2, and 3, C5HC2 Zinc finger domain, and a conserved leucine-X-cysteine-X-glutamic acid motif (LxCxE, where × is any amino acid). Although JmJN and JmJC are separated in KDM5A, both these domains protrude along with the C5HC2 Zinc finger domain to form the catalytic cassette [Citation24]. The catalytic domain of KDM5A is JUMONJI C (JmJC) domain, which contains a conserved JmjC motif responsible for the demethylation activity. While the JmJN domain is primarily responsible for providing structural integrity to the molecule, JmJC controls the catalytic activity of the molecule [Citation24]. JmjC-domain demethylases require iron (Fe2+) and 2-oxoglutarate as cofactors to catalyse the removal of the methylation group. They cause decarboxylation of the 2-oxoglutarate to succinate and CO2 in the presence of Fe2+ besides causing hydroxylation of the methylated lysine. A point mutation in two of the three amino acids that are involved in Fe2+ binding, namely H483 or E485, is sufficient to abrogate the demethylation activity of KDM5 proteins [Citation4,Citation6].
Figure 1. Structural and functional components of KDM5A: a) schematic showing various domains of KDM5A: (I) catalytic domain of KDM5A is split into two parts – Jumonji N (JmJN) and Jumonji C domains (JmJC). While JmJC is primarily responsible for catalytic activity of the molecule, JmJN provides the structural integrity to catalytic cassette. (II) ARID domain is the DNA binding domain and recognizes GC rich sequences. (III) PHD fingers- KDM5A has three plant homeodomains. PHD1 along with ARID domain are located between JmJN and JmJC. Along with the zinc finger, these five components form the catalytic cassette of the molecule. PHD1 also shows preferential binding for unmethylated H3K4 residues. While binding preference of PHD2 is not known, PHD3 has been shown to specifically binds H3K4me3 residues. (IV) KDM5 family contain a conserved PLU-1 like domain whose functionality is not known currently. (V) between PHD2 and PHD3 are two domains – LxCxE motif responsible for protein’s interaction with pocket protein family and PID (PAR interacting domain) which is important for PAR recognition and interaction. c) AlphaFold predicted structure of catalytic cassette of KDM5A showing spatial arrangement of different domains is shown: different domains are highlighted in different colours as indicated. Critical residues for different domains that are highlighted – Jumonji C [HIS483, GLU485], ARID [LYS152] and PHD1 [TRP335]. c) AlphaFold predicted structure and spatial arrangement of domain involved in KDM5A catalytic cassette without any linker chains is shown.
![Figure 1. Structural and functional components of KDM5A: a) schematic showing various domains of KDM5A: (I) catalytic domain of KDM5A is split into two parts – Jumonji N (JmJN) and Jumonji C domains (JmJC). While JmJC is primarily responsible for catalytic activity of the molecule, JmJN provides the structural integrity to catalytic cassette. (II) ARID domain is the DNA binding domain and recognizes GC rich sequences. (III) PHD fingers- KDM5A has three plant homeodomains. PHD1 along with ARID domain are located between JmJN and JmJC. Along with the zinc finger, these five components form the catalytic cassette of the molecule. PHD1 also shows preferential binding for unmethylated H3K4 residues. While binding preference of PHD2 is not known, PHD3 has been shown to specifically binds H3K4me3 residues. (IV) KDM5 family contain a conserved PLU-1 like domain whose functionality is not known currently. (V) between PHD2 and PHD3 are two domains – LxCxE motif responsible for protein’s interaction with pocket protein family and PID (PAR interacting domain) which is important for PAR recognition and interaction. c) AlphaFold predicted structure of catalytic cassette of KDM5A showing spatial arrangement of different domains is shown: different domains are highlighted in different colours as indicated. Critical residues for different domains that are highlighted – Jumonji C [HIS483, GLU485], ARID [LYS152] and PHD1 [TRP335]. c) AlphaFold predicted structure and spatial arrangement of domain involved in KDM5A catalytic cassette without any linker chains is shown.](/cms/asset/9be641f9-305f-4a15-9edb-61e12a6c8b37/kepi_a_2268813_f0001_oc.jpg)
All members of the KDM5 family possess either two or three Plant Homeodomains (PHD). KDM5A is no exception to this rule, and contains three PHD domains. PHDs are chromatin readers that recognize and bind histones. The PHD1 domain of KDM5A is responsible for its preferential interaction with the unmethylated H3K4 residues [Citation25]. The binding affinity of PHD1 for any other methylation state of H3K4 is very low compared to the unmethylated state. While not much is known about the role of PHD2, PHD3 is known to stably interact with H3K4me3 mark and drive the recruitment of this protein to the substrate [Citation26]. ARID domain, along with PHD1 is located between the JmJN and JmJC domains. In stark contrast to its name, in vitro experiments have shown that the ARID domain in KDM5A prefers to bind GC-rich sequences [Citation27]. The zinc finger domain, which contains C5HC2 motif, is also a part of the KDM5A catalytic cassette. In addition to its role in the KDM5A catalytic cassette, C5HC2 motif also has the potential to act as a DNA-binding domain owing to the presence of the zinc finger. However, no specific function has been attributed to this domain.
KDM5A contains the LxCxE motif between PHD2 and PHD3 fingers, which is responsible for the interaction of this protein with various pocket proteins (). Previous studies have shown that the pocket proteins contain two pocket-like structures, A and B boxes. Both boxes have a cyclin-fold-like structural organization, wherein the LxCxE motif was shown to bind the B-box [Citation28]. The LxCxE motif-dependent interactions of KDM5A with pocket proteins have been experimentally validated, indicating that this motif is functionally relevant [Citation29,Citation30]. Recently, a Poly ADP Ribose (PAR)-interacting domain (PID) was discovered in KDM5A [Citation31]. This domain lies after the LxCxE motif and allows KDM5A to function at DNA damage sites on replication forks. KDM5A also has an uncharacterized PLU-1 domain which spans residues 741–1072. Initially, the PLU-1 domain was discovered in KDM5B while studying breast cancer cells, and was thought to be linked to an aggressive phenotype in breast cancer. Later, it was found that the PLU-1 domain is conserved throughout the KDM5 family. Although this domain is found in the central region of lysine-specific demethylases, its exact role is not known. A recent structural study on KDM5B suggested that the PLU-1 domain comprises a curved α-helical structure that acts as a rigid linker between the catalytic portion and the rest of the protein [Citation32].
Figure 2. Pocket protein interaction site of KDM5A: KDM5A has a T/E1A binding domain located between PHD2 and PHD3. It contains LxCxE motif which is able to interact with all three pocket proteins pRb, p107 and p130. This interaction can be abrogated by changing a single residue (LxCxE to LxCxK). Beside the LxCxE motif, KDM5A also has a non-T/E1A binding domain through which it can interact with pRb protein. Amino acid residues indicating the position of relevant domains/motifs are shown.
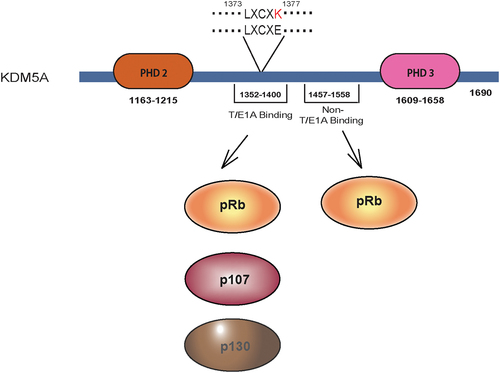
KDM5A shows specificity for H3K4 di- and tri-methylation and can remove methyl group from these sites. Initial studies tested its activity on several states of methylation on H3K4, H3K36, H3K79, and H4K20. However, KDM5A specifically demethylated H3K4me2 and me3, which has conclusively been shown using in vitro demethylation assays [Citation4,Citation6]. Different PHD fingers of KDM5A contribute to this specificity: PHD3 recognizes H3K4me2 and H3K4me3 modifications [Citation26], while PHD1 recognizes unmethylated H3K4 residues [Citation25]. Besides these inherent properties, it has been shown that KDM5A also interacts with several residues on the H3 tail, which specifically enhances H3K4me3 recognition. Some of the residues responsible for the selectivity of KDM5A towards H3K4me3 are H3Q5, H3R2, H3T6 [Citation33]. All these findings suggest that along with various KDM5A domains, chromatin context also plays an important role in H3K4me3 specificity [Citation33].
Activity of KDM5A protein
KDM5A has been shown to be involved in a variety of cellular events, such as differentiation, cell proliferation, replication, DNA repair, epithelial–mesenchymal transition, Notch signalling, metabolism etc [Citation11,Citation14,Citation31,Citation34–39]. KDM5A works in a demethylase-dependent and demethylase-independent manner to regulate the expression of thousands of genes [Citation12,Citation40–42]. Most studies identify with its demethylase role, where KDM5A occupies the promoters of target genes and drives the removal of H3K4me3, leading to repression of gene activity [Citation11,Citation13,Citation42,Citation43]. However, some studies have reported its non-catalytic role in transcriptional regulation [Citation6,Citation40]. A recent in vitro study reported that KDM5 proteins are capable of arginine demethylation (RDM) on H3 and H4 peptides. It was shown that the catalytic domain of all four members of the KDM5 family, including KDM5A, is capable of demethylation of H3R2me2a residues on peptide substrate in vitro [Citation44]. However, in vivo confirmation of KDM5A’s involvement on H3R2me2a is yet to be proven.
Even though different members of the KDM5 family share the ability to remove H3K4 methylation, they work in different pathways in a context-dependent manner. Knockdown of any of these proteins has been shown to only affect the targeted member indicating that they are not regulating each other through a feedback loop [Citation45]. In adipocyte differentiation, it has been shown that simultaneously knocking down KDM5A, 5B and 5C results in significantly greater phenotype compared to when they are individually knocked down. This indicates that this family does have some redundancy and compensation [Citation46]. Out of the four members, KDM5A and KDM5B share the most sequence homology and are very similar in structure. Despite this similarity, both of these proteins have very different physiological functions depending on the cellular context. The interplay between KDM5A and KDM5B is covered in detail in a recent review [Citation47].
Based on initial genome-wide studies, KDM5A target genes were mainly divided into two functionally distinct classes: differentiation-dependent and differentiation-independent. The differentiation-dependent category involves genes that regulate the cell cycle, whereas the differentiation-independent genes include genes that are involved in mitochondrial biogenesis [Citation10,Citation11,Citation15]. During differentiation, KDM5A has been shown to play a critical role by directly regulating the methylation marks on targets such as BRD2, BRD8, and HOX A genes. Interestingly, all three are involved in transcription to regulate specific pathways [Citation4,Citation41,Citation48]. Digressing from the classification above, KDM5A has also been shown to control differentiation by regulating mitochondrial function. Sufficient mitochondrial respiration is necessary for the activation of a gamut of cell signalling pathways responsible for differentiation [Citation15]. KDM5A is a suppressor of various metabolic genes and aids in differentiation by regulating mitochondrial biogenesis [Citation41]. In Rb-negative cells, the loss of KDM5A leads to differentiation rescue [Citation15]. In neural progenitor cells, the mitochondrial damage stimulates KDM5A degradation, which acts as a regulatory checkpoint for neuronal differentiation. KDM5A is involved in the activation of neuronal targets that are responsible for the early stages of differentiation. Owing to the loss of KDM5A, differentiation is suppressed, and hippocampal neurogenesis is inhibited [Citation49]. Thus, this study exemplifies a scenario where KDM5A, together with the mitochondria, acts as a safety check for differentiation to bring about cell-fate specific regulation. Independent of its role in differentiation, KDM5A is also a part of the MPC-1/KDM5A signalling pathway in mitochondria, which regulates pyruvate metabolism in the mitochondria [Citation34].
The involvement of KDM5A in cell cycle regulation has been demonstrated in several studies. In early G1, it regulates E2F4 target genes by suppressing transcription [Citation50]. Along with cell cycle regulation, KDM5A functions cooperatively with E2F4 to maintain gene repression during differentiation [Citation11]. During the late S to G2/M phases of the cell cycle, ORC2 is SUMOylated, and this SUMOylated ORC2 gradually changes its localization to the centromere [Citation37,Citation51,Citation52]. At the centromere, ORC2 recruits KDM5A to demethylate H3K4me3 to H3K4me2 to facilitate transcription. The authors propose that this demethylation is very important to maintain ‘permissive’ epigenetic landscape for alpha-satellite transcription at the centromere [Citation53]. KDM5A is also involved in maintaining the pluripotency of embryonic stem cells. KDM5A works together with several other chromatin regulators to maintain POU5F1 transcription, which is responsible for maintaining pluripotency [Citation54]. In contrast, KDM5A also plays a role in senescence, where it keeps Rb target genes suppressed by demethylation and thus helps maintain the senescence state [Citation12].
During development, KDM5A is involved in the differentiation of multiple tissues, such as neurogenesis, osteogenesis, adipogenesis, and astrogenesis [Citation38,Citation39,Citation46,Citation55]. During adipocyte differentiation, KDM5A is heavily enriched at cell cycle genes and maintains their high expression state for mitotic clonal expansion [Citation46]. A different study showed that KDM5A is transactivated by C/EBPβ, and it inhibits Wnt/β-catenin signalling, which is an important regulator of adipogenesis [Citation56,Citation57]. During astrogenesis, KDM5A reduces GFAP expression by demethylating the GFAP promoter, thereby inhibiting astrocyte differentiation [Citation55]. It is clear from the studies outlined above that KDM5A plays diverse roles that are delineated by the regulatory state, chromatin setting, and context-defined function of a particular region in the genome.
KDM5A involvement in cancer
KDM5A has been found to be associated with various cancers, where it is involved in different aspects of cancer biology, such as tumorigenesis, growth, invasion, and drug resistance. KDM5A is highly expressed in many types of cancers, such as breast cancer, lung cancer, gastric cancer, prostate cancer, and ovarian cancer (). In gastric cancer, lung cancer, and breast cancer, KDM5A overexpression inhibits CDK inhibitors (p16, p21, and p27) [Citation18,Citation19,Citation58–61]. By decreasing CDK inhibitors, KDM5A upregulates the proliferative and invasive abilities of cancer cells. In Her-2 Positive breast cancer cells, KDM5A promotes resistance to Trastuzumab and Erlotinib drugs by downregulating p16 and Bak1 [Citation21,Citation58,Citation59,Citation62]. In ovarian cancer, KDM5A overexpression promotes proliferation and epithelial to mesenchymal transition [Citation63].
Figure 3. Various roles of KDM5A in different type of cancers: KDM5A is overexpressed in many types of cancer where it drives cancer proliferation, invasion and drug resistance. Some of these interaction as shown above are as follows - (I) in acute myeloid leukaemia – NUP98 protein and PHD3 of KDM5A forms a fusion protein which regulate the expression of HOX a gene cluster. This fusion protein alters the expression level of HOX a cluster leading to erroneous differentiation, (II) in ovarian cancer – KDM5A overexpression promotes proliferation and epithelial-to-mesenchymal transition in SKOV3 cells. It was also shown to be playing in PTX resistance in SKOV3 cells, (III) in prostate cancer – KDM5A localizes on tumour suppressor and differentiation genes such as KLF-4 and E-cadherin to reduce their expression. Reduction in these gene correlate with higher malignancy of cancer, (IV) in breast cancer – overexpression of KDM5A drives the drug resistance by downregulating p16 and bak-1. It also helps in cancer proliferation by regulating p27 along with p16, (V) in lung cancer – KDM5A reduces the expression of p16, cyclin D1 etc. To drive cancer proliferation, invasion and drug resistance. In small cell lung cancer, KDM5A causes carcinogenesis by downregulates Notch1 and Notch2, (VI) in gastric cancer – KDM5A promotes the tumour activity by downregulating the cyclin dependent kinase inhibitors (CDKI: p16, p21 & p27). Transactivation of vascular endothelial growth factor (VEGF) by KDM5A also plays important role in gastric tumorigenesis.
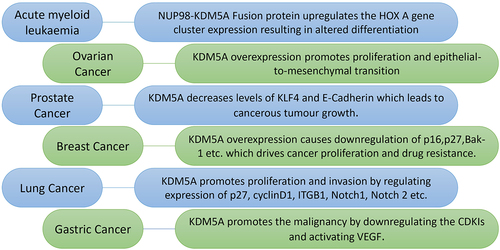
Interestingly, KDM5A overexpression was reported to be negatively correlated with mutations in the tumour suppressor protein p53 [Citation64]. KDM5A downregulates p53 expression by inhibiting translation initiation genes. Deletion of KDM5A results in overexpression of p53 in multiple cancer lineages, and inhibition of tumour growth. This indicates that the suppression of p53 function may be one of the mechanisms by which KDM5A promotes oncogenesis [Citation64].
In acute megakaryoblastic leukaemia, KDM5A is expressed in high amounts and forms a fusion protein with NUP98. The fusion protein includes the N-terminal of NUP98 and PHD3 containing C-terminal of KDM5A [Citation65]. The NUP98/KDM5A fusion protein is responsible for leukaemogenesis, which is driven by increased expression of the HOX A cluster, increased progenitor cell renewal, and inhibition of myeloid differentiation [Citation65–68]. In hepatocellular carcinoma (HCC), KDM5A is negatively regulated by the Homo sapiens microRNA miR-212 (has-miR-212). This KDM5A—hs-miR-212 interaction upregulates CDKIs and leads to cell growth inhibition and senescence. Has-miR-212-KDM5A-CDKI pathway plays a critical role in the pathogenesis of HCC [Citation69]. Because of its association with various oncogenic processes, KDM5A is a potential target for cancer therapy.
Notably, KDM5A is not only considered a therapeutic target for its role in carcinogenesis, but it is also an important therapeutic candidate in other areas. For example, cisplatin is a chemotherapeutic drug used for the treatment of malignant cancer, but it has several side effects including ototoxicity. Inhibition of KDM5A has been shown to prevent the cisplatin-induced hearing loss. KDM5A inhibition reduces the death of hair cells in the cochlea and spiral neuronal ganglions [Citation70]. From these examples, we can see that understanding KDM5A biology is crucial for understanding various aspects of human health.
Chromatin recruitment by protein domains of KDM5A
KDM5A binds to chromatin and regulates transcription, either through its demethylase activity or through its interaction with various chromatin-associated complexes. To understand the biology of KDM5 proteins, it is vital to define their target genes and the mechanisms by which these proteins regulate transcription of these targets. Genome-wide analysis has revealed that KDM5A occupies a large subset of promoters that are involved in the cell cycle, differentiation, and development [Citation11,Citation41]. This seems feasible considering the multiple DNA/chromatin-binding domains present in KDM5A. The structural and functional characteristics of these domains and their ability to recruit KDM5A to chromatin in a context-specific manner are described below:
ARID domain
The ARID domain, which is present between JmJN and JmJC, is a DNA-binding domain that selectively binds to the CCGCCC motif in DNA. NMR studies have revealed that the ARID domain is composed of six helices (H1-H6) and two loops (L1 and L2). Of six helices, three helices, H2, H3, and H4, form a salient U-shape. The DNA-binding interface is composed of loop 1 and a helix-turn-helix motif, which is comprised of H4, L2, and H5. Several residues in the region connecting L2 and H5 are of immense importance and play a critical role in DNA binding [Citation27]. The most notable among these was the K152 residue, as evidenced by the fact that the DNA binding ability of KDM5A was significantly reduced in the K152E mutants. Using a cell line-based reporter assay, the same study reported that this ARID domain residue, that is required for DNA contact, is also important for transcriptional regulation. Furthermore, while the ARID-domain deletion mutant was not able to remove H3K4me3 marks, point mutations/mutants in the same region that abolish DNA-contact of the protein, retained the histone demethylase activity. The authors hypothesize that structural alteration in the vicinity of the JUMONJI domain may adversely affect its catalytic activity due to altered protein structure [Citation27]. In contrast, another study, which deleted the ARID and PHD1 domains, reported minor effects on in vitro enzymatic kinetics of the enzyme [Citation24]. In this study, they have shown that linked JmJN-JmJC, along with C-terminal zinc finger domain, is capable of catalytic activity in vitro. These two studies show conflicting results regarding the importance of the ARID domain in the catalytic activity of KDM5A. The difference could be due to the fact that the first study is only deleting ARID and not PHD1 [Citation27], which probably leads to a change in the catalytic cassette conformation, while the second study [Citation24] is linking JmJN and JmJC domains by deleting both ARID and PHD1, and thus in all probability attains the necessary conformation of JUMONJI domain. Although further in vivo studies examining the role of the ARID domain in the recruitment of KDM5A to chromatin are lacking, several disease-associated mutations have been discovered in the ARID domain of the KDM5C protein. When analysed, some of these mutations (e.g., A77T, D87G) were shown to be important for DNA binding of KDM5C, underscoring the importance of this domain in the functions of KDM5 proteins [Citation71,Citation72].
PHD domains
KDM5A contains three PHD fingers: PHD1, PHD2, and PHD3. PHD fingers, often found as a part of chromatin-modifying enzymes, contain a Cys4-His-Cys3 motif that coordinates two Zn2+ ions in a cross-brace fashion, enabling modification-specific histone-recognition ability of the protein [Citation73,Citation74]. The PHD fingers of KDM5A are known to regulate its substrate specificity and genome occupancy, but whether their function extends beyond this aspect was not initially clear.
PHD1 has been found to have a very high affinity for unmethylated H3K4 in various in vitro qualitative pull-down assays. Although the function of PHD2 is not very clear at present, it has been discovered that deletion of PHD2 destabilizes the protein [Citation36]. PHD3 of KDM5A has been found to be fused with other proteins such as nucleoporin 98 (Nup98) in various cancers [Citation65]. NUP98-KDM5A PHD3 fusion protein was used to study the H3K4me3-binding ‘reader’ potential of PHD3. Structural studies revealed that PHD3 specifically binds to H3K4 tri- and di-methylation marks, although the binding mechanism was slightly different from that of the other well-characterized PHD fingers of proteins, such as BPTF and ING, which involve two rather than four hydrophobic residues to bind H3K4me3 [Citation26]. Even though PHD3-mediated H3K4me3 recognition cannot provide DNA sequence site-specific recruitment, the authors observed that these chimeras were targeted repeatedly to developmentally critical ‘bivalent’ loci enriched for the H3K4me3/2 marks [Citation26]. Another recent report also observed a strong overlap between NUP98–KDM5A fusion protein binding and H3K4me3/H3K27me3 marks from the chromatin immunoprecipitation-sequencing (ChIP-seq) studies in acute myeloid leukaemia cells [Citation75]. Similarly, studies in Drosophila have shown that transgenic flies harbouring point mutations in KDM5A PHD3 (with mitigated binding for H3K4me2 and me3 marks) have severely affected the promoter recruitment of KDM5A to essential mitochondrial genes [Citation76]. As a result, the transcription of these target mitochondrial genes was downregulated. These results were not observed in flies with a PHD1 point mutation, which were abrogated for unmodified H3K4 (H3K4me0) binding, leading the authors to conclude that both PHD1 and PHD3 may utilize distinct mechanisms for their chromatin binding, thereby regulating different sets of target genes [Citation76]. Another study has reported the role of the PHD1 domain of KDM5A in the demethylation of H3K4me3 during DNA damage [Citation36].
The PHD1 domain has been proposed to help not only in the specificity and occupancy of KDM5A but it also causes allosteric enhancement in the demethylation property of the protein after binding to H3K4me0 residues. PHD1 was found to be involved in a positive feedback loop wherein the demethylation of the H3K4me3 residue by KDM5A caused PHD1 to bind to the unmethylated site [Citation25]. This binding, in turn, increased the catalytic activity of the molecule. This was further proven by the fact that the demethylation ability of KDM5A is more prominent in homogenous H3K4me3 nucleosomes. Since PHD1 occupies a position between JmJN and JmJC, it has been proposed that PHD1 increases the catalytic activity by stabilizing the active site [Citation25]. However, some recent discoveries have stated that this enhancement in catalytic power is due to the improved substrate binding rather than the enhanced catalytic turnover [Citation77]. Other studies have reported that ARID and PHD1 domains have negligible effects on the demethylase ability of KDM5A [Citation24] and abrogating H3K4me0 binding by mutation in PHD1 does not affect its demethylase activity [Citation76].
From the studies conducted so far, it can be concluded that different domains in KDM5A work independently or in concert to recruit KDM5A to target sites. However, genome-wide target sites for various domains still need to be defined. While much is known about their role, much more remains to be discovered. For instance, the structures of individual or few adjacent domains have been solved, but how these domains function in the context of full-length protein or chromatin is yet to be understood. In this era of genomics, cryogenic electron microscopy, and AI predictive structures, the need to look at the KDM5A protein as a whole in future structural and functional analyses cannot be over-emphasized.
Chromatin recruitment by protein–protein interactions
Several studies have demonstrated that the histone-modifying enzymes exist as multi-subunit complexes. The advantage of such complexes is that they can bring together enzymes with distinct activities in a coordinated manner to efficiently regulate chromatin remodelling and gene expression [Citation78]. By virtue of their existence in distinct complexes, these enzymes can acquire distinct sets of target genes, where they can discharge context-specific functions. As discussed below, KDM5A has been reported to be a part of diverse multi-protein complexes [Citation36,Citation79,Citation80]. However, other studies have described its function with one or two factors [Citation10,Citation11,Citation13]. Whether other factors of such complexes are yet to be discovered or we are looking at the whole complex, it is clear that the wide-ranging protein–protein interactions confer KDM5A with the ability to occupy several hundred genes as its target loci, especially if there are other chromatin-binding proteins in the complex. Here, we discuss various interacting partners of KDM5A, such as pocket proteins, transcription factors, and chromatin remodellers, which have been shown to be involved in the direct recruitment of KDM5A to chromatin.
Pocket proteins
KDM5A was originally discovered as retinoblastoma (pRB) binding protein-2 (RBP-2). It was shown to be a nuclear phosphoprotein that is a potential interactor of pRB [Citation9,Citation81]. The retinoblastoma (RB) family of proteins, pRB (p105), p107, and p130, are also known as ‘pocket proteins’ because all of them share a homology for a bipartite pocket domain. This pocket domain comprises domains A and B connected by a spacer region [Citation82]. The pocket is not only capable of binding members of the E2F transcription factor family but also other cellular and viral proteins bearing the LxCxE motif. Interestingly, these two sets of interactions are maintained by two distinct regions, which is why pocket proteins can execute both these interactions simultaneously. KDM5A interacts with the pocket domains of all three pocket proteins with the help of its LxCxE motif () [Citation29,Citation50]. A change of one amino acid in this LxCxE motif is sufficient to abrogate the interaction of KDM5A with p107 and p130. However, the interaction between KDM5A and pRB is not limited to the LxCxE motif because KDM5A can also interact with pRB in an LxCxE-independent manner through a small non-T/E1A binding region [Citation29]. Interestingly, KDM5B, which shares high sequence homology with KDM5A, does not possess an LxCxE motif, yet it interacts with pRB using its non-T/E1A binding region [Citation83]. However, its binding to other pocket proteins or the interaction of other KDM5 members with pRB is unknown.
As mentioned above, KDM5A binds to gene promoters, and this binding is highly correlated with the presence of the H3K4me3 mark [Citation41]. KDM5A occupies genes in a differentiation-dependent or differentiation-independent manner. Differentiation-dependent targets include cell cycle genes that show high gene activity in proliferating cells but will be repressed in differentiated cells, while differentiation-independent targets include genes involved in mitochondrial function and DNA/RNA metabolism which are regulated constitutively [Citation41]. pRB plays a central role in the differentiation function of KDM5A. KDM5A was not only discovered as a pRB-binding protein but was also shown to be capable of binding to differentiation-induction competent pRb mutants that were defective in interacting with E2Fs [Citation10]. Along with the confirmation of the functional interaction between pRB and KDM5A, it was discovered that this complex was associated with chromatin, and the interaction with KDM5A was a prerequisite for pRB to induce differentiation (). It is of note that even though KDM5A and pRB were shown to be present together on the same target genes such as BRD2 and BRD8, KDM5A is proposed to be displaced on these promoters by pRB [Citation10]. Interestingly, ablation of KDM5A expression in pRB-deficient cells was sufficient to rescue the defects observed in inducing differentiation, similar to the complementation with pRB [Citation10,Citation84]. Later, studies showed that this rescue was not complete but was achieved in part by restoring mitochondrial gene expression, which was reduced in pRb-deficient cells. KDM5A directly represses mitochondrial genes, and the removal of KDM5A restores mitochondrial function, thereby inducing differentiation in pRB-deficient cells [Citation15].
Figure 4. Models showing various complexes of pocket proteins and KDM5A that are involved in differentiation, proliferation and senescence. (I) during proliferation E2F4 forms a complex with KDM5A with the help of p130. This interaction is limited to early G1 and the model proposes various members such as sin3 HDAC, dREAM complex components and SWI/SNF are a part of this complex to repress transcription at E2F-responsive promoter. (II) during differentiation, KDM5A is part of following complexes; (i) KDM5A interacts with pRb directly to regulate gene activity of BRD2 and BRD8. pRb sequesters KDM5A, preventing the H3K4me3 removal, which leads to increased transcriptional activity at BRD2 and BRD8 promoters. (ii) similar to the complex in proliferating cells, KDM5A interacts with E2F4 indirectly with the help of p130 along with various components like dREAM to form a repressor complex during differentiation. (III) during senescence, pRb, sin3HDAC and KDM5A forms a complex at the E2F responsive promoter, most likely with an E2F transcription factor, to repress transcription.
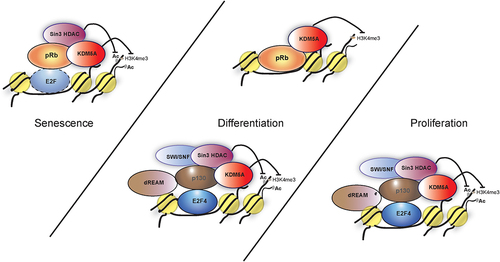
Similar to its complex relationship with pRB, the discovery of KDM5A’s interaction with p130 was also not straightforward and was revealed over the course of multiple studies. Genome-wide ChIP-on-CHIP studies revealed that KDM5A regulates many genes during differentiation, a large number of which are enriched for the H3K4me3 mark [Citation41]. However, the analysis of KDM5A target promoters and nucleosomes bearing H3K4me3 led to the hypothesis that the elements other than H3K4me3 contribute to KDM5A recruitment to chromatin. Scrutiny of these promoter sequences led to the proposal that these elements could be transcription factors, including E2Fs [Citation41]. This hypothesis sounded plausible for two reasons: i) chromatin-binding domains present in KDM5A could not account for site-specific target gene recruitment and ii) the long-standing functional relationship of KDM5A with pRB (which in turn associates with E2Fs). Subsequent genome-wide studies showed that KDM5A co-occupies a large number of E2F4 target promoters [Citation11]. Although E2F4 and KDM5A, along with components of the DREAM complex (including p130), have been shown to be present on specific genomic loci during differentiation, their recruitment was initially thought to be independent of each other [Citation11,Citation48]. This was primarily due to the inability to coprecipitate E2F4 with KDM5A. Eventually, it was shown in cycling cells that KDM5A is associated with E2F4 via the p130 pocket protein [Citation50]. At first, the authors believed that KDM5A interacted directly with E2F4. However, the mutational analysis mapped the E2F4-KDM5A interaction to the transactivation domain (TAD) of E2F4, a domain which is known to confer binding of E2Fs to pocket proteins [Citation50]. When cells were treated with p130 shRNA, the E2F4-KDM5A interaction diminished, leading to the hypothesis that the E2F4-KDM5A interaction was mediated by p130. Mutational studies, in vitro as well as ex-vivo, confirmed that the p130–KDM5A interaction was LxCxE motif dependent, although cellular cues played a role in regulating this interaction [Citation50]. This study used synchronized cells to demonstrate that KDM5A interacts with p130 and E2F4 in a cell cycle-dependent manner, and p130 recruits KDM5A to carry out H3K4me3 demethylation of the E2F-responsive promoters in the early G1 phase. The authors investigated the recruitment mechanism of KDM5A and found that the LxCxE motif-mediated p130 interaction plays a major role in cell-cycle phase-specific recruitment of KDM5A to E2F-responsive promoters (here E2F promoters), whereas the PHD3 domain was used broadly, both at E2F-responsive and non-responsive mitochondrial promoters. When considering these observations in conjunction with research conducted in Drosophila, it is plausible that PHD3 plays an important role in targeting genes enriched for H3K4me3, whereas interactions with individual factors such as pocket proteins (p130 here) provide a more function-specific recruitment mechanism.
Although both KDM5A and p130 are present throughout the cell cycle, their cellular association is restricted to the G1 phase only [Citation50]. A possible hypothesis is that KDM5A, p130, or both undergo post-translational modification during the G1 phase, which makes them amenable to this association. Multiple reports have shown that p130 is differentially phosphorylated during the cell cycle, which enables it to regulate discrete functions, including its interaction with E2F4 [Citation85,Citation86]. Distinct phosphorylated forms of KDM5A have also been reported, including phosphorylation of the residues remarkably close to the LxCxE motif [Citation87]. This phosphorylation-dependent association may not be unique to p130-KDM5A, as KDM5A has also been shown to preferentially bind to the hypo-phosphorylated form of pRB [Citation29]. KDM5A interacts with three different pocket proteins using LxCxE-dependent and -independent surfaces. Additionally, we should consider the potential regulation of these interactions by post-translational modifications or the cellular state, such as cycling versus differentiated cells. These interactions not only allow KDM5A to be a part of multiple protein complexes but also provide KDM5A with a range of opportunities for site-specific recruitment to discharge its functions in a context-dependent manner. As more and more functions of KDM5A in different aspects of cellular processes get discovered, it is likely that the new context-specific interactions of KDM5A with pocket proteins will emerge. Therefore, the interaction with pocket proteins may continue to be an integral part of KDM5A biology.
Transcription factors
KDM5A can act as a transcriptional repressor or an indirect activator, depending upon the interacting partner and the functional setting, either in a demethylation-dependent or independent manner. Being a demethylase, KDM5A is well established as a transcription repressor that removes the active methylation marks from the promoters and causes transcription repression. One example is the repression of E2F transcription factor-targets by removing the H3K4me3 mark [Citation11]. In various other processes, such as the differentiation of astrocytes and adipocytes, the repressor nature of KDM5A plays an important role [Citation46,Citation55]. With the help of various interacting partners, KDM5A can also work as a transcriptional activator. For example, the interacting partner may inhibit or sequester the catalytic activity of KDM5A, thereby allowing others to activate gene expression [Citation10]. The former was shown in a study where KDM5A, when complexed with pRB, promoted the expression of BRD8 promoters [Citation10]. In murine models, KDM5A interacts with CLOCK-BMAL1 and inhibits the acetylation of the Per2 promoter, leading to Per2 upregulation in a demethylase-independent manner () [Citation40].
Figure 5. Various protein–protein interactions of KDM5A for its chromatin recruitment: KDM5A is recruited by its many partners to the chromatin to regulate the transcription in a demethylase dependent/independent manner. Some of these interactions, as shown above, are as follows: (I) cell cycle – KDM5A interacts with pocket protein family member – p130 and is recruited to E2F4 responsive promoter in early G1 for repression of target genes, (II) notch signalling – KDM5A is recruited by RBP-J to various notch target gene to regulate their transcription, (III) IFN signalling – Piasy interacts with JmJC domain of KDM5A and recruits it to IFNI promoter. KDM5A represses the IFNI gene activity by removing H3K4me3 marks, (IV) differentiation – during development, KDM5A interacts with chromatin remodellers such as sin3B and NuRD. KDM5A, along with NuRD, controls developmentally regulated genes, (V) DNA damage repair – upon DNA damage, PARP1 generates PAR chains at the damage sites which helps in recruitment of KDM5A. KDM5A carries out the transcriptional repression, (VI) gene activity – KDM5A is also shown to interact with MAD1 transcription factor to regulate the gene activity by controlling H3K4me3 levels, (VII) circadian clock maintenance – KDM5A works in a demethylase independent manner and prevents the interaction of HDAC1 with CLOCK-BMAL1. Accumulation of H3K4me3 and H3K9ac leads to higher gene expression. (please see the text for details).
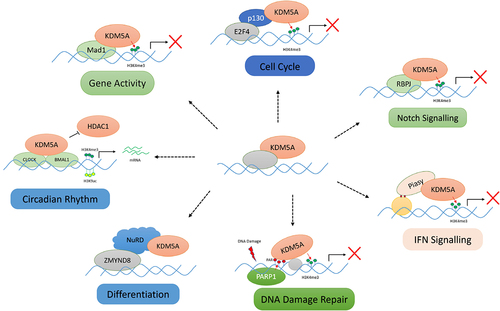
KDM5A has been shown to interact with various transcription factors. Below are some examples where interaction with KDM5A or its recruitment to chromatin has been shown experimentally. One prominent family of transcription factors is Mad. MYC/MAX/MAD are transcription factors that are a part of the helix-loop-helix/leucine zipper family and regulate various aspects of cell proliferation and differentiation [Citation88]. They regulate gene-specific transcription and activation by modulating interactions among themselves. Myc forms dimers with Max to bind to target promoters in a sequence-specific manner and activate gene expression. This dimer recruits histone acetyltransferases, which carry out histone acetylation, leading to an open chromatin structure [Citation89,Citation90]. Mad-Max dimer also binds to the same sequences but causes gene repression via recruitment of the mSin3-containing histone deacetylases complexes [Citation91].
In Drosophila, it has been shown that Myc forms a complex with Little imaginal discs (Lid; ortholog of KDM5A) and inhibits its demethylation activity on chromatin. A study reported genetic and physical interactions between the two proteins. The interaction of KDM5A with this family in Drosophila raises the possibility that KDM5A could also play a similar role in humans [Citation92,Citation93]. However, when another study attempted to reproduce these findings in undifferentiated HL60 cells (high Myc expression), no such interaction between c-Myc and KDM5A was detected. Instead, the Mad1 protein was found to interact with KDM5A in differentiated HL60 cells (with high Mad1 expression). This complex was then discovered to be targeted to the hTERT gene promoter, where KDM5A carried out demethylation and repression of hTERT mRNA expression [Citation13]. In contrast to these observations, KDM5A has been shown to promote Myc target genes in multiple myeloma. The authors used ChIP-seq and RNA-seq to demonstrate a significant overlap between KDM5A and Myc target genes and used a KDM5A inhibitor as a therapeutic target for multiple myeloma [Citation45]. These studies highlight how the MYC/MAX/MAD family uses distinct interactions to recruit KDM5A in various cellular contexts.
In a murine cell line, the transcription factor RBP-J recruits KDM5A to chromatin. KDM5A has been shown to interact with the DNA-bound RBP-J via its C-terminal domain, including the PHD3 domain. The KDM5A-RBP-J complex has been found on various Notch target genes, where KDM5A removes H3K4me3 marks and regulates gene expression. The binding of KDM5A to these promoters was reduced upon RBP-J knockdown but remained unchanged on Notch/RBP-J-independent KDM5A target promoters [Citation14]. A similar mechanism was observed in Drosophila between Lid and Su(H) (homolog of RBP-J). Another study reported the RBP-J/KDM5A complex on the mitotic chromatin, where the occupancy of this complex was negatively regulated by HDAC1 [Citation35]. In humans, it was discovered that KDM5A’s repression of Notch signalling resulted in sustained levels of the neuroendocrine transcription factor ASCL1, promoting small cell lung cancer (SCLC) proliferation and tumorigenesis. The authors suggested the inhibition of KDM5A as a possible therapeutic strategy to reduce ASCL1 expression in SCLC [Citation38]. All the above examples demonstrate the range of transcription factors that recruit KDM5A to the chromatin in a cell- and function-specific context.
Chromatin remodellers
Chromatin remodellers are responsible for maintaining and changing chromatin architecture according to cellular requirements. Chromatin remodellers regulate gene expression by controlling the access of the transcriptional machinery to the chromatin. Sin3B-containing histone deacetylase, and Nucleosome Remodelling and histone Deacetylation (NuRD) complex are two such chromatin remodellers that are involved in transcription regulation. Both chromatin remodellers show physical interaction and functional cooperation with KDM5A [Citation94]. The NuRD core complex consists of HDAC1/2, histone-binding protein RbAp46/48, ATP-dependent chromatin remodeller Mi-2α/β (CHD3/CDH4), metastasis-associated factor (MTA1/MTA2/MTA3), methyl-DNA-binding protein (MBD2/MBD3), and GATAD2. This complex contains both histone demethylation and chromatin remodelling abilities. The NuRD complex is associated with a transcriptional co-regulator complex composed of ZMYND8, ZNF592, and ZNF 687— the Z3 complex [Citation95]. Although the Z3 complex is associated with various histone demethylases, it is believed that KDM5A interacts indirectly with ZMYND8 through the NuRD complex. In association with various transcriptional regulators, NuRD and KDM5A play key roles in developmental pathways and tumorigenesis by cooperatively regulating H3K4m2/3 levels [Citation94]. Gene expression studies have revealed that CHD4 and KDM5A share hundreds of developmentally regulated gene targets. CHD4, an ATP-dependent chromatin remodelling factor and a catalytic subunit of the NuRD complex, cooperatively regulates methylation levels with KDM5A [Citation94]. KDM5A promotes the recruitment of CHD4 at target sites, but how exactly CHD4 plays a role in this instance is not known. The authors speculate that there may be changes in the nucleosome occupancy due to the action of the NuRD complex [Citation94].
Another study highlighted the importance of KDM5A in the functional activities of the NuRD complex during DNA damage repair and showed that the KDM5A deficiency results in impaired DSB repair [Citation36]. In the presence of DNA damage, the PHD1 domain of KDM5A drives the recruitment of demethylases to the target sites. Once recruited at the damage site, KDM5A carries out the demethylation of H3K4me3. The demethylation activity, as well as both the PHD1 and PHD3 domains, promotes the recruitment of the bromodomain protein ZMYND8. Ultimately, ZMYND8 is responsible for recruiting the NuRD complex at the DNA damage site to carry out DNA repair. This study highlights the role of KDM5A in modifying the local chromatin state at DNA damage sites to promote repair via the ZMYND8–NuRD complex, and to maintain genomic integrity [Citation36]. Another study reported that KDM5A is a component of the MRG15 interactome. MRG15 is a chromodomain protein that recognizes H3K36me and is a component of various HDAC complexes. As KDM5A reduced the H3K4 methylation of actively transcribed genes and MRG15 was colocalized with nuclear subdomains enriched for elongating RNA polymerase II, the authors suggest that MRG15 complexes aid in the recruitment of H3K4 demethylases to regions rich in H3K36 methylation. This highlights the functional interplay between H3K4 methylation and H3K36 methylation [Citation79].
Recent protein–protein interaction studies have revealed that KDM5A, GATAD1, EMSY, and components of Sin3B HDAC form a novel protein complex called the EMSY/KDM5A/SIN3B complex (referred to as the EMSY complex). This complex is similar to the Lid/sin3 complex discovered in Drosophila and Sin3B uniCORE complex in human cells. The EMSY complex, with the help of ZNF131, is recruited to various active promoters enriched for the H3K4me3 mark, and regulates the expression of its target genes [Citation80]. Various subunits of this complex are overexpressed in primary breast cancer. Although this study does not shed light on how KDM5A may be recruited to the chromatin, it reinforces the occurrence of KDM5A with Sin3/HDAC in a complex. Despite the belief that KDM5A is not a stable component of these complexes, there is no denying that it plays an important role in various interactions of these complexes on the chromatin.
Other diverse interacting partners
In addition to the activities of KDM5A with the NuRD complex in DNA damage repair, the involvement of this demethylase in other DNA repair pathways has been reported in several studies [Citation36,Citation58,Citation96]. However, the recruitment of KDM5A to these DNA lesions was not clearly understood. A recent study showed that KDM5A contains a non-canonical PID domain [Citation31]. When this region is mutated or when there are PAR Polymerase inhibitors that block the KDM5A-PAR interaction, KDM5A-mediated DNA repair functions are blocked. It was also shown that the histone variant macro H2A1.2 is specifically required for the recruitment of KDM5A to the DNA damage site. This variant has been shown to be involved in homology-directed dsDNA breaks and repression of transcription at DNA damage sites. The authors proposed PAR-binding and macro H2A1.2-based recruitment as two key factors that facilitate the binding of KDM5A to DNA damage sites.
IFN signalling, a crucial aspect of the immune system, is also associated with KDM5A. KDM5A has been reported to interact with p50, and via this interaction, KDM5A is recruited to the promoter of the suppressor of cytokine Signalling 1(SOCS1), where KDM5A causes an increase in IFN-γ production in NK cells [Citation97]. E-Cadherin promoter is also one of the proposed recruitment sites of KDM5A [Citation98]. Furthermore, KDM5A interacts with Piasy to regulate IFNI signalling. Piases belonging to the family of pias (protein inhibitor of activated signal transducer and activator of transcription) play a crucial role in reducing IFNI gene transcription. This interaction between KDM5A and Piasy is driven by the PINIT domain of Piasy and JmJC domains of KDM5A. Piasy recruits KDM5A to IFNI gene promoters and drives their repression by removing the activating H3K4me3 marks [Citation99].
In a demethylation-independent manner, KDM5A is also capable of potentiating the nuclear hormone receptor-mediated transcription. Nuclear receptors are transcription factors that promote transcription of target genes upon ligand stimulation. KDM5A interacts with the DNA-binding domain of nuclear receptors with the help of its C-terminus. Both the N- and C-termini of KDM5A are important for the transactivation activity of KDM5A. In this context, KDM5A acts as a co-regulator [Citation100].
Conclusion
Generally, transcription occurs as the sum total of many dynamic changes occurring at the chromatin on a few or genome-wide loci. External or internal signals often prompt chromatin-modifying enzymes to act in multi-subunit complexes to exert their regulatory roles. On the surface, KDM5A also appears to be an enzyme that is a member of multi-protein complexes and regulates thousands of loci depending on the cellular context. However, it is unclear whether KDM5A is a permanent member of such complexes or transiently associates with them in a functional context. Does KDM5A require other proteins in the complex to efficiently discharge its functions (and vice versa) or can it function in their absence? Furthermore, given that KDM5A itself has functionally defined chromatin-binding domains, what is the role of the interacting partners in the recruitment of KDM5A to the chromatin. In addition, KDM5A functions are not limited just to its demethylase activity, it also has roles that are independent of the demethylation-driven process. In both instances, KDM5A interacts with a large number of interacting partners to drive various aspects of the cellular machinery, such as differentiation, cell cycle regulation, DNA repair pathways, and circadian rhythm maintenance.
This epigenetic modulator possesses multiple domains that are responsible for its interactions with various partners. Here, we reviewed these domains and their interactions with different partners that are ultimately responsible for the multifaceted involvement of KDM5A in various processes. Inherently, ARID and PHD domains carry out the chromatin recruitment of KDM5A, but the indirect recruitment through proteins such as pocket proteins is also a critical component. Through its interaction with other proteins, KDM5A plays a role in a multitude of pathways. Multiple known and unknown regions within KDM5A are responsible for its interaction with other proteins. To understand the involvement of KDM5A in these pathways, we need to determine how it interacts with multiple partners and what these interactions entail. As we discover more about these interactions, we will also be able to discover how KDM5A plays other roles that are currently unknown.
Author contributions
S.T. conceived and designed the content of the review. A.K. and S.T. wrote the manuscript and prepared figures.
Acknowledgments
We thank R. Kaur for critical reading of the manuscript. A.K. is a recipient of the Junior and Senior Research Fellowships of the Council of Scientific and Industrial Research, India, towards the pursuit of a PhD degree at Manipal University.
Disclosure statement
No potential conflict of interest was reported by the author(s).
Data availability statement
Data sharing is not applicable to this article as no new data were created or analysed in this study.
Additional information
Funding
References
- Fisher CL, Fisher AG. Chromatin states in pluripotent, differentiated, and reprogrammed cells. Curr Opin Genet Dev. 2011 Apr;21(2):140–19. doi: 10.1016/J.GDE.2011.01.015
- Shi Y, Lan F, Matson C, et al. Histone demethylation mediated by the nuclear amine oxidase homolog LSD1. Cell. 2004 Dec;119(7):941–953. doi: 10.1016/j.cell.2004.12.012
- Carnesecchi J, Forcet C, Zhang L, et al. ERRα induces H3K9 demethylation by LSD1 to promote cell invasion. Proc Natl Acad Sci U S A. 2017 Apr;114(15):3909–3914. doi: 10.1073/PNAS.1614664114/SUPPL_FILE/PNAS.1614664114.SD01.XLSX
- Christensen J, Agger K, Cloos PAC, et al. RBP2 belongs to a family of demethylases, specific for tri-and dimethylated lysine 4 on histone 3. Cell. 2007;128(6):1063–1076. doi: 10.1016/j.cell.2007.02.003
- Iwase S, Lan F, Bayliss P, et al. The X-linked mental retardation gene SMCX/JARID1C defines a family of histone H3 lysine 4 demethylases. Cell. 2007 Mar;128(6):1077–1088. doi: 10.1016/J.CELL.2007.02.017
- Klose RJ, Yan Q, Tothova Z, et al. The retinoblastoma binding protein RBP2 is an H3K4 demethylase. Cell. 2007 Mar;128(5):889–900. doi: 10.1016/J.CELL.2007.02.013
- Lee MG, Norman J, Shilatifard A, et al. Physical and functional association of a trimethyl H3K4 demethylase and Ring6a/MBLR, a polycomb-like protein. Cell. 2007 Mar;128(5):877–887. doi: 10.1016/J.CELL.2007.02.004
- Tahiliani M, Mei P, Fang R, et al. The histone H3K4 demethylase SMCX links REST target genes to X-linked mental retardation. Nature. 2007 May;447(7144):601–605. doi: 10.1038/NATURE05823
- Defeo-Jones D, Huang PS, Jones RE, et al. Cloning of cDnas for cellular proteins that bind to the retinoblastoma gene product. Nature. 1991;352(6332):251–254. doi: 10.1038/352251a0
- Benevolenskaya EV, Murray HL, Branton P, et al. Binding of pRB to the PHD protein RBP2 promotes cellular differentiation. Mol Cell. 2005;18(6). doi: 10.1016/j.molcel.2005.05.012
- Beshiri ML, Holmes KB, Richter WF, et al. Coordinated repression of cell cycle genes by KDM5A and E2F4 during differentiation. Proc Natl Acad Sci U S A. 2012 Nov;109(45):18499–18504. doi: 10.1073/pnas.1216724109
- Chicas A, Kapoor A, Wang X, et al. H3K4 demethylation by Jarid1a and Jarid1b contributes to retinoblastoma-mediated gene silencing during cellular senescence. Proc Natl Acad Sci U S A. 2012;109(23):8971–8976. doi: 10.1073/pnas.1119836109
- Ge Z, Li W, Wang N, et al. Chromatin remodeling: recruitment of histone demethylase RBP2 by Mad1 for transcriptional repression of a Myc target gene, telomerase reverse transcriptase. FASEB J. 2010 Feb;24(2):579–586. doi: 10.1096/FJ.09-140087
- Liefke R, Oswald F, Alvarado C, et al. Histone demethylase KDM5A is an integral part of the core Notch–RBP-J repressor complex. Genes Dev. 2010 Mar;24(6):590–601. doi: 10.1101/gad.563210
- Váraljai R, Islam ABMMK, Beshiri ML, et al. Increased mitochondrial function downstream from KDM5A histone demethylase rescues differentiation in pRB-deficient cells. Genes Dev. 2015 Sep;29(17):1817–1834. doi: 10.1101/GAD.264036.115
- Dimitrova E, Turberfield AH, Klose RJ. Histone demethylases in chromatin biology and beyond. EMBO Rep. 2015 Dec;16(12):1620–1639. doi: 10.15252/EMBR.201541113
- Pavlenko E, Ruengeler T, Engel P, et al. Functions and interactions of mammalian KDM5 demethylases. Front Genet. 2022 Jul;13:906662. doi: 10.3389/fgene.2022.906662
- Teng YC, Lee C-F, Li Y-S, et al. Histone demethylase RBP2 promotes lung tumorigenesis and cancer metastasis. Cancer Res. 2013 Aug;73(15):4711–4721. doi: 10.1158/0008-5472.CAN-12-3165
- Li L, Wang L, Song P, et al. Critical role of histone demethylase RBP2 in human gastric cancer angiogenesis. Mol Cancer. 2014;13(1). doi: 10.1186/1476-4598-13-81
- Spangle JM, Dreijerink K, Groner A, et al. PI3K/AKT signaling regulates H3K4 methylation in breast cancer. Cell Rep. 2016 Jun;15(12):2692–2704. doi: 10.1016/j.celrep.2016.05.046
- Choi HJ, Joo H-S, Won H-Y, et al. Role of RBP2-induced ER and IGF1R-ErbB signaling in tamoxifen resistance in breast cancer. J Natl Cancer Inst. 2018 Apr;110(4):400–410. doi: 10.1093/JNCI/DJX207
- Garcia TB, Uluisik RC, van Linden AA, et al. Increased HDAC activity and c-MYC expression mediate acquired resistance to WEE1 inhibition in acute leukemia. Front Oncol. 2020 Mar;10:296.
- Yan H, Chen X, Zhang Q, et al. Drug-tolerant cancer cells show reduced tumor-initiating capacity: depletion of CD44 cells and evidence for epigenetic mechanisms. PLoS One. 2011 Sep;6(9):e24397. doi: 10.1371/JOURNAL.PONE.0024397
- Horton JR, Engstrom A, Zoeller EL, et al. Characterization of a linked Jumonji domain of the KDM5/JARID1 family of histone H3 lysine 4 demethylases. J Biol Chem. 2016 Feb;291(6):2631–2646. doi: 10.1074/JBC.M115.698449
- Torres IO, Kuchenbecker KM, Nnadi CI, et al. Histone demethylase KDM5A is regulated by its reader domain through a positive-feedback mechanism. Nat Commun. 2015;6(1). doi: 10.1038/ncomms7204
- Wang GG, Song J, Wang Z, et al. Haematopoietic malignancies caused by dysregulation of a chromatin-binding PHD finger. Nature. 2009;459(7248):847–851. doi: 10.1038/nature08036
- Tu S, Teng Y-C, Yuan C, et al. The ARID domain of the H3K4 demethylase RBP2 binds to a DNA CCGCCC motif. Nat Struct Mol Biol. 2008;15(4):419–421. doi: 10.1038/nsmb.1400
- Lee JO, Russo AA, Pavletich NP. Structure of the retinoblastoma tumour-suppressor pocket domain bound to a peptide from HPV E7. Nature. 1998 Feb;391(6670):859–865. doi: 10.1038/36038
- Kim YW, Otterson GA, Kratzke RA, et al. Differential specificity for binding of retinoblastoma binding protein 2 to RB, p107, and TATA-binding protein. Mol Cell Biol. 1994 Nov;14(11):7256–7264. doi: 10.1128/MCB.14.11.7256
- Palopoli N, Foutel NSG, Gibson TJ, et al. Short linear motif core and flanking regions modulate retinoblastoma protein binding affinity and specificity. Protein Eng Des Sel. 31(3): Oxford University Press:69–77. Mar. 1, 2018. 10.1093/protein/gzx068
- Kumbhar R, Sanchez A, Perren J, et al. Poly(adp-ribose) binding and macroH2A mediate recruitment and functions of KDM5A at DNA lesions. J Cell Bio. 2021;220(7). doi: 10.1083/jcb.202006149
- Dorosz J, Kristensen LH, Aduri NG, et al. Molecular architecture of the Jumonji C family histone demethylase KDM5B. Sci Rep. 2019 Dec;9(1). doi: 10.1038/S41598-019-40573-Y
- Petronikolou N, Longbotham JE, Fujimori DG. Extended recognition of the histone H3 tail by histone Demethylase KDM5A. Biochemistry. 2020;59(5). doi: 10.1021/acs.biochem.9b01036
- Cui J, Quan M, Xie D, et al. A novel KDM5A/MPC-1 signaling pathway promotes pancreatic cancer progression via redirecting mitochondrial pyruvate metabolism. Oncogene. 2019;39:(5):1140–1151. vol. 39, no. 5,. doi: 10.1038/s41388-019-1051-8
- Dreval K, Lake RJ, Fan HY. HDAC1 negatively regulates selective mitotic chromatin binding of the Notch effector RBPJ in a KDM5A-dependent manner. Nucleic Acids Res. 2019 May;47(9):4521–4538. doi: 10.1093/nar/gkz178
- Gong F, Clouaire T, Aguirrebengoa M, et al. Histone demethylase KDM5A regulates the ZMY ND8-NuRD chromatin remodeler to promote DNA repair. J Cell Bio. 2017 Jul;216(7):1959–1974. doi: 10.1083/jcb.201611135
- Lee KY, Bang SW, Yoon SW, et al. Phosphorylation of ORC2 protein dissociates origin recognition complex from chromatin and replication origins. J Biol Chem. 2012 Apr;287(15):11891. doi: 10.1074/JBC.M111.338467
- Oser MG, Sabet AH, Gao W, et al. The KDM5A/RBP2 histone demethylase represses NOTCH signaling to sustain neuroendocrine differentiation and promote small cell lung cancer tumorigenesis. Genes Dev. 2019;33(23–24):1718–1738. doi: 10.1101/gad.328336
- Wang C, Wang J, Li J, et al. KDM5A controls bone morphogenic protein 2-induced osteogenic differentiation of bone mesenchymal stem cells during osteoporosis. Cell Death Dis. 2016;7(8):e2335–e2335. doi: 10.1038/CDDIS.2016.238
- DiTacchio L, Le HD, Vollmers C, et al. Histone lysine demethylase JARID1a activates CLOCK-BMAL1 and influences the circadian clock. Sci (1979). 2011;333(6051):1881–1885. doi: 10.1126/science.1206022
- Lopez-Bigas N, Kisiel TA, DeWaal DC, et al. Genome-wide analysis of the H3K4 histone demethylase RBP2 reveals a transcriptional program controlling differentiation. Mol Cell. 2008 Aug;31(4):520–530. doi: 10.1016/J.MOLCEL.2008.08.004
- Pasini D, Hansen KH, Christensen J, et al. Coordinated regulation of transcriptional repression by the RBP2 H3K4 demethylase and polycomb-repressive complex 2. Genes Dev. 2008 May;22(10):1345–1355. doi: 10.1101/gad.470008
- Xu S, Wang S, Xing S, et al. KDM5A suppresses PML-RARα target gene expression and APL differentiation through repressing H3K4me2. Blood Adv. 2021 Sep;5(17):3241–3253. doi: 10.1182/BLOODADVANCES.2020002819
- Bonnici J, Oueini R, Salah E, et al. The catalytic domains of all human KDM5 JmjC demethylases catalyse N-methyl arginine demethylation. FEBS Lett. 2023;597(7):933–946. doi: 10.1002/1873-3468.14586
- Ohguchi H, Park PMC, Wang T, et al. Lysine demethylase 5A is required for MYC driven transcription in multiple myeloma. Blood Cancer Discov. 2021 Jul;2(4):370–387. doi: 10.1158/2643-3230.BCD-20-0108
- Brier ASB, Loft A, Madsen J, et al. The KDM5 family is required for activation of pro-proliferative cell cycle genes during adipocyte differentiation. Nucleic Acids Res. 2017 Feb;45(4):1743–1759. doi: 10.1093/NAR/GKW1156
- Yoo J, Kim GW, Jeon YH, et al. Drawing a line between histone demethylase KDM5A and KDM5B: their roles in development and tumorigenesis. vol. 54, no. 12 Exp Mol Med. 2022;54(12):2107–2117. doi: 10.1038/s12276-022-00902-0
- van Oevelen C, Wang J, Asp P, et al. A role for mammalian Sin3 in permanent gene silencing. Mol Cell. 2008;32(3):359–370. doi: 10.1016/j.molcel.2008.10.015
- Kim DK, Jeong H, Bae J, et al. Aβ-induced mitochondrial dysfunction in neural progenitors controls KDM5A to influence neuronal differentiation. Exp Mol Med. 2022 Sep;54(9):1461–1471. doi: 10.1038/S12276-022-00841-W
- Zargar ZU, Kimidi MR, Tyagi S. Dynamic site-specific recruitment of RBP2 by pocket protein p130 modulates H3K4 methylation on E2F-responsive promoters. Nucleic Acids Res. 2018 Jan;46(1):174–188. doi: 10.1093/nar/gkx961
- Craig JM, Earle E, Canham P, et al. Analysis of mammalian proteins involved in chromatin modification reveals new metaphase centromeric proteins and distinct chromosomal distribution patterns. Hum Mol Genet. 2003 Dec;12(23):3109–3121. doi: 10.1093/HMG/DDG330
- Prasanth SG, Prasanth KV, Siddiqui K, et al. Human Orc2 localizes to centrosomes, centromeres and heterochromatin during chromosome inheritance. EMBO J. 2004 Jul;23(13):2651–2663. doi: 10.1038/SJ.EMBOJ.7600255
- Huang C, Cheng J, Bawa-Khalfe T, et al. Sumoylated ORC2 recruits a histone demethylase to regulate centromeric histone modification and genomic stability. Cell Rep. 2016 Apr;15(1):147–157. doi: 10.1016/j.celrep.2016.02.091
- Wang WP, Tzeng T-Y, Wang J-Y, et al. The EP300, KDM5A, KDM6A and KDM6B chromatin regulators cooperate with KLF4 in the transcriptional activation of POU5F1. PLoS One. 2012 Dec;7(12):e52556. doi: 10.1371/journal.pone.0052556
- Kong SY, Kim W, Lee HR, et al. The histone demethylase KDM5A is required for the repression of astrocytogenesis and regulated by the translational machinery in neural progenitor cells. FASEB J. 2018 Feb;32(2):1108–1119. doi: 10.1096/FJ.201700780R
- Bennett CN, Ross SE, Longo KA, et al. Regulation of Wnt signaling during adipogenesis. J Biol Chem. 2002 Aug;277(34):30998–31004. doi: 10.1074/JBC.M204527200
- Guo L, Guo YY, Li BY, et al. Histone demethylase KDM5A is transactivated by the transcription factor C/EBPβ and promotes preadipocyte differentiation by inhibiting Wnt/β-catenin signaling. J Biol Chem. 2019 Jun;294(24):9642–9654. doi: 10.1074/JBC.RA119.008419
- Hou J, et al. Genomic amplification and a role in drug-resistance for the KDM5A histone demethylase in breast cancer. Am J Transl Res. 2012;4(3):247. [Online]. Available:/pmc/articles/PMC3426386/ Accessed 12, 10, 2022
- Paolicchi E, Crea F, Farrar WL, et al. Histone lysine demethylases in breast cancer. Crit Rev Oncol Hematol. 2013 May;86(2):97–103. doi: 10.1016/J.CRITREVONC.2012.11.008
- Zeng J, Ge Z, Wang L, et al. The histone demethylase RBP2 is overexpressed in gastric cancer and its inhibition triggers senescence of cancer cells. Gastroenterology. 2010 Mar;138(3):981–992. doi: 10.1053/J.GASTRO.2009.10.004/ATTACHMENT/B76E5BE1-8DAA-4B32-B783-B2B185C95EA3/MMC1.PDF
- Li S, Wu Z, Li Q, et al. The prognostic value of AT-Rich interaction domain (ARID) family members in patients with hepatocellular carcinoma. Evid Based Complement Alternat Med. 2022;2022:1–16. doi: 10.1155/2022/1150390
- Yang GJ, Ko CN, Zhong HJ, et al. Structure-based discovery of a selective KDM5A inhibitor that exhibits anti-cancer activity via inducing cell cycle arrest and senescence in breast cancer cell lines. Cancers (Basel). 2019 Jan;11(1). doi: 10.3390/CANCERS11010092.
- Feng T, Wang Y, Lang Y, et al. KDM5A promotes proliferation and EMT in ovarian cancer and closely correlates with PTX resistance. Mol Med Rep. 2017 Sep;16(3):3573–3580. doi: 10.3892/mmr.2017.6960
- Hu D, Jablonowski C, Cheng P-H, et al. KDM5A regulates a translational Program that controls p53 protein expression. iScience. 2018;9:84–100. doi: 10.1016/j.isci.2018.10.012
- De Rooij JDE, Hollink IHIM, Arentsen-Peters STCJM, et al. NUP98/JARID1A is a novel recurrent abnormality in pediatric acute megakaryoblastic leukemia with a distinct HOX gene expression pattern. Leukemia. 2013;27(12):2280–2288. doi: 10.1038/leu.2013.87
- Gough SM, Slape CI, Aplan PD. NUP98 gene fusions and hematopoietic malignancies: common themes and new biologic insights. Blood. 2011 Dec;118(24):6247–6257. doi: 10.1182/BLOOD-2011-07-328880
- Pineault N, Buske C, Feuring-Buske M, et al. Induction of acute myeloid leukemia in mice by the human leukemia-specific fusion gene NUP98-HOXD13 in concert with Meis1. Blood. 2003 Jun;101(11):4529–4538. doi: 10.1182/BLOOD-2002-08-2484
- Wang GG, Cai L, Pasillas MP, et al. NUP98-NSD1 links H3K36 methylation to hox-A gene activation and leukaemogenesis. Nat Cell Biol. 2007 Jul;9(7):804–812. doi: 10.1038/NCB1608
- Liang X, Zeng J, Wang L, et al. Histone demethylase retinoblastoma binding protein 2 is overexpressed in hepatocellular carcinoma and negatively regulated by hsa-miR-212. PLoS One. 2013 Jul;8(7):e69784. doi: 10.1371/JOURNAL.PONE.0069784
- Liu C, Zheng Z, Li W, et al. Inhibition of KDM5A attenuates cisplatin-induced hearing loss via regulation of the MAPK/AKT pathway. Cell Mol Life Sci. 2022 Dec;79(12). doi: 10.1007/S00018-022-04565-Y
- Peng Y, Suryadi J, Yang Y, et al. Mutations in the KDM5C ARID domain and their plausible association with Syndromic Claes-Jensen-type disease. Int J Mol Sci. 2015 Nov;16(11):27270. doi: 10.3390/IJMS161126022
- Ugur FS, Kelly MJS, Galonic´fujimori D, et al. Chromatin sensing by the Auxiliary domains of KDM5C regulates its demethylase activity and is disrupted by X-linked intellectual disability mutations. J Mol Biol. 2023;435(2):167913. doi: 10.1016/j.jmb.2022.167913
- Miller J, McLachlan AD, Klug A. Repetitive zinc-binding domains in the protein transcription factor IIIA from xenopus oocytes. EMBO J. 1985;4(6):1609–1614. doi: 10.1002/J.1460-2075.1985.TB03825.X
- Hanas JS, Hazuda DJ, Bogenhagen DF, et al. Xenopus transcription RNA gene* factor a requires Zinc for binding to the 5 s. J Biol Chem. 1983;258(23):14120–14125. doi:10.1016/S0021-9258(17)43831-2
- Zhang Y, Guo Y, Gough SM, et al. Mechanistic insights into chromatin targeting by leukemic NUP98-PHF23 fusion. Nat Commun. 2020 Dec;11(1). doi: 10.1038/S41467-020-17098-4
- Liu X, Secombe J. The histone demethylase KDM5 activates gene expression by recognizing chromatin context through its PHD reader motif. Cell Rep. 2015;13(10):2219–2231. doi: 10.1016/J.CELREP.2015.11.007
- Longbotham JE, Chio CM, Dharmarajan V, et al. Histone H3 binding to the PHD1 domain of histone demethylase KDM5A enables active site remodeling. Nat Commun. 2019 Dec;10(1). doi: 10.1038/s41467-018-07829-z
- Suganuma T, Workman JL. Crosstalk among histone modifications. Cell. 2008 Nov;135(4):604–607. doi: 10.1016/j.cell.2008.10.036
- Hayakawa T, Ohtani Y, Hayakawa N, et al. RBP2 is an MRG15 complex component and down-regulates intragenic histone H3 lysine 4 methylation. Genes Cells. 2007 Jun: 070606122915002–???. doi: 10.1111/j.1365-2443.2007.01089.x
- Varier RA, de Santa Pau EC, van der Groep P, et al. Recruitment of the mammalian histone-modifying EMSY complex to target genes is regulated by ZNF131. J Biol Chem. 2016 Apr;291(14):7313–7324. doi: 10.1074/jbc.M115.701227
- Chen PL, Scully P, Shew JY, et al. Phosphorylation of the retinoblastoma gene product is modulated during the cell cycle and cellular differentiation. Cell. 1989 Sep;58(6):1193–1198. doi: 10.1016/0092-8674(89)90517-5
- Giacinti C, Giordano A. RB and cell cycle progression. Oncogene. Aug. 28, 2006;25(38):5220–5227. doi: 10.1038/sj.onc.1209615
- Roesch A, Becker B, Meyer S, et al. Retinoblastoma-binding protein 2-homolog 1: a retinoblastoma-binding protein downregulated in malignant melanomas. Mod Pathol. 2005 Sep;18(9):1249–1257. doi: 10.1038/MODPATHOL.3800413
- Lin W, Cao J, Liu J, et al. Loss of the retinoblastoma binding protein 2 (RBP2) histone demethylase suppresses tumorigenesis in mice lacking Rb1 or Men1. Proc Natl Acad Sci U S A. 2011 Aug;108(33):13379–13386. doi: 10.1073/PNAS.1110104108
- Canhoto AJ, Chestukhin A, Litovchick L, et al. Phosphorylation of the retinoblastoma-related protein p130 in growth-arrested cells. Oncogene. 2000;19(44):5116–5122. vol. 19, no. 44. doi: 10.1038/sj.onc.1203893.
- Mayol X, Garriga J, Grana X. Cell cycle-dependent phosphorylation of the retinoblastoma-related protein p130. Oncogene. 1995 Aug [Accessed 16, Nov 2023];11(4):801–808. [Online]. Available: https://europepmc.org/article/med/7651744
- Dephoure N, Zhou C, Villén J, et al. A quantitative atlas of mitotic phosphorylation. Proc Natl Acad Sci U S A. 2008 Aug;105(31):10762. doi: 10.1073/PNAS.0805139105
- Cole MD, Henriksson M. 25 years of the c-Myc oncogene. Semin Cancer Biol. 2006 Aug;16(4):241. doi: 10.1016/J.SEMCANCER.2006.08.003
- McMahon SB, Van Buskirk HA, Dugan KA, et al. The novel ATM-related protein TRRAP is an essential cofactor for the c-Myc and E2F oncoproteins. Cell. 1998 Aug;94(3):363–374. doi: 10.1016/S0092-8674(00)81479-8
- Nikiforov MA, Chandriani S, Park J, et al. TRRAP-Dependent and TRRAP-Independent transcriptional activation by Myc family oncoproteins. Mol Cell Biol. 2002 Jul;22(14):5054. doi: 10.1128/MCB.22.14.5054-5063.2002
- Ayer DE, Lawrence QA, Eisenman RN. Mad-max transcriptional repression is mediated by ternary complex formation with mammalian homologs of yeast repressor Sin3. Cell. 1995 Mar;80(5):767–776. doi: 10.1016/0092-8674(95)90355-0
- Secombe J, Eisenman RN. The function and regulation of the JARID1 family of histone H3 lysine 4 demethylases: the Myc connection. Cell Cycle. 2007 Jun;6(11):1324–1328. doi: 10.4161/CC.6.11.4269
- Secombe J, Li L, Carlos L, et al. The Trithorax group protein Lid is a trimethyl histone H3K4 demethylase required for dMyc-induced cell growth. Genes Dev. 2007 Mar;21(5):537. doi: 10.1101/GAD.1523007
- Nishibuchi G, Shibata Y, Hayakawa T, et al. Physical and functional interactions between the histone H3K4 demethylase KDM5A and the nucleosome remodeling and deacetylase (NuRD) complex. J Biol Chem. 2014 Oct;289(42):28956–28970. doi: 10.1074/JBC.M114.573725
- Malovannaya A, Lanz R, Jung S, et al. Analysis of the human endogenous coregulator complexome. Cell. 2011 May;145(5):787–799. doi: 10.1016/J.CELL.2011.05.006
- Gaillard S, Charasson V, Ribeyre C, et al. Kdm5a and kdm5b histone-demethylases contribute to hu-induced replication stress response and tolerance. Biol Open. 2021 May;10(5). doi: 10.1242/BIO.057729/268370
- Zhao D, et al., ‘Erratum: H3K4me3 demethylase Kdm5a is required for NK cell activation by associating with p50 to suppress SOCS1 (cell reports (2016) 15(2) (288–299), ( S2211124716302911), (10.1016/j.celrep.2016.03.035), Cell Reports, vol. 30(7) Elsevier B.V, p. 2460, Feb. 18, 2020. doi: 10.1016/j.celrep.2020.01.104.
- Manna S, Kumar Patra S, Samir Kumar Patra P. Physical association of functionally antagonistic enzymes: kDM5A interacts with MLLs to regulate gene expression in a promoter specific manner facilitating EMT and pluripotency. DOI:10.1101/2021.12.03.471073.
- Yu X, Chen H, Zuo C, et al. Chromatin remodeling: demethylating H3K4me3 of type I IFNs gene by Rbp2 through interacting with Piasy for transcriptional attenuation. FASEB J. 2018;32(2):552–567. doi: 10.1096/fj.201700088RR
- Chan SW, Hong W. Retinoblastoma-binding protein 2 (Rbp2) potentiates nuclear hormone receptor-mediated transcription *. J Biol Chem. 2001 Jul;276(30):28402–28412. M100313200. doi: 10.1074/jbc.M100313200