ABSTRACT
Wounding induces systemic potentials in Arabidopsis thaliana that can be abolished by concomitant suppression of the GLUTAMATE RECEPTOR-LIKE GLR3.3 and GLR3.6 genes. However, the roles of specific GLR channels to these potentials remain unclear. Here I applied the Electrical Penetration Graph (EPG) to study the contribution of three GLR channels to wound-induced, systemically propagated electrical potentials in Arabidopsis thaliana. In contrast to recordings made with conventional rigs for whole-plant electrophysiology, the EPG allows for the unambiguous distinction of the phloem-propagated action potential (AP) from the electrical activity outside of the phloem. The data reported here suggest that: (a) the transmission of wound-induced, phloem-propagated AP to neighbor leaves, requires expression of GLR3.3 or GLR3.6, whereas GLR3.5 prevents its transmission to non-neighbor leaves; (b) the generation of wound-induced electrical signals outside the phloem network depends on GLR3.6 expression; and (c) wound-induced systemic potentials initiated in the shoot are transmitted to the root in the adult plant, which suggests a role for these electrical signals in coordinating the plant defenses in the shoot and in the root. Here, I propose a model for wound-induced systemic electrical signals at the molecular, cellular and anatomical level. In this model, GLR3.3 and GLR3.6 function as on switches for the propagation of wound-induced potentials beyond the wounded leaf, while GLR3.5 functions as an off switch that prevents the propagation of wound-induced electrical potentials to distal, non-neighbor leaves.
Abbreviations
CC | = | Companion cell |
EPG | = | Electrical Penetration Graph |
GLR | = | Glutamate receptor-like channel |
SE | = | Sieve element |
Introduction
Plants have evolved sophisticated biochemical, genetic and physiological adaptations to environmental stresses. Insect herbivory is a major threat in a plant's life. Insect-made wounds are potentially lethal, because they represent points through which a plant quickly loses water pressure,Citation1 and through which infectious organisms may enter.Citation2 The removal of plant tissue in this manner is also detrimental to plant health. Recently it has been shown that small wounds induce the expression of the jasmonate-dependent defense genes in unwounded regions, in a manner that depends on the propagation of electrical signals from the wounded area.Citation3 Likely, systemically propagated electrical signals in plants were selected for during evolution because they significantly increased the plant's likelihood of survival and reproduction in the context of herbivory. Therefore, unveiling the genetic underpinnings for the systemic electrical transmission in plants is essential to understanding the plant-insect co-evolution.
Electrical signals are generated by a variety of voltage and ligand-dependent ion channels and transporters. These are complex multi-subunit proteins, expressed at the plasma membrane, which harbor multiple motifs that determine their ion selectivity, conduction properties, and modulation by second messengers.Citation4 The genome of Arabidopsis thaliana contains over 70 genes for ion channels and transporters.Citation5 Twenty of these genes belong to the glutamate receptor-like (GLR) family of ion channels, which is related to the ionotropic glutamate receptor (iGluR) gene family in animalsCitation6 that mediate most excitatory transmission in the brain.Citation7 In the animal nervous system, GLR channels assemble as tetramers that contain two types of subunits, 2 that bind glutamate and 2 that bind glycine.Citation8 The subunit composition of native plant GLRs remains elusive, although it is known that single cells express 4 to 6 different GLR subunits.Citation9 Interestingly, Taniura et al.Citation10 (2006) have noted that the plant GLR3.5 channel is the only of the 20 plant GLRs whose N-terminal LIVBP domain contains the same 5 key amino acid residues that in mammalian iGluRs are required for binding to the α-carboxylic and α-amino groups of glutamate.Citation11
A survey of T-DNA Arabidopsis mutants with surface electrodes identified the GLR3.3 and the GLR3.6 channels as key mediators of wound-induced potentials propagated between leaves.Citation3 Another study that used an alternative rig for whole-plant electrophysiology, namely the Electrical Penetration Graph (EPG), showed that the phloem of the glr3.3 glr3.6 double mutant does not propagate wound induced electrical signals beyond the wounded leaf.Citation12 The consistency between these two studiesCitation3,12 suggests that the wound-induced potentials measured with conventional surface electrodes and the potentials measured with the EPG share cellular and molecular origins. The recordings made with coarse surface electrodes are low signal-to-noise ratio recordings in which the different electrical signals are difficult or impossible to distinguish from each other. Surface electrode recordings of single GLR mutants showed that suppression of only GLR3.3 or GLR3.6 does not abolish the propagation of wound-induced electrical signals between neighbor leaves, although these signals are markedly shorter than in the wild type, in both mutants.Citation3 However, these data did not clarify the contribution of each these channels to specific signals. For instance, the shortening of the electrical signal could be due to elimination of the function of an entire population of contributing cells, or to a modification of the biophysics of the ion currents that underlie these potentials, or to both. The EPG, in contrast, yields high signal-to-noise ratio recordings of the phloem-transmitted AP; however, single GLR mutants have not yet been examined with the EPG technique. Here I investigated the individual contributions of GLR3.3. GLR3.5 and GLR3.6 to the different wound-induced electrical signals with the EPG technique, as illustrated in . The results of these experiments suggest that GLR3.3 or GLR3.6 are required to send the phloem-propagated AP beyond the wounded leaf, that GLR3.6 is necessary for the occurrence of the electrical potential outside of the phloem, and that GLR3.5 prevents the entry of the phloem-propagated AP into leaves that are not neighbor to the wounded ones. In summary, this study suggests that GLR channels switch on and off the propagation of the phloem AP to unwounded leaves, and perhaps contribute electrical currents underlying wound-induced electrical signals outside of the phloem network.
Figure 1. The Electrical Penetration Graph (EPG) re-purposed for whole-plant electrophysiology. (A) Diagram of the general configuration of the EPG rig, which records potential differences between a coarse copper electrode placed within the root medium, and a SE/CC electrode, represented by the stylet bundle of an aphid or other phloem-feeding insect. The insect is integrated into the EPG via an ultra-thin (ø = 18 µm) gold wire immersed into a dried up droplet of silver glue on the aphid's abdomen. Both electrodes feed into a differential amplifier with an inverting input and a non-inverting input. The input resistance (Ri = 1 GΩ) lies between the ground electrode and the point of measurement. (B) Electron micrograph of a transversal section of the stylet bundle of Nasonovia ribisnigri in between cells of Lactuca sativa. Abbreviations used are: fc = food canal, sc = salivary canal, mx = maxillar stylet, md = mandibular stylet, nc = neural canal, ss = salivary sheath. Image courtesy of Freddy Tjallingii.
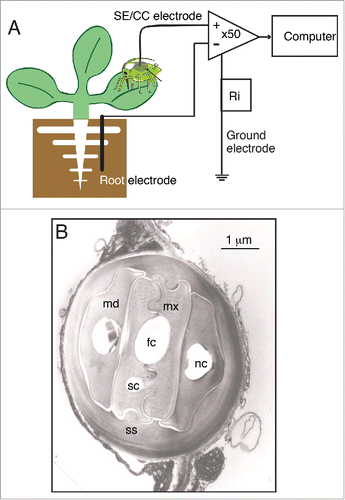
Results
Wounding induces long and transient potentials between the root and the SE/CC complexes in unwounded leaves
Cutting a leaf at the petiole/lamina junction induced long, sustained potentials, as well as short potentials referred to as action potentials (APs), between the root and SE/CCs in unwounded neighbor leaves (). When the EPG-recorded aphid (i.e. the SE/CC electrode) was placed on a non-neighbor leaf, only the long potential was recorded (). The leaf pairs 5-8 and 5-3 were used to test electrical transmission between neighbor leaves, and the pair 8-9 was used for examining electrical transmission between non-neighbor leaves (Fig. S1). The long potential rises slowly, reaching peak amplitude within 15s, and has variable durations between 1 and 3 minutes. In contrast, the AP rises quickly, reaching peak amplitude within a second, and appears invariably superimposed over the long potential in the EPG recordings (). The average amplitudes of the AP and the long potential were c. 75 mV and c. 37 mV respectively (). The average duration of the entire wound-induced potential was c. 150s if the SE/CC electrode was in a neighbor leaf (), and c. 130s if the SE/CC electrode was in a non-neighbor leaf (). Note that in the latter case there is no contribution of the AP to the total duration of the electrical signal. These results are consistent with a previous study.Citation12
Figure 2. Wound-induced systemic potentials between the root and SE/CCs in neighbor leaves of Arabidopsis thaliana wild type and GLR mutants. Typical wound-induced potentials of a wild type plant (A), as well as of the single mutants glr3.3a (B), glr3.6b (C), and glr3.5a (D) are shown. The inset illustrates the design for these experiments. In all cases except in the glr3.6 mutant, the recordings show unambiguously 2 potentials, a long potential and a superimposed action potential, annotated as LP and AP in (A). The time of wounding is indicated with arrows.
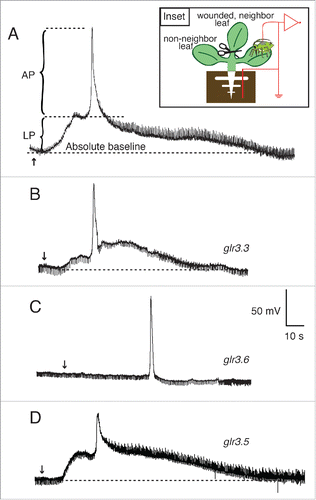
Figure 3. Amplitudes and durations of wound-induced systemic potentials between the root and SE/CCs in neighbor leaves of Arabidopsis thaliana wild type and single GLR mutants. (A) Average maximal (peak) amplitudes of action potentials and long potentials produced by wild type (WT), glr3.3, glr3.6, and glr3.5 plants, recorded by EPG in the configuration shown in the inset of . (B) Average durations of the total systemic potentials in wild type (WT), and in each of the mutant plants. Bars represent mean ± s.e.m. The number of plants is indicated in parentheses. Statistical differences between the mean amplitudes and durations of the potentials in wild type and each of the mutants were assessed with Student's t tests.
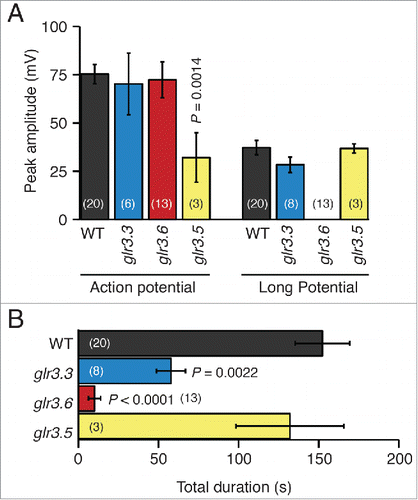
Figure 4. Wound-induced systemic potentials between the root and SE/CCs in non-neighbor leaves of Arabidopsis thaliana wild type and single GLR mutants. Typical wound- induced potentials of a wild type plant (A), as well as of the single mutants glr3.3a (B), glr3.6b (C), and glr3.5-a (D), recorded by EPG in the configuration illustrated in the inset are shown. Panel C shows a long, continuous EPG recording from a glr3.6b mutant that is broken into 3 segments. Arrows indicate the time of wounding. The results from both T-DNA alleles of GLR3.5 were similar. Results from glr3.5-b are shown in Fig. S2. The time of wounding is indicated with arrows.
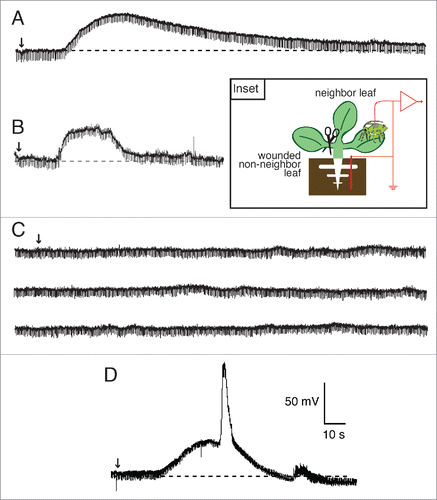
Figure 5. Amplitudes and durations of wound-induced systemic potentials between the root and SE/CCs in non-neighbor leaves of Arabidopsis thaliana wild type and single GLR mutants. (A) Average maximal (peak) amplitudes of transient potentials and long potentials produced by wild type (WT), and glr3.3, glr3.6, and glr3.5 single mutant plants, recorded with the EPG in the configuration shown in the inset of . (B) Average durations of the total systemic potentials in wild type (WT) and each of the mutant plants. Bars represent mean ± s.e.m. The number of plants is indicated in parentheses. Statistical differences between the mean amplitudes and durations of the potentials in wild type and each of the mutants were assessed with Student's t tests.
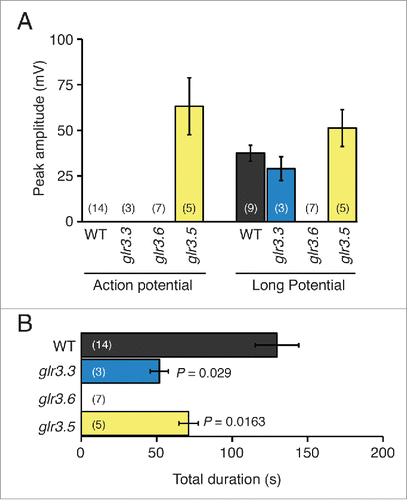
Suppressing GLR3.3 expression shortens the duration of the wound-induced long potential between the root and the SE/CC complex in unwounded leaves
The general waveforms of wound-induced potentials between the root and the SE/CC in unwounded leaves of glr3.3 and wild type plants were similar (), and the amplitudes of the AP and the long potential in both genotypes were not significantly different (). However, the average total duration of the wound-induced potentials in glr3.3 plants was c. 60% shorter than that of the wild type (). These results are consistent with the previous study that showed a significant reduction in the duration of potentials transmitted between neighbor leaves in the glr3.3 mutant with respect to the wild type.Citation3 The new EPG data here additionally shows that the glr3.3 mutation affects the duration of the long potential, but not that of the AP.
Suppressing GLR3.6 expression abolishes the wound-induced long potentials between the root and the SE/CC complex in unwounded leaves
In contrast to wild type plants, which invariably produce long potentials between the root and SE/CCs in unwounded leaves, the glr3.6 mutant appeared to be unable to produce these long potentials in response to wounding, although it still propagated APs between neighbor leaves (). Here it is important to note that, in the EPG recordings, the remotely induced AP in the glr3.6 mutant rises from the absolute baseline, whereas in the wild type, it rises from the depolarized steady-state of the long potential (compare ). Interestingly, the amplitudes of the remotely induced APs in wt and glr3.6 plants were not significantly different (). In other words, the occurrence of the long potential did not affect the maximum level reached by the AP that is apparently superimposed on it. Consistently, the surface potentials transmitted between neighbor leaves in the glr3.6 mutant were much shorter than those in wild type plants, as if a large component of the signal had been eliminated.Citation3 The new EPG data shown here demonstrates that the dramatic shortening in the wound-induced systemic potential in the glr3.6 mutant results from the suppression of the long potential.
Suppressing GLR3.5 expression creates wound-induced APs between the root and SS/CC complexes in unwounded non-neighbor leaves
Next, I examined the wound-induced systemic potentials in glr3.5 plants. The motivation for examining the contribution of GLR3.5 was that this is the only GLR channel in Arabidopsis with a complete binding site for glutamate, which may confer an important role in the wound-induced systemic electrical signal.Citation11 The data show that the potentials between the root and the SE/CCs in neighbor leaves were similar in waveform to those of the wild type (), although the amplitude of the AP in this mutant was markedly shorter than in the wild type (). In addition, the duration of the entire wound-induced systemic potential in glr3.5 was slightly reduced with respect to the wild type, but not significantly so (). However, the most surprising phenotype of the glr3.5 mutant was that it generated wound-induced APs in SE/CCs of non-neighbor leaves in all plants tested (). This is an all-or-nothing, gain-of-function phenotype, because none of the wild type plants examined in this study or in the previous studyCitation12 transmitted wound-induced APs between non-neighbor leaves. Additionally, this new AP in the glr3.5 mutant was doubled in size compared to the AP transmitted between neighboring leaves of the same mutant, and of similar size as the AP transmitted between neighboring leaves in wild type plants (compare ). A comparison of these data with those in the previous study using surface electrodesCitation3 is not possible, because in that study wound-induced electrical signals between non-neighbor leaves were not reported for any single GLR mutant, including GLR3.5.
Concomitant suppression of GLR3.3 and GLR3.6 expression abolishes the wound-induced AP in unwounded leaves but not in wounded leaves
shows the absence of wound-induced potentials between the root and a SE/CC in an unwounded neighbor leaf of the double mutant glr3.3 glr3.6, corroborating the results from the previous study.Citation12 However, the same double mutant consistently generated and propagated wound-induced APs along the SE/CC network of the wounded leaf (). Average amplitudes of the locally propagated AP were 77 ± 12 mV (n = 6) for wild type, and 85 ± 14 mV (n = 4) for the double mutant glr3.3 glr3.6. The average durations of these potentials were: 3 ± 0.5s for the wild type, and 4 ± 0.5s for glr3.3 glr3.6. Thus, the double glr3.3 glr3.6 mutation does not affect the generation and propagation of the wound-induced AP in the wounded leaf. This result is consistent to the previous study with surface electrodes, which shows the transmission of the wound-induced signal within the wounded leaf in the double glr3.3 glr3.6 mutant.Citation3
Figure 6. Wound-induced systemic potentials between the root and SE/CCs wounded and unwounded leaves of Arabidopsis thaliana wild type and glr3.3 glr3.6 mutant. (A) EPG recording of the potential between the soil electrode and a SE/CC electrode in an unwounded neighbor leaf in a glr3.3 glr3.6 mutant. Small wounds produced with tweezers in the middle of the leaf, on the midvein (B), induced transient potentials that propagated basipetally in the wounded leaf via the SE/CC network, both in the wild type (C) and in the double glr3.3 glr3.6 mutant (D). Arrows indicate the moment a leaf was cut (A), or wounded with tweezers (C,D).
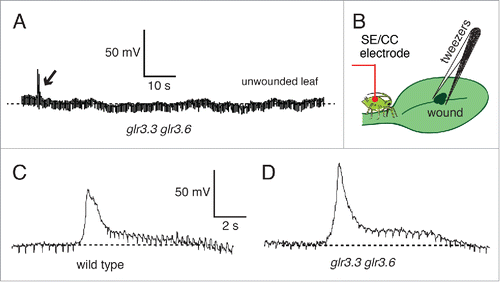
Discussion
Alternative mechanisms for electrical transmission in multicellular organisms
Until recently, long-distance electrical transmission in plants was generally regarded as a simplified version of long-distance electrical transmission in animals. However, if multicellularity evolved independently in plants and animals,Citation13 then the mechanisms for propagating electrical signals in these two groups evolved independently as well. It is thus fundamentally incorrect to regard one system for electrical transmission as a simplified or imperfect version of the other, given that they fulfill completely different requirements. The results presented here highlight the different utilization of a common, primitive building block of excitability by plants and animals. The GLR gene is ancient, since phylogenetic analyses suggest that it was present in the common unicellular ancestor of plants and animals.Citation14 Animals express GLR channel genes at the synaptic junction, where they generate ionic currents that directly mediate the transmission of electrical signals between neurons.Citation7 In contrast, the data provided here, together with previous experimental studiesCitation3,12 suggest that in plants, GLR channels play a key role in determining the electrical connections between the phloem vasculature of leaves. Therefore, it appears that plants and animals have deconstructed the GLR gene from its original unicellular context to re-frame it in the context of multicellularity in completely different ways.
Re-interpretation of the EPG data on whole-plant electrophysiology
The waveform of the wound-induced systemic potentials acquired with the EPG is peculiar, as it contains an AP apparently superimposed onto the steady state of a low amplitude, long-lasting depolarization (). In the previous study describing the use of the EPG as a method for plant electrophysiology,Citation12 both the AP and the long potential were assumed to originate from ionic currents across the SE/CC complex probed by the stylet of the EPG-recorded aphid. Here I contend that this notion is incorrect. Whereas the high resolution with which the AP is recorded by the EPG is consistent with its being produced by a single SE/CC complex, it seems unlikely that the same SE/CC complex generates the long potential as well. Prolonged depolarizations are detrimental to cell health, and are a sign of defective repolarizing K+ channels, as in the case of the long QT2 syndrome.Citation15 One could argue that the membrane potential of the SE/CC complex is forced into a depolarized state by an ephaptic effect,Citation16 in which ionic currents flowing through cells – in this case cells surrounding the SE/CCs – affect the gating of ion channels in nearby cells, in this case the SE/CC. If the long potential origin were the SE/CC complex, regardless of whether it is primary excitation or secondary (ephaptic) excitation, this first long depolarization would diminish the electrical driving force for the ion currents underlying the AP. However, the new data on the glr3.6 mutant presented here, provides evidence against this hypothesis. In the glr3.6 mutant, the remotely induced AP, which occurs in the absence of the long potential, has similar amplitude to that of the remotely induced AP in the wild type, which appears on top of the long potential (compare ). Therefore, it is unlikely that the long potential is generated in the SE/CC complex or in its vicinity.
Where is then the long potential generated? The two major possibilities are: within the recording horizon of the intracellular SE/CC electrode (i.e. the aphid's stylet), or within the recording horizon of the root electrode. An electrode's recording horizon is positively correlated with its tip diameter; thin titanium nitride electrodes (Ø ∼10 µm) used in multi-electrode arrays (MEA) capture signals within a radius within 30 µm around the electrode.Citation17 Extrapolating the recording horizons for the MEA electrodes to the special EPG intracellular bio-electrode is not straightforward. Nevertheless, since the inner Ø of the food and salivary canals are, respectively, 1 and 0.5 µm (), it is reasonable to assume that the radius of the stylet's recording horizon is only a few µm in magnitude, and it does not significantly extend beyond the SE/CC complex into which it is inserted. Therefore, the most likely origin of the long potential is within the recording horizon of the large EPG soil electrode (Ø ∼1.5 mm). The roots of 4-5 week old A. thaliana plants were profusely ramified through the small soil pots used here. The variations in root architecture and exact position of the root electrode between experiments likely account for the variability in the waveform and the duration of the long potentials. illustrates the proposed contributions of the SE/CC complex and the root to the different phases of a typical EPG recording. Although EPG can only record the long potentials originated in the root area, it is likely that these are also produced in leaves (see next section), but outside of the recording horizon of the SE/CC electrode. The plant root contains excitable tissue that expresses a wide variety of voltage- and ligand-gated ion channels that support the generation of electrical potentials.Citation9,18,19. Interestingly, spontaneous small depolarizations propagating over short distances in the root apical meristem have been recorded with micro-electrode arrays.Citation20,21 This spontaneous electrical activity in the root could underlie spontaneous, more or less regular fluctuations of the EPG baseline during the stable feeding phase (E2 waveform) that are occasionally observed by myself and other EPG users. Because of this evidence indicating that the root is an electrically excitable area, caution should be exerted when interpreting data acquired by whole-plant electrophysiology rigs that, like the EPG, use a fixed soil/root electrode. For instance, if increasing the distance between the point of stimulus and the recording site is not accompanied by an increased latency of the electrophysiological response, it may not be because an instantaneous hydraulic pressure wave causes the electrophysiological responseCitation1, but because the response originates in the root, recorded by an electrode that has remained in the same position.
Figure 7. Proposed contributions of the root and the SE/CC network to the wound-induced action potential and long potential. (A) Drawn waveform of a typical wound-induced EPG-recorded systemic potential. The potential difference (PD) between the root and the SE/CC electrode is plotted as a function of time. In a typical EPG-recorded systemic signal there are 3 potential levels. (B) At rest (PD0) only the resting membrane potential of the SE/CC contributes to the PD. By convention, the extracellular or external resting potential (in this case external to the root) is regarded as negligible (Vs→0), although there are always background ionic currents. In the given example, the intracellular potential of the SE/CC has an arbitrary value of −100 mV. (C) The first potential level of a typical EPG-recorded signal (PD1) is generated by electrical activity in the root. At this point, the SE/CC is still unexcited. Intracellular depolarizations in root cells are recorded as a compound extracellular hyperpolarization by the root electrode. The size of the recorded extracellular hyperpolarization will be reduced proportionally with the distance between the root and the electrode. In the given example, the excitation (depolarization) of a root area is recorded externally as a +30 mV hyperpolarization (these are arbitrary values). Since the EPG uses a differential amplifier with an inverting input and a non-inverting input, the polarity of the root potential is reversed before calculating the PD. (D) The second potential level of a typical EPG-recorded signal (PD2) is generated by the still ongoing electrical activity in the root and a maximally excited SE/CC. In the given example, the maximal depolarization in the SE/CC is −50 mV. Abbreviations used are: SE = sieve element; CC = companion cell, VSE = membrane potential of the sieve element; VS = extracellular potential of the root, in the vicinity of the soil electrode. The excited depolarized membrane potential is shown with an asterisk (e.g. V*SE).
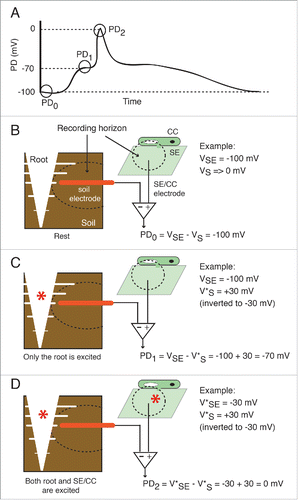
A new model of the molecular, cellular and anatomical substrates for plant electrical transmission
The new data acquired with the EPG technique, and interpreted as explained above and illustrated in supports the following conclusions:
The long potential that is generated outside of the SE/CC complex depends on GLR3.6 expression. However, the precise mode in which GLR3.6 contributes to the long potential is not clear yet. GLR3.6 mediates inward Ca2+ currents in the root,Citation22 which could underlie the long potential. How would the GLR3.6 channel become activated? An ephaptic mechanismCitation16 by which the electrical field associated with the SE/CC-propagated AP activates Ca2+ currents in non-phloem cells is not far-fetched since of a new study shows that relatively weak electric fields of only 2–5 mV/mm are sufficient to allow the transverse propagation of action potentials, inducing electrical currents in nearby cells.Citation23
The duration of the long potential, but not of the AP, is significantly affected by GLR3.3 expression. Two explanations are: (i) Ionic currents through GLR3.3 channels affect the duration of the electrical signals in cells other than SE/CCs, and (ii) GLR3.3 channels switch on the wound-induced electrical potentials in one or more, but not all, participating cell populations outside of the SE/CC network.
Expression of GLR3.3 or GLR3.6 is necessary for the exit of the AP beyond the wounded leaf via the SE/CC network. GLR3.3 and GLR3.6 are functionally redundant in this context.
Expression of GLR3.5 blocks the entry of the AP into the SE/CC network of unwounded non-neighbor leaves, preventing the wound-induced electrical signal from being fully systemic. This is one of the most interesting result of this study, because it suggests that the plant actively regulates the spatial distribution of systemic electrical signals. By restricting the electrical signal to the leaves that are closer to the wounded one, where it is more relevant, the plant avoids investing excessive energy in defense. In addition, this result opens the question of whether the spatial regulation of systemic electrical signals in plants is plastic, even subject to epigenetic regulation.
shows a model for the molecular mechanisms governing the spatial distribution of the wound-induced long-distance electrical signal, in which the GLR3.3, GLR3.5, and GLR3.6 channels function as digital switches that allow or block the transmission of the AP between leaves. The precise mechanisms by which these channels determine the 3D spatial distribution of the wound-induced AP remain to be determined. A possibility is that GLR channels are expressed at early stages of development to carry important Ca2+ signals that regulate the formation of electrical bridges and barriers between the vasculatures of neighbor and non-neighbor leaves. The recent findings that GLR3.5 plays an important role in signaling during development,Citation24 and that the phloem-expressed GLR3.2 and GLR3.4 interact with each other to modulate the root architecture,Citation25 are consistent with this hypothesis. If GLR channels do not carry the ionic currents that underlie the phloem propagated AP, which ion channels or transporters are responsible? Future studies with forward genetic approaches will provide unbiased information on the molecular effectors and regulators of this poorly understood phenomenon. Studying electrical transmission in plants with modern tools and techniques is necessary for building an alternative model of electrical transmission in multi-cellular organisms.
Materials and methods
Plants and growth conditions
Wild type Arabidopsis thaliana L. plants, Columbia-0 accession, were used. The T-DNA single mutants glr3.3a (At1g42540, salk_099757) and glr3.6b (At3g51480, salk_035353), as well as the double mutant glr3.3a glr3.36a were donated by Ted Farmer.Citation3 Two T-DNA alleles of the GLR3.5 gene were purchased from the Nottingham Arabidopsis Stock Center: glr3.5-a (At2g32390, salk_035264) and glr3.5-b (At2g32390, salk_111204). All mutant plants are in Columbia-0 background. All plants were soil grown in small plastic soil pots of 6 cm in diameter, growth chambers at 23° C in 70% humidity, with a light intensity of 100 μE m−2 s−1 in a 9:15 h Light: Dark [L: D] photoperiod. Plants between 4 and 5 weeks of age were used in the experiments.
Figure 8. Model for the molecular, cellular, and anatomical bases of the systemically transmitted wound-induced electrical potentials in Arabidopsis thaliana. Wild type A. thaliana plants generate 2 major types of electrical signals upon wounding that can be recorded in unwounded leaves, namely an action potential (AP) in the SE/CC network of the phloem, and a long potential (LP) in unknown cell populations, outside of the SE/CC network. The model proposes that GLR3.3 and GLR3.6 have a redundant function in sending the AP to unwounded leaves via the SE/CC network. GLR3.5 functions as an off-switch that blocks the transmission of the AP to non-neighbor leaves. However, the AP sent to the neighbor leaf in this mutant is significantly small, compared with that of wild type plants (c. 30 mV), and therefore, it may act as a partial on-switch for the transmission of the AP between neighbor leaves. This is not shown in the model, as it emphasizes “all or none” phenotypes. GLR3.6 is essential for the occurrence of the wound-induced long potential. The model proposes that the GLR3.6-dependent LP is not independently propagated between leaves, but induced locally by the phloem-propagated AP. Therefore, the AP represents primary excitation, and the LP represents secondary excitation. This model suggests that the fine coordination of the expression of these GLR channels results in a highly regulated spatial distribution of stimulus-induced electrical signals in plants.
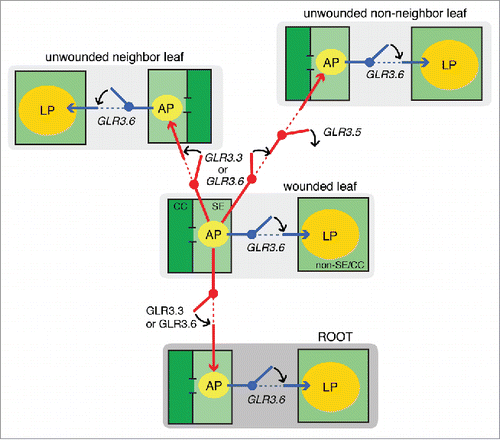
Aphids
Clones of the cabbage aphid Brevicoryne brassicae L. originated from single virginiparous females collected in Villa del Prado, Madrid (Spain). Aphids were greenhouse reared on cabbage, Brassica oleracea L., without supplemental lighting, and within temperature ranges of 20:18° C [L:D].
Genotyping of T-DNA insertion lines
The two T-DNA alleles of the GLR3.5 gene were genotyped to ensure that they were homozygous. For this purpose, small fresh leaf samples were placed into Eppendorf tubes and ground with a Quiagen TissueLyser II (Retsch Technology). Then, 60 μL of extraction buffer (200 mM Tris HCl pH 7.5, 250 mM NaCl, 25 mM Na2EDTA, 0.5% SDS) was added to each tube, and these were centrifuged at 4,000g for 10 min. The supernatants were transferred into new Eppendorf tubes, to which 50 μL isopropanol was added. Tubes were then centrifuged at 4,000 for 5 min. The pellets were washed with 70% ethanol (500 μL) and centrifuged at 4,000g for 5 min. The DNA pellets were re-suspended in 50 μL de-ionized water. Two μL of this extracted DNA was used for genotyping of T-DNA insertion lines: glr3.5-a (At2g32390, salk_035264) forward, 5′–TGAAGTTGCTGCAAATGTGAG–3′; reverse, 5′–TGTCGACATGTCCACAGCTAG–3′. glr3.5-b (At2g32390, salk_111204) forward, 5′–ACCCATCTCATGTA-GGGGAAG–3′; reverse, 5′– TTCAGAAAAAGCAATCCA-TGC–3′.
Electrophysiology
The Electrical Penetration Graph (EPG) technique was re-purposed as a method for recording systemic electrical signals in plants, as described previously.Citation12,26 Probing by B. brassicae aphids was recorded with a Giga-8 Amplifier (8-channel DC system; EPG Systems, Netherlands). Signals were acquired at a sampling rate of 100 Hz, digitized with a built-in converter, and analyzed using Stylet+ software (EPG Systems, Netherlands) on a PC. Since the input resistance (Ri) of the DC-EPG amplifier was 1GΩ, the measured voltage differences were calibrated with a pulse of known voltage (in this case, 50 mV), in order to compensate for the attenuation of the membrane potential changes due to the relatively low Ri.
The aphid, the plant, and the EPG pre-amplifier were set inside a Faraday cage. Aphids for EPG recording were attached to a gold wire (φ18 μm) by means of a water-based glue droplet that contains silver particles, as originally described.Citation27,28 Aphids were placed on the adaxial side of Arabidopsis leaves of potted plants; the EPG external copper electrode was inserted in the soil. These aphids inserted their stylets into the plant just a few seconds after being placed on the leaves thus closing the EPG circuit; a preliminary starvation period was not necessary. Following a several hour- long pathway phase, aphids entered into the phloem phase, characterized by an abrupt voltage drop due to the penetration of the stylet into a sieve element (SE). The phloem phase contains two EPG waveforms with low amplitude rhythmic fluctuations that correspond to streaming potentials: the E1 and the E2 waveforms. The E1 waveform corresponds to an initial period, c. 1 minute-long, of salivation into the SE. During this initial period, there are small fluctuations of the EPG baseline,Citation12 probably corresponding to small disturbances in the membrane potential induced by the stylet puncture. The E2 waveform corresponds to sap ingestion, lasting from several minutes to several hours. All experiments were performed during the E2 waveform. In these experiments, only one aphid per plant was used, and plants where exposed to single aphids for less than 12 hours. Although aphids are known to induce the expression of plant defenses, it is unlikely that a single aphid would induce a significant response from the plant that would affect the phenotypes of the AP and the long potentials. Most studies on the defense response of plants to aphids test the effect of aphid infestations.Citation29
Wounding experiments were only performed after the aphid had been in the stable feeding phase, i.e. in E2 waveform, for at least 10 min. During this period, there were no APs or other variations in the EPG baseline indicative of changes in the membrane potential of the aphid-probed SE/CC complex that could have been induced by the stylet's puncture. Wounding was inflicted by cutting a leaf at the leaf/petiole junction, since these type of wounds induce identical electrical signals as those produced by feeding caterpillars (Pieris brassicae).Citation12 In some experiments, the cut leaf was neighbor to the EPG-recorded leaf, and in other experiments, the cut leaf was non-neighbor to the EPG-recorded one. The EPG-recorded leaves, i.e. leaves analyzed for electrophysiological responses, were unwounded leaves in most cases. For the experiments shown in , the EPG-recorded leaves were also the wounded ones. Wild type plants were examined concomitantly with mutant plants, i.e. control and test experiments were performed in parallel. On several occasions, the same aphid used to record from a mutant was re-used to record from a wild type plant, and vice versa.
Note that whereas other conventional electrophysiological rigs for whole-plant electrophysiology measure potential differences (PDs) between two shoot electrodes,Citation3,30-32 EPG measures PDs between a fine intracellular electrode specific for the SE/CC complex (represented by the aphid), and a coarse external copper electrode inserted into the root medium. shows the general configuration of the EPG rig, and illustrates the localization and recording horizons of its two measuring electrodes.
Data analyses
Statistical analyses were performed with SPSS software v. 22 (IBM Corporation, Armonk, NY). Data are given as mean ± s.e.m. Statistical differences between means of wild type and each of the mutants were determined with the Student t test for independent samples. The level of statistical significance was set at P < 0.05.
Disclosure of potential conflicts of interest
No potential conflicts of interest were disclosed.
Supplemental_Figures.zip
Download Zip (728.6 KB)Acknowledgments
I would like to thank Ted Farmer (University of Lausanne, Switzerland) for providing laboratory facilities and reagents, as well as GLR mutant seeds. I would also like to thank Rainer Hedrich (University of Würzburg, Germany) for providing laboratory facilities, and Elisa Garzo and Alberto Fereres (CSIC Madrid, Spain) for donating aphids. This research was supported by the IIF Marie Curie grant WOUND IN EARTH.
References
- Malone M. Kinetics of wound-induced hydraulic signals and variation potentials in wheat seedlings. Planta 1992; 187:505-10; PMID:24178145; http://dx.doi.org/10.1007/BF00199969
- Lippincott BB, Whatley MH, Lippincott JA. Tumor induction by Agrobacterium involves attachment of the bacterium to a site on the host plant cell wall. Plant Physiol 1977; 59:388-90; PMID:16659858; http://dx.doi.org/10.1104/pp.59.3.388
- Mousavi SAR, Chauvin A, Pascaud F, Kellenberger S, Farmer EE. Glutamate receptor-like genes mediate leaf-to-leaf wound signalling. Nature 2013; 500:422-6; PMID:23969459; http://dx.doi.org/10.1038/nature12478
- Hille B. Ion channels of excitable membranes. Sinauer Associates, Inc. Sunderland, Massachusetts U.S.A. 3rd edition. 2001.
- Ward JM, Mäser P, Schroeder JI. Plant ion channels: gene families, physiology, and functional genomics analyses. Ann Rev Physiol 2009; 71:59-82; http://dx.doi.org/10.1146/annurev.physiol.010908.163204
- Lam H-M, Chiu J, Hsieh M-H, Meisel L, Oliveira IC, Shin M, Coruzzi G. Glutamate receptor genes in plants. Nature 1998; 396:125-6; PMID:9823891; http://dx.doi.org/10.1038/24066
- Traynelis SF, Wollmuth LP, McBain CJ, Menniti FS, Vance KM, Ogden KK, Hansen KB, Yuan H, Myers SJ, Dingledine R. Glutamate receptor ion channels: structure, regulation, and function. Pharmacol Rev 2010; 62:405-96; PMID:20716669; http://dx.doi.org/10.1124/pr.109.002451
- Colquhoun D, Sivilotti LG. Function and structure in glycine receptors and some of their relatives. Trends Neurosci 2004; 27:337-44; PMID:15165738; http://dx.doi.org/10.1016/j.tins.2004.04.010
- Roy SJ, Gilliham M, Berger B, Essah PA, Cheffings C, Miller AJ, Davenport RJ, Liu L-H, Skynner MJ, Davies JM, Richardson P, Leigh RN, Tester M. Investigating glutamate receptor-like gene co-expression in Arabidopsis thaliana. Plant Cell Environ 2008; 31:861-71; PMID:18284583; http://dx.doi.org/10.1111/j.1365-3040.2008.01801.x
- Taniura H, Sanada N, Kuramo N, Yoneda Y. Evolutional divergence of the metabotropic glutamate receptor genes: a new receptor belonging to the metabotropic glutamate receptor family in Dictostelium discoideum. Curr Genomics 2006; 7:245-52; http://dx.doi.org/10.2174/138920206778426979
- Kunishima N, Shimada Y, Tsuji Y, Sato T, Yamamoto M, Kumasaka T, Nakanishi S, Jingami H, Morikawa K. Structural basis of glutamate recognition by a dimeric metabotropic glutamate receptor. Nature 2000; 407:971-77; PMID:11069170; http://dx.doi.org/10.1038/35039564
- Salvador-Recatalà V, Tjallingii WF, Farmer EE. Real-time, in vivo intracellular recordings of caterpillar–induced depolarization waves in sieve elements using aphid electrodes. New Phytol 2014; 203:674-84; http://dx.doi.org/10.1111/nph.12807
- Blair Hedges S, Blair JE, Venturi ML, Shoe JL. A molecular timescale of eukaryote evolution and the rise of complex multicellular life. BMC Evol Biol 2004; 4:2; PMID:15005799; http://dx.doi.org/10.1186/1471-2148-4-2
- Chiu J, DeSalle R, Lam H-M, Meisel L, Coruzzi G. Molecular evolution of glutamate receptors: A primitive signaling mechanism that existed before plants and animals diverged. Mol Biol Evol 1999; 16:826-38; PMID:10368960; http://dx.doi.org/10.1093/oxfordjournals.molbev.a026167
- Curran ME, Splawski I, Timothy KW, Vincent GM, Green ED, Keating MT. A molecular basis for cardiac arrhythmia: HERG mutations cause long QT syndrome. Cell 1995; 80:795-803; PMID:7889573; http://dx.doi.org/10.1016/0092-8674(95)90358-5
- Byzov AL, Shura-Bura TM. Electrical feedback mechanism in the processing of signals in the outer plexiform layer of the retina. Vis Res 1986; 26:33-44; PMID:3012877; http://dx.doi.org/10.1016/0042-6989(86)90069-6
- Lin Y, Chen C, Chen L, Zeng S, Luo Q. The analysis of electrode-recording horizon. Conf Proc IEEE Eng Med Biol Soc 2005; 7:7345-8; PMID:17281977; http://dx.doi.org/10.1109/IEMBS.2005.1616208
- Maathuis FJM, Sanders D. Contrasting roles in ion transport of two K+-channel types in root cells of Arabidopsis thaliana. Planta 1995; 197:456-64; PMID:8580759; http://dx.doi.org/10.1007/BF00196667
- Diatloff E, Roberts M, Sanders D, Roberts SK. Characterization of anion channels in the plasma membrane of Arabidopsis epidermal root cells and the identification of a citrate-permeable channel induced by phosphate starvation. Plant Physiol 2004; 136:4136-49; PMID:15563625; http://dx.doi.org/10.1104/pp.104.046995
- Hejnowicz Z, Krause E, Glebicki K, Sievers A. Propagated fluctuations of the electric potential in the apoplasm of Lepidium sativum L. roots. Planta 1991; 186:127-34; PMID:24186585; http://dx.doi.org/10.1007/BF00201508
- Masi E, Ciszak M, Stefano G, Renna L, Azzarello E, Pandolfi C, Mugnai S, Baluška F, Arecchi FT, Mancuso S. 2008. Spatiotemporal dynamics of the electrical network activity in the root apex. Proc Nat Acad Scis USA 2008; 106:4048-53; http://dx.doi.org/10.1073/pnas.0804640106
- Singh SK, Chien CT, Chang IF. The Arabidopsis glutamate receptor-like gene GLR3.6 controls root development by repressing the Kip-related protein gene KRP4. J Exp Bot 2016; pii:erv526; PMID:26773810; http://dx.doi.org/10.1093/jxb/erv576
- Qiu C, Shivacharan RS, Zhang M, Durand DM. Can neural activity propagate by endogenous electrical field? J Neurosci 2015; 35:15800-11; PMID:26631463; http://dx.doi.org/10.1523/JNEUROSCI.1045-15.2015
- Kong D, Ju C, Parihar A, Kim S, Cho D, Kwak JM. Arabidopsis glutamate receptor homolog 3.5 modulates cytosolic Ca2+ level to counteract effect of abscisic acid in seed germination. Plant Physiol 2015; 167:1630-42; PMID:25681329; http://dx.doi.org/10.1104/pp.114.251298
- Vincill ED, Clarin AE, Molenda JN, Spalding EP. Interacting glutamate receptor-like proteins in phloem regulate latéral root initiation in Arabidopsis. Plant Cell 2013; 25:1304-13; PMID:23590882; http://dx.doi.org/10.1105/tpc.113.110668
- Salvador-Recatalà V, Tjallingii WF. A new application of the Electrical Penetration Graph (EPG) for acquiring and measuring electrical signals in phloem sieve elements. J Vis Exp 2015; 101:e52826; PMID:26168018; http://dx.doi.org/10.3791/52826
- McLean DL, Kinsey MG. A technique for electronically recording aphid feeding and salivation. Nature 1964; 202:1358-9; http://dx.doi.org/10.1038/2021358a0
- Tjallingii WF. Electronic recording of penetration behavior by aphids. Entomol Exp Appl 1978; 24:721-30; http://dx.doi.org/10.1111/j.1570-7458.1978.tb02836.x
- Zhu-Salzman K, Salzman RA, Ahn J-E, Koiwa H. Transcriptional regulation of sorghum defense determinants against a phloem-feeding aphid. Plant Physiol 2004: 134:420-31; PMID:14701914; http://dx.doi.org/10.1104/pp.103.028324
- Rhodes, JD, Thain JF, Wildon DC. The pathway for systemic electrical signal conduction in the wounded tomato plant. Planta 1996; 200:50-7; http://dx.doi.org/10.1007/BF00196648
- Stanković B, Davies E. Both action potentials and variation potentials induce proteinase inhibitor gene expression in tomato. FEBS Lett 1996; 390:275-9; PMID:8706876; http://dx.doi.org/10.1016/0014-5793(96)00672-2
- Zimmermann MR, Maischak H, Mithöfer A, Boland W, Felle HH. System potentials, a novel electrical long-distance apoplastic signal in plants, induced by wounding. Plant Physiol 2009; 149:1593-1600; PMID:19129416; http://dx.doi.org/10.1104/pp.108.133884
- Dengler NG. The shoot apical meristem and development of vascular architecture. Can J Bot 2006; 84:1660-71; http://dx.doi.org/10.1139/b06-126