ABSTRACT
Diseases caused by plant-parasitic nematodes in vegetables, among them Meloidogyne spp. root-knot nematodes (RKNs), lead to extensive yield decline. A molecular understanding of the mechanisms underlying plants’ innate resistance may enable developing safe alternatives to harmful chemical nematicides in controlling RKNs. A tight relationship has been revealed between the WRKY transcription factors and RKN parasitism on tomato roots. We investigated the function role of tomato SlWRK3 and SlWRKY35 in regulating nematode disease development. Using promoter–GUS reporter gene fusions, we show that both SlWRKY3 and SlWRKY35 are induced within 5 days of infection and through feeding-site development and gall maturation, with a much stronger response of the former vs. the latter to nematode infection. Histological analysis of nematode-feeding sites indicated a high expression of SlWRKY3 in developing and mature feeding cells and associated vasculature cells, whereas SlWRKY35 expression was only observed in mature feeding sites. Both SlWRKY3 and SlWRKY35 promoters were induced by the defense phytohormones salicylic acid and indole-3-butyric acid, with no response to either jasmonic acid or methyl jasmonate. SlWRKY3 overexpression resulted in lower infection of the RKN Meloidogyne javanica, whereas knocking down SlWRKY3 resulted in increased infection. Phytohormone and oxylipin profiles determined by LC–MS/MS showed that the enhanced resistance in the former is coupled with an increased accumulation of defense molecules from the shikimate and oxylipin pathways. Our results pinpoint SlWRKY3 as a positive regulator of induced resistance in response to nematode invasion and infection, mostly during the early stages of nematode infection.
Introduction
Among plant-parasitic nematodes, root-knot nematode (RKN) (Meloidogyne spp.) is considered the most widely distributed and economically important pest of a wide range of crop plants. As an obligate biotrophic parasite, RKN establishes and maintains an intimate relationship with its host plants that results in hyperplasia of the surrounding feeding cells leading to organogenesis of a typical root gall.Citation1 Within the stele, there is a unique specialized feeding site that is characterized by repeated rounds of nuclear division and cell growth in the absence of cytokinesis, leading to the formation of multinucleate, hypertrophied giant cells that are up to 100 times the size of normal root vascular parenchyma cells. This feeding site construction facilitates nutrient extraction from the enlarged plant cells, ensuring nematode growth and reproduction.Citation2,Citation3 A study of the regulation of plant gene expression upon nematode infection revealed the involvement of major developmental reprogramming processes,Citation4 involving genes related to metabolism, stress responses, protein synthesis, cell division, transport, and signal transductionCitation5-Citation7
A search for transcription factors (TFs) underlying this RKN-mediated cell reprogramming revealed WRKY – one of the largest TF families – with a broad-spectrum role as positive and negative regulators of abiotic and biotic stress in plants.Citation8,Citation9 As TFs, WRKYs can activate or limit the transcription of genes’ targets by directly binding to their promoters in a sequence-specific manner.Citation10,Citation11 Characteristics of WRKYs include the presence of one or two highly conserved WRKY domains and a zinc-finger motif in the C-terminal region.Citation12 WRKY proteins containing a single WRKY domain with the C2-H2 (C-X4-5-C-X22-23-H-X1-H) pattern are group I, and those containing two WRKY domains followed by a C2-H2 are group II; WRKY proteins containing a single WRKY domain with the C2-HC (C-X7-C-X23-H-X1-C) pattern are group III; group IV consists of WRKY proteins that contain a WRKY domain but lack a complete zinc finger.Citation12,Citation13 The WRKY domain can bind to TTGAC(C/T) of the W-box found in promoters of target genes and regulate their transcription.Citation14 In recent years, WRKY TFs have been intensively studied for their function in regulating the transcriptional reprogramming associated with plant immunity.Citation12 Whereas the contribution of individual WRKY proteins to the outcome of defined plant–pathogen or plant–pest interactions is often subtle, the concerted activity of multiple members of this family is likely to be of key importance for efficient control of immune responses, often referred to as the WRKY web.Citation15
WRKYs’ involvement in regulating plant-parasitic nematode infections has been studied in Arabidopsis, rice, tomato, pepper, and soybean hosts.Citation16–Citation19 In Arabidopsis, Grunewald et al.Citation20 showed that feeding-site establishment by the cyst nematode Heterodera schachtii is accompanied by increased expression of the auxin-inducible gene AtWRKY23. Later, Ali et al.Citation21 demonstrated that among 59 WRKYs represented on an Arabidopsis ATH1 GeneChip, 28 genes were downregulated and 6 upregulated, whereas overexpression of the respective genes demonstrated that their downregulation is important for nematode development, probably via interference with plant defense signaling. In a rice-migratory nematode (Hirschmanniella oryzae) system, strong upregulation of three TFs – OsWRKY62, OsWRKY70, and OsWRKY11 – 3 days after migratory nematode infection was observed. Similarly, strong upregulation was detected for transcripts encoding OsWRKY62, OsWRKY59, and OsWRKY13, following rice infection by the RKN Meloidogyne graminicola.Citation16,Citation17 Overexpression of three WRKY genes in a cyst-nematode-susceptible soybean cultivar displayed increased resistance to the soybean–cyst nematode interaction.Citation19 In tomato, a genome-wide computational analysis revealed 81 WRKY genes.Citation9 To date, the function of four WRKY TFs implicated in the tomato–RKN interaction has been studied with respect to their role in regulating plant defense response. The first report revealed that WRKYs function in the pathway mediated by RKN resistance gene showing that paralogues – SlWRKY72a and b – were upregulated during disease resistance mediated by Mi-1; virus-induced gene silencing of these two genes in tomato resulted in a clear reduction of Mi-1-mediated resistance against RKNs.Citation22 Subsequently, Atamian et al.Citation23 showed that silencing of SlWRKY70 attenuates Mi-1-mediated resistance against RKN, and that SlWRKY70 transcript levels are upregulated by salicylic acid (SA) and suppressed by methyl jasmonate (MeJA). In tomato, at least 19 WRKY genes from the tomato genome were found to be differentially expressed in response to nematode infection;Citation24,Citation25 one of them – SlWRKY45 – was recently shown to enhance susceptibility to nematodes.Citation24,Citation25 Here, we report on the involvement of two more WRKYs – SlWRKY3 and SlWRKY35 – during tomato–nematode infections. Our results demonstrate that both genes are induced upon nematode infection, with WRKY3 exhibiting a stronger induction compared to WRKY35. To provide genetic evidence for the role of SlWRKY3 in the response to RKN, overexpression and knockout lines were employed to measure nematode performance and host phytohormones and metabolite response. Knowledge gained from this study may be extrapolated to understand the contribution of the WRKY web to the intimate molecular dialog governing the tomato response to RKN infection.
Results
SlWRKY3 and SlWRKY35 are canonical WRKY transcription factors
To gain further insight into the role of WRKY TFs in mediating RKN parasitism on tomato,Citation24,Citation25 we studied the functional characteristics of two WRKYs revealed by a recent RNA-Seq analysis of tomato roots following M. javanica infection: SlWRKY3 (Solyc02g088340.2.1; accession no. KU674829) and SlWRKY35 (solyc02g021680.2.1; accession no. XM_010317323). In-silico analysis indicated that SlWRKY3 consists of 461 amino acids from four exons encoding 132, 238, 16, and 75 amino acids, respectively, and contains two WRKY domains (WRKYGQK/WRKYGQK) and a zinc-finger-like motif ligand, C-X6-C-X27-H-X1-H. The presence of a nuclear-localization signal sequence (KKKVER) at position 250, PTKRRK at position 275, RKYGQK at position 223 and RKYGQK at position 393, supports its function as a TF. In light of these domains and features, this protein was assigned by Huang et al.Citation9 to group I of the WRKY TFs. SlWRKY35 consists of 381 amino acids from three exons encoding 147, 47, and 187 amino acids, respectively, and contains only one WRKY domain (WRKYGQK) and a zinc-finger-like motif ligand, C-X5-C-X23-H-X1-H. The presence of nuclear-localization signal sequence TGIKRRKSQ at position 143 agrees with its function as a TF, and this protein was assigned by Huang et al.Citation9 to WRKY group II-e.
M. javanica infection induces SlWRKY3 and SlWRKY45 in roots
The full-length SlWRKY3 and SlWRKY35 promoter regions, 1973 bp and 1959 bp, were respectively isolated from tomato DNA and cloned, resulting in the final binary vectors carrying a promoter–GUS fusion product; this product was used to generate SlWRKY3::GUS and SlWRKY35::GUS tomato hairy root reporter gene lines via Rhizobium rhizogenes-mediated transformation. To analyze expression profiles of both SlWRKY lines following inoculation, root tissues were checked for GUS signal at 2, 5, 15, and 28 days after inoculation (dai) with second-stage juveniles (J2s) of M. javanica, and compared with GUS expression in the respective noninoculated root lines. For SlWRKY3 at 2 dai, a weak signal could be detected proximate to the invading nematode in the root elongation zone restricted to the stele (). At 5 dai, a strong GUS signal was observed in the vascular system and the swelling root tissue associated with the invading nematode (). At 15 and 28 dai, the GUS signal gradually increased in all infected root regions containing the developed gall, showing a dark blue signal (), with no signal observed in noninoculated roots (). SlWRKY35 expression pattern consisted of an increasing GUS signal at the earlier time points, from 2 to 15 dai, limited to the vascular root system (), with no GUS signal in noninoculated roots (). In contrast to SlWRKY3, SlWRKY35 the observed GUS signal decreased at 28 dai, with only a faint signal in the mature galls (). Thus, the GUS assay confirms the upregulation of SlWRKY3 and SlWRKY35 during RKN infection.Citation24,Citation25
Figure 1. Microscopic analysis of β-glucuronidase (GUS) expression patterns in root-knot nematode (RKN)-infected tomato roots harboring the SlWRKY3 and SlWRKY35 promoter–GUS fusion constructs. (A–H) Micrographs of SlWRKY3::GUS reporter line. (A) Noninoculated root harboring the WRKY3:GUS fusion construct exhibited no GUS signal in root tip or elongation zone. (B) Infected roots at 2 dai. (C) Noninoculated roots at 5 days. (D) Infected roots at 5 dai. (E) Noninoculated roots at 15 days. (F) Developing galls in infected roots at 15 dai. (G) Noninoculated roots at 28 days. (H) Mature galls in infected roots at 28 dai. (I–P) Micrographs of SlWRKY35:GUS reporter line. (A) Noninoculated root harboring the WRKY35:GUS fusion, exhibiting no GUS signal in root tip or elongation zone. (B) Infected roots at 2 dai. (C) Noninoculated roots at 5 days. (D) Infected roots at 5 dai. (E) Noninoculated roots at 15 days. (F) Developing galls in infected roots at 15 dai. (G) Noninoculated roots at 28 days. (H) Mature galls in infected roots at 28 dai. Arrows indicate nematodes. (A–E, G, I–L): micrographs as viewed under light microscope. (F, H, M–P): bright-field image of galls photographed through a stereomicroscope. Bars: (A–E, G, I–L) 50 μm; (F, H, M–P) 500 μm.
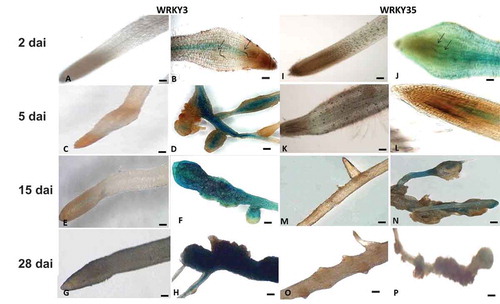
SlWRKY3 and SlWRKY35 are expressed in developing and mature feeding sites
To determine the involvement of SlWRKY3 and SlWRKY35 during feeding-site establishment, thin sections of galls expressing SlWRKY3 and SlWRKY35 promoter–GUS constructs were analyzed at 15 and 28 dai. At 15 dai, SlWRKY3 showed expression associated with the endodermis and attached pericycle cells, with a visible signal in the developing feeding sites, as indicated by the red precipitate obtained with dark-field optics (). At 28 dai, expression spread to the phloem as well as to the mature feeding sites, as indicated by the pronounced GUS signal observed by light- and dark-field microscopy (). Feeding sites associated with the developing nematodes in SlWRKY35::GUS lines demonstrated no GUS signal in the developing feeding sites at 15 dai (), whereas an apparent signal was observed in the mature feeding sites at 28 dai (), which suggests that SlWRKY35 interact primarily within mature feeding sites.
Figure 2. SlWRKY3 and SlWRKY35 expression in feeding sites induced upon root-knot nematode (RKN) infection. Microscopic analyses of β-glucuronidase (GUS) activity in cross sections of tomato root galls expressing WRKY3 and WRKY35 promoter–GUS constructs at 15 and 28 dai. At these time points, all observed giant cells were already mature. Sections of galls induced in WRKY3:GUS line (a, b) and WRKY35:GUS line (E, F) at 15 dai. Sections of galls induced in WRKY3:GUS line (c, d) and WRKY35:GUS line (g,h) at 28 dai. N, body of female nematode seen at the edge of the *, giant cells. Bars, 200 μm. GUS staining is observed as blue color in whole mounts, and as a red precipitate in the dark-field micrographs.
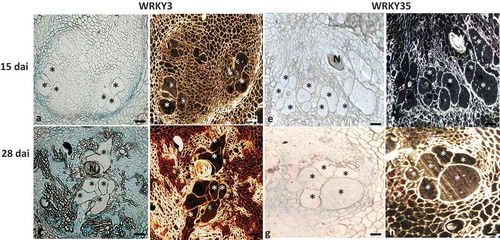
SlWRKY3 and SlWRKY35 are wound inducible in roots
As nematode penetration causes mechanical damage by generating cell wall fragments that can act as damage associated molecular pattern similar with wounding responses,Citation26 the expression of SlWRKY3 and SlWRKY35 was assessed 9 and 24 h after a wounding treatment by promoter-GUS fusion assay. The line harboring the SlWRKY3–GUS construct showed a remarkable increase in GUS signal, confined to the root stele, as early as 9 h after wounding, and persisting until 24 h after wounding (). In contrast, the GUS signal following wounding of line SlWRKY35 was delayed, and only weakly observed 24 h after wounding (). In addition to the wound-induced GUS activity found in local tissue, both SlWRKY3 and SlWRKY35 were found to be systemically induced in the vasculature in nonwounded tissue. These results indicate that both SlWRKY3 and SlWRKY35 are wound-induced and suggest that they are involved in the transcriptional reprogramming associated with local and systemic wound responses.
Salicylic acid and indole-3-butyric acid induces expression of SlWRKY3 and SlWRKY35
As WRKYs are frequently induced by phytohormone signaling, a pharmacological approach was employed to investigate the regulation of SlWRKY3 and SlWRKY35 expression in tomato roots by the phytohormones SA, jasmonic acid (JA), MeJA, NCitation6-benzyladenine (BA), indole-3-acetic acid (IAA), and indole-3-butyric acid (IBA). Hormones were applied to 2-week-old dark-grown tomato roots (grown under prolonged darkness), and GUS expression was monitored in the root tips and along the roots following the treatments. SlWRKY3 moderate expression was induced by 1 mM SA in the elongation zone and middle root portion compared to controls (, a–c). SlWRKY3 was also induced by IBA treatment, as shown by a mild GUS signal in the elongation zone and mature roots at 1 and 10 µM IBA compared to controls (, d–f). Application of exogenous IAA and BA did not induce any visible SlWRKY3 expression (, g–i and J–L, j–l, respectively). Similarly, among the jasmonates, neither JA nor MeJA affected SlWRKY3 expression in the root elongation zone or mature roots (, m–o; P–R, p–r, respectively). For SlWRKY35, the application of SA-induced expression in the root tip localized within the vascular cylinder (, a–c). IBA induced a similar pattern of expression, as observed by GUS signal within the vascular bundles (, d–f). SlWRKY35 expression was neither induced by IAA, BA, JA, nor by MeJA treatment (, respectively). Taken together, these observations suggest a role for SlWRKY3 and SlWRKY35 in mediating the downstream signaling events governed by SA and IBA.
Figure 4. Effect of exogenous phytohormone application on GUS expression in WRKY3:GUS root line. Subcultured roots (7 days old) were transferred to GB as control (A,D,G,J,M,P and a,b,g,j,m,p) or to GB containing SA (1, 5 mM; B,C, b,c), IBA (1, 10 µM; E,F, e,f), IAA (1, 5, µM; H,I; h,i), BA (0.1, 0.5 µM; K,L, k,l), JA (10, 20 µM; N,O, n,o), MeJA (0.01, 0.1 mM; Q,R, q,r) for 16 h before root staining. GUS was detected histochemically and root elongation zone (A–R) or mature roots (a–r) were monitored. Figures are representative of at least five independent experiments. Scale bar = 0.5 mm.
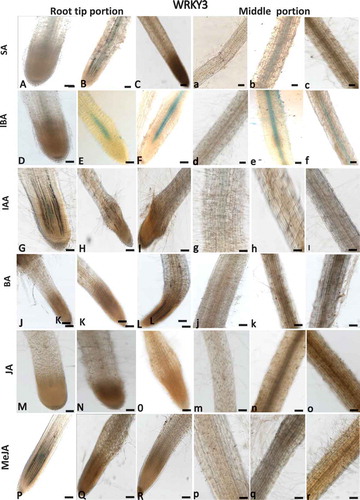
Figure 5. Effect of exogenous phytohormone application on GUS expression in WRKY35:GUS root line. Subcultured roots (7 days old) were transferred to GB as control (A,D,G,J,M,P and a,b,g,j,m,p) or to GB containing SA (1, 5 mM; B,C, b,c), IBA (1, 10 µM; E,F, e,f), IAA (1, 5 µM; H,I; h,i), BA (0.1, 0.5 µM; K,L, k,l), JA (10, 20 µM; N,O, n,o), MeJA (0.01, 0.1 mM; Q,R, q,r) for 16 h before root staining. GUS was detected histochemically and root elongation zone (A–R) or mature roots (a–r) were monitored. Figures are representative of at least five independent experiments. Scale bar = 0.5 mm.
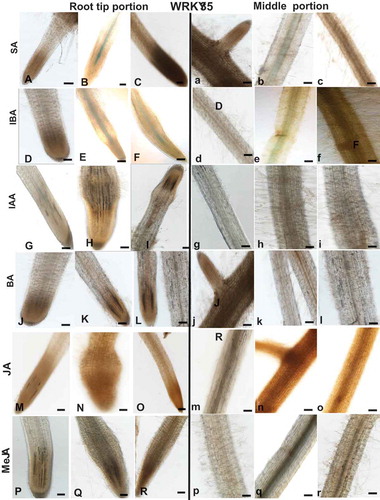
SlWRKY3 governs development and fecundity of M. javanica on tomato
As SlWRKY3 expression was the most responsive to nematode, wounding, and hormonal treatment, its role in nematode infection was further explored with overexpression and genetic knockout tools. Plant binary vector containing the 1383-bp SlWRKY3 open reading frame under the control of the CaMV 35S promoter was introduced into tomato by R. rhizogenes-mediated transformation. A total of 30 kanamycin-resistant putative transformants were obtained and maintained on selective media. Similarly, SlWRKY3 RNAi-silenced lines were generated by amplifying a 571-bp product and were further subcloned in pHANNIBAL vector,Citation27 generating the hairpin dsRNA complementary to SlWRKY3, under the constitutive CaMV 35S promoter, followed by cloning into the binary vector pART27 that was used for R. rhizogenes-mediated transformation. A total of 20 kanamycin-resistant putative transformants were obtained and maintained on selective media. None of the resulting transgenic hairy root lines showed any phenotypic changes compared to the wild type. Two overexpression lines (oe:wk-02, oe:wk-03), with high SlWRKY3 transcript levels according to qRT-PCR analysis, were selected for further analysis. Similarly, two RNAi lines (RNAi:wk-03, RNAi:wk-04), carrying the hairpin WRKY3 dsRNA, were chosen for further analysis (Figure S1). To determine the effect of SlWRKY3 overexpression on disease development in tomato, hairy roots from transgenic and pControl roots (carrying pART27) were inoculated with M. javanica J2s, and nematode developmental stages were monitored at 15 and 28 dai ( and ). Remarkably, SlWRKY3 overexpression resulted in an increased number of juveniles at 28 dai; however, there was a dramatic decrease of nematodes molting to the female stage and associated number of egg masses recovered (). In sharp contrast, silencing of SlWRKY3 reduced the number of J2s but increased the number of females and egg masses, at 28 dai (). Taken together, these data provide evidence that SlWRKY3 is a major player in controlling the development, reproduction, and performance of RKN.
Figure 6. Overexpression of SlWRKY3 in tomato roots increases root susceptibility to infection by the root-knot nematode M. javanica. Meloidogyne susceptibility/resistance of transgenic tomato roots overexpressing SlWRKY3 (oe) (A, B) and transgenic roots expressing WRKY3 dsRNA (RNAi) (C, D) was measured as nematode developmental stages counted at 15 and 28 dai in comparison to the pControl line. All root lines were inoculated with 300 sterile preparasitic J2s and the infected roots were assessed for development of J3/J4s and mature females at 15 (A, C) and 28 (B, D) dai for each root line, through observation under the dissecting microscope following staining with acid fuchsin dye. Data are expressed as means of 25 roots from each line; the experiment was repeated three times, giving consistent results. The percentage of each developmental stage is represented by a mean standard error. Different letters above the bars denote a significant difference (p ≤ 0.05, analysis of variance) between hairy root lines analyzed by Tukey–Kramer multiple comparison tests.
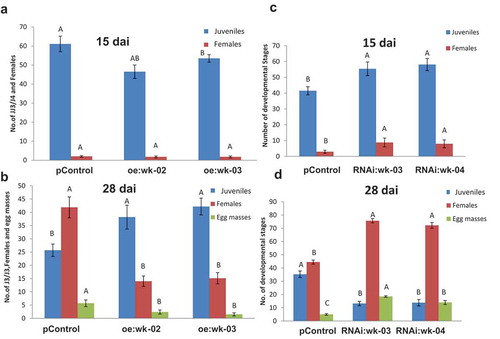
SlWRKY3 overexpression regulates oxylipin- and hormone-biosynthesis during early RKN infection processes
To begin to understand the mechanisms downstream of SlWRKY3-mediated host responses to RKN, targeted metabolite analysis was performed to investigate phytohormone and oxylipin – oxygenated fatty acids involved in defense responses against pathogensCitation28 – and related metabolites were measured in the WRKY3-overexpressing line at 5 and 15 dai using LC–MS/MS analysis (, ). The accumulation of key metabolites of the shikimate pathway was observed, e.g., an increased accumulation of cinnamic acid (CA) – a phenylpropanoid with antioxidant activity – was observed in roots overexpressing SlWRKY3 at 5 dai. The accumulation of shikimate-derived benzoates also increased in the SlWRKY3-overexpressing line, as well as that of the converted metabolite SA resulting from the activity of benzoic acid 2-hydroxylase. The level of the phytohormone IAA also increased in roots overexpressing SlWRKY3 at 5 dai (). Oxylipins of the reductase pathways, the hydroxy fatty acids 13-hydroxy octadecadienoic acid (13HOD), 13-hydroxy octadecatrienoic acid (13HOT), 9-hydroxy octadecadienoic acid (9HOD), and 9-hydroxy octadecatrienoic acid (9HOT) were significantly increased at 5 dai in the SlWRKY3-overexpressing line compared to pControl lines, with no significant differences at 15 dai (). In addition, a significant increase in the accumulation of 12, 13-epoxy-metabolites generated through the cytochrome p450 pathway was observed in roots overexpressing SlWRKY3 at 5 dai, including epoxyoctamonoenoic acid (12,13-EpOM), 12,13-epoxyoctadecadienoic acid (12,13-EpODE), and 12,13-dihydroxyoctadecenoic acid (12,13-DiHOM) diols resulting from conversion of the epoxy-metabolites by soluble epoxide hydrolase (sEH) (). Among the jasmonate group, significant differences were only observed for the bioactive isoleucine conjugate JA–Ile at 5 dai with concentrations increasing approximately 10-fold (p < 0.05) compared to pART27 control roots, with no significant differences observed for either JA or 12-OPDA (oxo-phytodienoic acid) ().
Figure 7. Analysis of major branch-point intermediates and products associated with and derived from the shikimate pathway. Changes in levels of the metabolites: cinnamic acid, benzoic acid, salicylic acid, and indole-3-acetic acid (IAA) were measured through direct LC–MS/MS analysis in negative-phase mode in root tissues at 5 and 15 dai with M. javanica J2s, and data were expressed as means of four replicates. Mock treatments consisted of inoculation with H2O only. Errors bars correspond to SD (n = 4), and different letters above the bars denote a significant difference (p < 0.05, analysis of variance) between samples, as analyzed by Tukey–Kramer multiple comparison test. Isochorismate mutase (ICS), Phenylalanine ammonia-lyase (PAL); Isochorismate pyruvate lyase (IPL); benzaldehyde dehydrogenase (BALDH); benzoic acid 2-hydroxylase (BA2H).
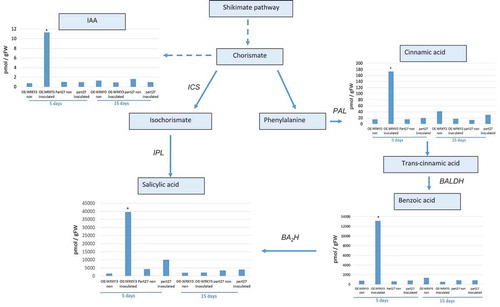
Figure 8. Analysis of products associated with and derived from the oxylipin pathway governed by lipoxygenase. Endogenous lipoxygenase (LOX), allene oxide synthase (AOS), and reductase and peroxygenase (POX)-derived oxylipins were analyzed. Oxylipin production was monitored by LC–MS/MS in negative-phase mode in root tissues at 5 and 15 dai with M. javanica J2s and data were expressed as the means of four replicates. Mock treatments consisted of inoculation with H2O only. Errors bars correspond to standard deviation (n = 4), and different letters above the bars denote a significant difference (p < 0.05, analysis of variance) between samples, as analyzed by Tukey–Kramer multiple comparison test. FA = fatty acid.
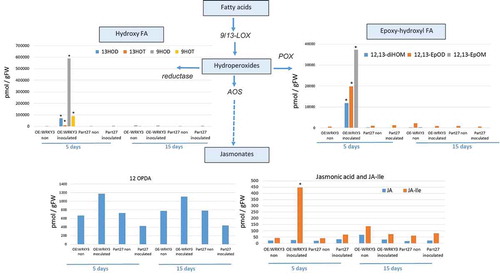
Discussion
SlWRKY3 and SlWRKY35 are implicated in regulating tomato immune response to RKNs
The direct role of several WRKY proteins in regulating plant’ defense responses to parasitic nematodes has been well demonstrated.Citation19,Citation20,Citation24,Citation25,Citation29 However, functional redundancy among certain WRKY family members exists in all studied systems, hampering attempts to functionally analyze the role of specific WRKYs in plant biological processes.Citation30 Moreover, given that a large number of plant WRKY genes are responsive to different hormones, including SA, JA, ethylene, auxin, cytokinins, ABA, and gibberellins, there is a tightly controlled and coordinated web of WRKY TFs. The tomato genome contains 81 WRKY proteins classified into four main groups, according to their number of WRKY domains and zinc-finger motifs.Citation12 A study of tomato–RKN interactions revealed the induction of several WRKYs upon nematode inoculation: Bhattarai et al.Citation22 showed that both SlWRKY72a and b are transcriptionally upregulated during disease resistance mediated by the resistance gene Mi-1, with no response to analogs of the defense hormone SA. Similarly, SlWRKY70 was shown to be required for Mi-1 activity but its expression was upregulated by SA and suppressed by MeJA.Citation23 Recent transcriptomic data of tomato roots inoculated with M. javanica have revealed differential expression of 19 WRKY-encoding transcripts.Citation24,Citation25 Among them, SlWRKY45 was induced by exogenous application of SA, IBA, IAA, and BA, whereas overexpression of SlWRKY45 resulted in promoting nematode infection, suggesting that WRKY45 TF is manipulated by the invading nematode.Citation24,Citation25 Here, the functions of two additional members, SlWRKY3 and SlWRKY35, were studied. They demonstrated increased expression upon nematode inoculation (, ) and wounding (), and upregulation following exogenous application of SA and IBA (, ). Thus, SA and IBA signaling in tomato is partially mediated by SlWRKY3 and SlWRKY35. Similar to previously studied WRKYs, they may form a regulatory positive-feedback loop that amplifies the hormonal signals.Citation31,Citation32 Both metabolites possess signaling activity relevant to RKN infection and disease development. SA is the classical phytohormone that induces immunity against biotrophic and hemibiotrohic pathogens. This might explain the disease attenuation observed in the SlWRKY3-overexpressing roots line (). Indole compounds such as IBA are found in extracts of galls incited on tomato roots by Meloidogyne hapla, M. javanica, and M. incognitaCitation33,Citation34 . As an IAA precursor, IBA maintains IAA level and distribution within the plant to support normal development.Citation35 The role of auxin in regulating RKN disease is well understood: the auxin-insensitive tomato mutant dgt does not support RKN development due to arrested early feeding-site formation.Citation36 Results from these studies indicate that auxin accumulates early in RKN-induced feeding sites to ensure nematode development.Citation37,Citation38 Taken together, our results support SlWRKY3 and SlWRKY35 regulators of pathways being responsible for the activation of local as well as systemic defense responses (termed as systemic acquired resistance).Citation39,Citation40
SlWRKY3 activates the shikimate pathway
Several studies have connected WRKY proteins to the regulation of plant metabolism.Citation41 Among them, SPF1 regulates the expression of β-amylase involved in catabolism of starch into sugars.Citation42 Similarly, recent studies indicated that WRKY TFs regulate phosphate acquisition,Citation43 and the biosynthesis of ligninCitation19,Citation44 and other secondary metabolites, including pharmaceutically valuable ones.Citation45 In our study, overexpression of SlWRKY3 induced the accumulation of several metabolites of the shikimate pathway whose end product, chorismate, is the precursor of a multitude of secondary metabolites that play important roles in the interaction of plants with their environments (). LC–MS/MS analysis of roots overexpressing SlWRKY3 clearly showed the increased accumulation of certain metabolites belonging to the shikimate pathway, i.e., CA, BA, and SACitation46 (). SA is an important part of the Mi-1-mediated defense response to RKNs in tomato.Citation47 Although Bhattarai et al.Citation48 suggested that low levels of SA are already sufficient for basal resistance to RKN in Solanum lycopersicum, Wubben et al.Citation49 showed that SA-deficient Arabidopsis thaliana mutants sid2-1, pad4-1, and NahG exhibit increased susceptibility to H. schachtii. Implication of SA in RKN disease defense signaling has been widely studied; exogenous application of SA to tomato seedlings limits RKN reproduction,Citation50 likewise exogenous application of benzothiadiazole, a functional analog of SA, reduced Meloidogyne spp. egg development and deposition on grapevine roots,Citation51 while the application of SA suppressed M. incognita infestation on okra and cowpea.Citation52 Our results clearly show an increased accumulation of key metabolites of the shikimate pathway, including the phytohormone SA in roots overexpressing SlWRKY3. These observations are correlate with the pronounced attenuation of disease development in roots overexpressing SlWRKY3 (, ). Furthermore, the early nature of the response seen at 5 dai highlights the importance of timing for this pathway’s elicitation. Apparently, differences in infection level are observed only at 28 dai; however, the increased number of J3/4 observed at 28 dai already indicates the suppression of nematode development in roots overexpressing WRKY3, occurred at the earlier time point. Given that these metabolites only increased accumulation in inoculated lines overexpressing SlWRKY3, the pathway under WRKY3 regulation requires additional factors, caused by nematode infection for their induction. Induction of IAA was also found in RKN-infected SlWRKY3-overexpressing roots (), and as indicated previously; while IAA has is important for early feeding-site formation,Citation36 its induction suggests other signaling pathways are more relevant for SlWRKY3-mediated RKN disease attenuation
SlWRKY3 activates distinct branches of tomato oxylipin biosynthesis in a RKN-inducible manner
The role of SlWRKY3 in the regulation of the plant oxylipin metabolome was also explored (). Oxylipins include a large number of structurally diverse compounds formed by the oxygenation of fatty acids in response to abiotic and biotic stresses.Citation53,Citation54 In plants, the oxylipin pathway is initiated by the coordinated action of lipases and lipoxygenases, resulting in hydroperoxides which are the substrates for downstream enzymes.Citation55 Among these pathways, epoxy-hydroxy fatty acid metabolites arises from hydroperoxides via the epoxyalcohol synthase (EAS) branch.Citation56 Among them, 12,13-EpOM, 12,13-DiHOM, and 12,13-EpOD () were highly induced in roots overexpressing WRKY3. In 2006, Hanano et al.Citation57 implicated oxylipins from the peroxygenase pathway in the response to environmental stress or in the biosynthesis of cutin polymers for the plant surface area. Furthermore, Meesapyodsuk and QiuCitation58 showed that the accumulation of peroxygenase pathway products is stored and used as a precursor for the synthesis of active oxylipins in germinating seeds, suggesting a function in inducing defense systems against abiotic and biotic stresses during seed germination and seedling establishment. Likewise, the accumulation of 12,13 EPOM might be related to any toxic activity as in mammalian system its paralogue vernolic acid (leukotoxin) has been demonstrated to possess cytotoxin properties.Citation59 An additional branch that showed significant accumulation in our study was the reductase pathway leading to accumulation of hydroxyl fatty acids 13-HOD, 13-HOT, 9-HOD, and 9-HOT. Vellosillo et al.Citation60 indicated that changes induced by 9-HOT treatment modulate root development through cell wall modification. This conclusion was based on treatment with 9-HOT leading to the formation of polysaccharide deposits composed of callose (β-1,3-glucan) and pectin (complex galacturonans), which are components of the cell wall, and to the production of reactive oxygen species, namely superoxide ion, whose deposition and subsequent modification allowed growth and development.Citation61,Citation62 Among the tested jasmonates, increased accumulation of JA–Ile was observed in roots overexpressing SlWRKY3 with no observed changes in JA or OPDA. Given that JA and SA signaling usually act antagonistically, although synergy between these two phytohormones has also been observed,Citation63 it is suggested that an increased level of JA–Ile is required to regulate the dynamic balance between SA and JA that will determine nematode accommodation in the roots. The two hormones may also regulate distinct aspects of defense against RKN as was shown for Trichoderma-mediated RKN resistance.Citation64
Remarkable conclusions
Ongoing efforts toward disentangling the WRKY web in diverse biological processes for any plant species has been challenging due to the large number of gene members and interaction. Our findings provide convincing genetic evidence to shed light on the involvement of two WRKY members in their regulation of RKN disease development in tomato. Previously, while SlWRKY45 had a positive influence on the outcome of this plant-pathogen interaction,Citation25 SlWRKY3 seemed to negatively regulate nematode accommodation in roots. Our results suggest that activation of the biosynthesis for oxylipins and shikimate metabolites underlying RKN disease attenuation. Future work should identify the cellular/nuclear component that interacts with SlWRKY3 at specific target gene sites and regulates defense signaling. Furthermore, while most of the WRKY genes in tomato are still uncharacterized, information regarding WRKYs’ involvement in nematode diseases on tomato is attracting attention, especially as possible target genes for use by the genome-editing tool CRISPR/Cas9, to confer broad-spectrum resistance to plant-parasitic nematodes.Citation65
Materials and methods
Sequence alignment and phylogenetic analyses
The tomato SlWRKY3 protein sequence (Solyc02g088340.2.1; accession KU674829) was classified by Huang et al.Citation9 into group I of the WRKY TFs, comprised of 15 members in tomato. Tomato WRKY35 protein sequence (solyc02g021680.2.1; accession no. XM_010317323) was classified into group II-e, comprising 17 members in tomato.Citation9
SlWRKY3 and SlWRKY35 sequences were downloaded from the solgenomic website (https://solgenomics.net/) and their active domains were analyzed using the NCBICDD server (http://www.ncbi.nlm.nih.gov/Structure/cdd/cdd). The functional motifs in SlWRKY were identified using MEME (Multiple Expectation Maximization for Motif Elicitation) Suite 5.0.2 at http://meme.nbcr.net/Citation66. Conserved secondary-structure features along with potential predicted motifs for SlWRKY3 and SlWRKY35 proteins were analyzed using PHD,Citation67 Jpred algorithms,Citation27 and MEME program (Suite 5.0.2) available at http://meme-suite.org/tools/meme.
Nematode inoculum and infection procedure
M. javanica populations were maintained in greenhouse-grown tomato plants (Solanum lycopersicum cv. Avigail 870). Eggs were extracted from infected roots with 0.05% (v/v) sodium hypochlorite and sucrose flotation.Citation68 For the in-vitro infection experiment, eggs were passed through a sterile Whatman H filter holder (Whatman International Ltd., Dassel, Germany) preassembled with a cellulose-acetate filter membrane (Sartorius Stedim Biotech GmbH, Gottingen, Germany, pore size 5 mm). Filter containing eggs was exposed to 0.01% (w/v) mercury chloride (HgCl2) (Sigma-Aldrich, St. Louis, MO), followed by 0.7% (w/v) streptomycin solution for 10 min. Excess HgCl2 and streptomycin were washed away in three rinses with 50-mL sterile distilled water.Citation69 Then sterile eggs were collected and placed in 0.01 M MES (Sigma-Aldrich) on a 30-µm sieve in a sterile petri plate. The plates were kept in the dark for 4–6 days. Then, freshly hatched sterile J2s were collected in a 50-mL falcon tube. For the infection test, control and transgenic tomato roots, grown in standard Gamborg’s medium (Duchefa, Haarlem, The Netherlands), were inoculated with 300 freshly hatched sterile preparasitic J2s. Plates were left open until water had completely evaporated from the media.Citation70 Both inoculated and noninoculated plates were kept horizontally under controlled conditions.
Plant materials and growth conditions
Tomato cv. Avigail 870 was used in the transformation as a background line. Seeds were soaked in 1.6% NaOCl in a sterile conical flask for 10 min with moderate shaking, washed with sterile water for 5 min, and then placed on standard Gamborg’s medium with 2% (w/v) sucrose and 0.8% (w/v) Gelrite agar (Duchefa). Plates were kept in the dark for 2 days at 26°C, and then under a 16/8-h photoperiod for an additional 2 weeks until cotyledons emerged; the well-developed cotyledons were used immediately for co-cultivation.
Plasmid construction
All PCR amplifications used for plasmid construction were performed using Recombinant Taq DNA polymerase (Thermo Scientific, Surrey, UK), according to the manufacturer’s instructions. For SlWRKY3 and SlWRKY35 promoter region amplification, tomato genomic DNA was used as the template for the PCRs with the following primers: for SlWRKY3, WRKY3Fp (5’-GGTCATCCTTTTTAAAACACAGAGCTCAAT-3’) and WRKY3Rp (5’-TGAGTTATACCCCGGGTTTCAGTTTTCTTCTTCTTC-3’), giving 1983-bp products in which restriction sites Eco53kl and SmaI were inserted at the 5’ and 3’ ends, respectively; for SlWRKY35, primer pair WRKY35Fp (5’-GATCTCAAATAATGAGCTCTGACTCA-3’) and WRKY35Rp (5’-GTAACCATAACCCGGGCATAGATAAATCACTTC-3’), giving a 1959-bp WRKY35 amplicon and with SacI and SmaI restriction sites inserted at the 5’ and 3’ ends of the promoter, respectively. The SmaI restriction site was placed before the ATG start codon of the GUS-encoding gene to guarantee the correct reading frame when the promoter was fused to the GUS gene. WRKY35 and WRKY45 promoter amplicons were then cloned into the pUC19_Y vectorCitation71 at the SacI and SmaI restriction sites. The whole cassettes containing the specific gene promoters and the GUS reporter gene were then isolated by restriction digestion with SacI and SalI and cloned into the pCAMBIA2300 binary vector.Citation67 The identity, orientation, and junctions of the resulting pCAM–WRKY3GUS and pCAM–WRKY35GUS constructs were confirmed by digestion patterns. The pCAMBIA2300 empty-vector control and both pCAM–WRKY3GUS and pCAM–WRKY35GUS constructs were subsequently used for R. rhizogenes-mediated transformation as described below. For the overexpression of SlWRKY3, the coding sequence was amplified from RNA of 1-month-old tomato plants with specific primers by the addition of KpnI in the forward primer (OEWRKY3Fp1: 5’- tatgGGTACCATGGGGGAAACAGGGG-3) and HindIII in the reverse primer (OEWRKY3 Rp1:5’- AGAAGCTTTTAGCTGTATTTATTCCTTGTTC TTTTTTGCATTTGA-3’) as well. The full-length tomato WRKY3 gene (1383 bp) was cloned into the pHANNIBAL vector with Kpnl and HindIII enzymes. The 3538-bp fragment, containing the CaMV 35S promoter, WRKY3 coding sequence and OCS terminator, was digested with NotI enzyme that was further subcloned into the binary vector (pART27 vector).Citation27 The orientation and junction of the resulting pART27+ OEWRKY3 construct were confirmed by restriction digestion. Empty pART27 and pART27+ OEWRKY3 constructed plasmids were then used for R. rhizogenes-mediated transformation as described below. To build the RNAi construct, the 571-bp coding sequence of SlWRKY3 was amplified through specific primers prepared to generate the XhoI–HindIII and KpnI–ClaI sites in forward WRKY3RNAi Fp3 (ccCCtcGAgCAAgCttCCAAACGAAGAAAA) and reverse WRKY3RNAi Rp3 (GGccGGTACCTTAGaTcgATTTATTCCTTGTTCCTC). The purified products were then cloned in sense and antisense directions at the XhoI–KpnI and HindIII–ClaI sites, respectively, in pHANNIBAL containing the CaMV 35S promoterCitation27 to create hairpin dsRNA of SlWRKY3 in transformed tomato roots. Subsequently, the NotI fragment of pHANNIBAL was cloned into a binary vector (pART27) and the orientation and junction of the resulting pART27+ RNAiWRKY were confirmed by digestion. The whole construct was transformed into tomato roots via R. rhizogenes-mediated transformation.
R. rhizogenes-mediated root transformation and production of hairy root cultures
The binary vectors pCAM–WRKY35 GUS, pCAM–WRKY3 GUS, pART27+ OEWKRY3, and pART27+ RNAiWRKY3, along with the empty vector controls pCAMBIA and pART27, were electrotransferred into R. rhizogenes ATCC 15834.Citation72 Aseptically grown individual cotyledons were excised from 15- to 20-day-old tomato seedlings and immersed in 2-day-old R. rhizogenes suspension for 2 h at 28°C. The excess R. rhizogenes was removed from the excised cotyledons by sterile paper towel and immediately placed on standard strength Gamborg’s medium for 4 days of co-cultivation. Then, cotyledons were transferred to B5 media containing 50 mg/mL kanamycin (Duchefa Biochemie) and 300 mg/mL timentin (ticarcillin disodium/potassium clavulanate 15:1) (Duchefa Biochemie) antibiotics. All of the plates were incubated at 25°C in the dark; within 7 to 10 days, roots started to emerge from the punctured surface of the cotyledons. Hairy roots were excised from the cotyledon and transferred to Gamborg’s medium (Duchefa) containing 0.8% Gelrite and 50 mg/mL kanamycin. For the M. javanica-infection experiment, well-developed transformed roots were subcultured in antibiotic-free Gamborg’s media for 2 weeks and then 300 sterile M. javanica were used to infect the transgenic root lines. The samples were collected for GUS-staining and infection assessments at the designated time points. The presence and expression of WRKY3-overexpressing and RNAi-WRKY3 transgenes were confirmed with kanamycin primers (see Table S1) and qRT-PCR using total RNA isolated from transformed and nontransformed root tissues. For the qRT-PCR analysis, the total RNA was isolated at 5 dai from control and overexpressing lines using InviTrap Spin Plant Mini Kit (Gene JET Plant RNA purification Mini Kit, Thermo Scientific, Vilnius, Lithuania). QRT-PCR was carried out with the primer set WRKY3 Real Fp and WRKY3 Real Rp (Table S1).
Histochemical localization of GUS activity and microscopic analysis
Transformed root lines (promoter–GUS; 15 days old) were infected with M. javanica as described above, and GUS activity was determined at the selected time points. In the promoter–GUS experiment, the noninfected plants served as controls. Both infected and noninfected transgenic roots were collected from Gamborg’s media at the specific time points and infiltrated with GUS buffer (50 mM sodium phosphate pH 7.0, 10 mM EDTA, 5 mM K4[Fe2(CN)6], 5 mM K3[Fe2(CN)6], 0.2% v/v Triton X-100, and 2 mM 5-bromo-4-chloro-3-indolyl β-D-glucuronide) for 12 h at 37ºC. All of the roots were then washed twice with distilled water. At each time point, at least 15 infected transgenic roots were checked for GUS expression. To ensure the cellular localization of GUS expression in giant cells, thin sections were taken and studied microscopically as described previously.Citation73 For the histological sectioning, GUS-stained roots were dehydrated in 0.25% (w/v) glutaraldehyde and 4% (v/v) paraformaldehyde in 50 mM PBS pH 7.2, and then embedded in Technovit 7100 (Heraeus Kulzer, Wehrheim, Germany) as described by the manufacturer. All of the embedded root tissues were sectioned (3 μm) and mounted posteriorly in Depex (Sigma-Aldrich). For microscopic observation, GUS-stained root sections mounted on microscope slides and morphologically distinguished semi-thin sections were photographed with a Nikon DS Ri2 equipped with dark-field illumination (Leica Microsystems GmbH, Wetzlar, Germany; Nikon Corporation, Tokyo, Japan). The stereomicroscope (Leica MZFLIII, Leica Microsystems GmbH) equipped with a Nikon DS-Fi1 camera was used to captured 15 and 28 dai GUS-stained root samples.
Hormone exogenous treatment
To determine the effects of exogenous phytohormone application on SlWRKY3 and SlWRKY35 promoter–GUS expression in the transformed root lines, 1-week-old roots of each respective line from phytohormone free root culture were subcultured in a Gamborg’s medium plate containing SA (1 or 5 mM), IBA (1 or 10 µM), BA (0.1 or 0.5 µM), IAA (1 or 5 µM), JA (10 or 20 µm), or MeJA (0.01 or 0.1 mM) for 16 h following monitoring GUS expression as described previously.Citation24,Citation25 Seven-day-old transformed roots were subcultured in plain Gamborg’s medium and used as a control. All of the root tissues were carefully monitored for GUS expression with a Nikon DS Ri2.
Evaluation of tomato root response to nematode infection
To determine nematode infection in control, overexpressed and RNAi root lines, root samples were harvested from monoxenic culture at 15 and 28 dai. All root samples were stained overnight with acid fuchsin (Sigma-Aldrich) (17.5 mg acid fuchsin, 500 mL ethanol, and 500 mL acetic acid). Stained root samples were destained with three water rinses and stored for further analysis.Citation74 The root samples were mounted in tap water and galls were opened under a stereomicroscope (Olympus SZX12, Tokyo, Japan). For the analysis of nematode development, the number of J2s, J3/J4s, mature females, and egg masses were counted at 15 and 28 dai. Mean values of infective J2s, J3/J4s, females, and egg masses were collected by analyzing 10 replicates per line. The infection experiments were repeated twice with similar results, and results of only one individual experiment are presented here. Data were evaluated for statistical significance and the differences were determined for every experiment by comparing all means, using Tukey–Kramer test with an alpha level of 0.05 by JMP software (SAS Institute, Cary, NC).
Genomic DNA and cDNA isolation
Genomic DNA was isolated from 1-month-old soil-grown tomato cv. Avigail 870 seedlings by the cetyltrimethylammonium bromide method as described by Goetz et al.Citation75 To obtain the full-length SlWRKY3 cDNA, tomato roots and leaves were homogenized in liquid nitrogen, and total RNA was extracted with the GeneJET Plant RNA Purification Mini Kit (Thermo Scientific). To remove contaminating genomic DNA, the RNA samples were incubated in the presence of 10 units of TURBO DNA-free DNASE (Applied Biosystems, Foster City, CA), and the DNA-free RNA was converted into first-strand cDNA with the Verso cDNA Kit (Thermo Scientific).
Quantitative PCR analysis
Gene transcripts were quantified by real-time RT-PCR on total RNA extracted from noninoculated and inoculated root lines at 5 dai. Five root systems (200 mg) were pooled for the corresponding time point while noninfected roots were used as controls and RNA was extracted as described above. QRT-PCR was performed using the SYBR-Green ROX Mix (ABgene, Epsom, UK) and all primers used for gene quantification (Table S1) were designed using PrimerExpress software (Applied Biosystems). The subsequent real-time PCR contained 3.4 µL cDNA in a total volume of 10 µL, consisting of SYBR-Green ROX Mix and 150 nM forward primer and 150 nM reverse primer in real-time PCR plasticware (Axygen, Union City, CA). All PCR cycles began with 2 min at 50°C then 10 min at 95°C, followed by 40 two-step cycles comprising 10 s at 95°C and 1 min at 60°C.
After PCR, a melting curve was generated by gradually increasing the temperature to 95°C to test for amplicon specificity. For qPCR, a mixture of all cDNAs was used for all treatments, as a template for calibration curves designed for each pair of primers. Each reaction was performed in triplicate and the results represent the means of two independent biological experiments. Three constitutively expressed genes – actin (GenBank accession no. U60482.1), β-tubulin (GenBank accession no. NM_001247878.1), and 18S (GenBank accession no. BH012957.1) – were the endogenous controls for tomato gene-expression analyses (Table S1). Transcript levels were normalized for each sample with the geometric mean of the corresponding selected housekeeping genes. All of the housekeeping genes were confirmed to display minimal variation across the treatments and were the most stable housekeeping genes from a set of tested genes in a given cDNA sample.Citation76 Values were expressed as increase or decrease in level relative to the calibration sample. The control reactions were: PCR negative control without cDNA template to confirm that there were no nonspecific PCR products, and a second reaction containing cDNA that had not been subjected to reverse transcriptase reaction (NRT control). Statistical differences between treatments and/or root lines were calculated by LSD, according to Tukey–Kramer multiple comparison test at p ≤ 0.05. For confirmation of all qRT-PCR results, expression of the subset of genes was analyzed in another two independent experiments, producing the same results. Relative expression for each treatment was calculated in relation to pControl noninoculated roots.
Oxylipin and phytohormone extraction and analysis by LC–MS(/MS)
Our comprehensive platform allowed the quantitative evaluation of approximately 100 oxylipins down to low nanomolar levels. The analytical conditions were modified from existing methods to define the best conditions for the quantitation of oxylipins in the biological matrix of the present study.Citation77 We used the quantitative profiling method for oxylipin metabolome by LC–electrospray ionization (ESI) tandem MS.Citation77 Quantification was performed with authentic standards, where the transition of each compound was monitored on calibration curves with at least seven points. The lowest and highest concentration points of the calibration curves of the individual compounds were 0.097–0.197 and 25–50 µM, respectively. The calibration curves were drawn by plotting the ratio between the area of the target compound and that of the IS (internal standard) deuterated 9-hydroxy-10E,12Z-octadecadienoic acid (9-HODEd4) against the above concentrations. To test the validity of the method, measurements were performed using different aliquots of inoculated and noninoculated tomato roots. Fatty acids were extracted from transgenic hairy roots infected or not infected with the RKN M. javanica, as described by Scala et al.Citation78 with some modifications. The internal reference standard added for the quantitative analysis was 9-HODEd4 (MW 300.5 g/mol) at a final concentration of 2 µM. Briefly, 20 mg of lyophilized matrix was vortex-mixed with 1 mL of extraction mixture (Hex:iPrOH, 3:2 v/v, with 0.0025% w/v of butylated hydroxytoluene). The clear upper phase was collected and added to 0.5 mL of a saline solution of 6.7% (w/v) potassium sulfate. The Hex upper layer was transferred into a clear tube and dried under N2, reconstituted in 200 µL of MeOH, and loaded into the HPLC injector for oxylipin quantitative analysis. The concentration of oxylipins in the final volume of 200 µL was reported in micromolar. The equipment, chromatographic column, and analytical software were all from Agilent Technologies (Santa Clara, CA). Samples were analyzed by LC (HPLC 1200 series rapid resolution) coupled to a triple quadrupole MS (G6410A series triple quadrupole, QqQ) equipped with an ESI source. Acquisition was in negative-ion mode. Chromatographic separation was performed with a Zorbax ECLIPSE XDB-C18 rapid resolution HT 4.6 × 50 mm 1.8 µm p.s. column. The mobile phases consisted of A: water/ACN (Acetonitrile) 97:3 v/v containing 0.1% (v/v) HCOOH, and B: ACN/iPrOH 90:10 v/v. The elution program was as follows: 0–2 min 20% B, 2–4 min 35% B, 4–6 min 40% B, 6–7 min 42% B, 7–9 min 48% B, 9–15 min 65% B, 15–17 min 75% B, 17–18.5 min 85% B, 18.5–19.5 min 95% B, 19.5–24 min 95% B, 24–26 min 99% B, 26–30 min 99% B, and 30–34 min 20% B. The flow rate was as follows: 0–24 min at 0.6 mL/min, 24–30 min at 1 mL/min, and 30–34 min at 0.6 mL/min. The column thermostat was set to 40°C. The injection volume was 10 µL. The injector needle was washed with the mobile phase in the wash port at the end of each HPLC run. Nitrogen was used as the nebulizing and desolvation gas. The temperature and flow of the drying gas were 350°C and 10 L/min, respectively, with a nebulization pressure of 20 psi. The capillary and cone voltage was 4000 V. All data were statistically evaluated with GraphPad Prism 5.0 (GraphPad Software, Inc., La Jolla, CA). Results of the oxylipin quantification from different matrices are given as the mean (±SE) of six replicates from two independent experiments. Means were compared by Mann–Whitney test. For tissue-specific and RKN-induced analysis of JA and OPDA levels, inoculated and noninoculated tomato hairy roots were harvested and immediately frozen in liquid N2. Four biological replicates (three roots per replicate) were analyzed. JA and OPDA were analyzed using an Ascentis Express 18 column (3 cm × 2.1 mm, 2.7 µm), together with a next-generation machine API 3200 LC/MS/MS in negative-phase mode, according to the methods outlined by Christensen. Citation79 For the JA internal standard, 15 pmol of deuterated JA was used (https://www.cdnisotopes.com cat3 D-6936). The organic phase was dried under continuous nitrogen and resuspended in 150 µL of methanol. Levels of 12-OPDA in the control and overexpressing roots were measured as described by Stumpe et al.Citation80 Each treatment was replicated four times, and the data were expressed as the means of four replicates.
Disclosure of Potential Conflicts of Interest
No potential conflicts of interest were disclosed.
Supplemental Material
Download MS Power Point (36.8 KB)Acknowledgments
The current study was supported by US-ISRAEL Ninational Agricultural Research and Development Fund (BARD) project no. IS-4727-1
Supplemental material
Supplemental Material footnote should be located in the CFN.
Additional information
Funding
References
- Favery B, Quentin M, Jaubert-Possamai S, Abad P. Gall-forming root-knot nematodes hijack key plant cellular functions to induce multinucleate and hypertrophied feeding cells. J Insect Physiol. 2016;84:60–69. doi:10.1016/j.jinsphys.2015.07.013.
- de Almeida Engler J, Engler G. G. G. Unravelling the plant cell cycle in nematode induced feeding sites. In: Jones J, Gheysen G, F C, editors. Genomics and molecular genetics of plant-nematode interactions. Dordrecht: Springer Science+Business Media; 2011. p. 349–368.
- Kyndt T, Vieira P, Gheysen G, de Almeida-Engler J. Nematode feeding sites: unique organs in plant roots. Planta. 2013;238:807–818. doi:10.1007/s00425-013-1923-z.
- Gheysen G, Fenoll C. C. F. Gene expression in nematode feeding sites. Annu Rev Phytopathol. 2002;40:191–219. doi:10.1146/annurev.phyto.40.121201.093719.
- Goto M, Amino H, Nakajima M, Tsuji N, Sakamoto K, Kita K. Cloning and characterization of hypoxia-inducible factor-1 subunits from ascaris suum—A parasitic nematode highly adapted to changes of oxygen conditions during its life cycle. Gene. 2013;516:39–47. doi:10.1016/j.gene.2012.12.025.
- Goverse A, Smant G. The activation and suppression of plant innate immunity by parasitic nematodes. Annu Rev Phytopathol. 2014;52:243–265. doi:10.1146/annurev-phyto-102313-050118.
- Li J, Zou C, Xu J, Ji X, Niu X, Yang J, Huang X, Zhang K-Q. Molecular mechanisms of nematode-nematophagous microbe interactions: basis for biological control of plant-parasitic nematodes. Annu Rev Phytopathol. 2015;53:67–95. doi:10.1146/annurev-phyto-080614-120336.
- Agarwal P, Reddy MP, Chikara J. WRKY: its structure, evolutionary relationship, DNA-binding selectivity, role in stress tolerance and development of plants. Mol Biol Rep. 2011;38:3883–3896. doi:10.1007/s11033-010-0504-5.
- Huang SX, Gao YF, Liu JK, Peng XL, Niu XL, Fei ZJ, Cao S, Liu Y. Genome-wide analysis of WRKY transcription factors in solanum lycopersicum. Mol Genet Genomics. 2012;287:495–513. doi:10.1007/s00438-012-0696-6.
- Pape S, Thurow C, Gatz C. The Arabidopsis PR-1 promoter contains multiple integration sites for the coactivator NPR1 and the repressor SNI1. Plant Physiol. 2010;154:1805–1818. doi:10.1104/pp.110.165563.
- Rushton PJ, Somssich IE, Ringler P, Shen QJ. WRKY transcription factors. Trends Plant Sci. 2010;15:247–258. doi:10.1016/j.tplants.2010.02.006.
- Eulgem T, Rushton PJ, Robatzek S, Somssich IE. The WRKY superfamily of plant transcription factors. Trends Plant Sci. 2000;5:199–206.
- Ross CA, Liu Y, Shen QJ. The WRKY gene family in rice (Oryza sativa). J Integr Plant Biol. 2007;49:827–842. doi:10.1111/jipb.2007.49.issue-6.
- Sun C, Palmqvist S, Olsson H, Boren M, Ahlandsberg S, Jansson C. A novel WRKY transcription factor, SUSIBA2, participates in sugar signaling in barley by binding to the sugar-responsive elements of the iso1 promoter. Plant Cell. 2003;15:2076–2092.
- Eulgem T. Dissecting the WRKY web of plant defense regulators. PLoS Pathog. 2006;2:e126. doi:10.1371/journal.ppat.0020126.
- Kyndt T, Denil S, Haegeman A, Trooskens G, Bauters L, Van Criekinge W, De Meyer T, Gheysen G. Transcriptional reprogramming by root knot and migratory nematode infection in rice. New Phytol. 2012;196:887–900. doi:10.1111/j.1469-8137.2012.04311.x.
- Nguyễn PV, Bellafiore S, Petitot A-S, Haidar R, Bak A, Abed A, Gantet P, Mezzalira I, de Almeida Engler J, Fernandez D. Meloidogyne incognita-rice (Oryza sativa) interaction: a new model system to study plant-root-knot nematode interactions in monocotyledons. Rice. 2014;7:23. doi:10.1186/s12284-014-0023-4.
- Oh SK, Yi SY, Yu SH, Moon JS, Park JM, Choi D. CaWRKY2, a chili pepper transcription factor, is rapidly induced by incompatible plant pathogens. Mol Cells. 2006;22:58–64.
- Yang Y, Zhou Y, Chi Y, Fan B, Chen Z. Characterization of soybean WRKY gene family and identification of soybean WRKY genes that promote resistance to soybean cyst nematode. Sci Rep-Uk. 2017;7:17804. doi:10.1038/s41598-017-18235-8.
- Grunewald W, Karimi M, Wieczorek K, Van de Cappelle E, Wischnitzki E, Grundler F, Inzé D, Beeckman T, Gheysen G. A role for AtWRKY23 in feeding site establishment of plant-parasitic nematodes. Plant Physiol. 2008;148:358–368. doi:10.1104/pp.108.119131.
- Ali MA, Wieczorek K, Kreil DP, Bohlmann H, Jones J. The beet cyst nematode Heterodera schachtii modulates the expression of WRKY transcription factors in syncytia to favour its development in Arabidopsis roots. PLoS One. 2014;9:e102360. doi:10.1371/journal.pone.0102360.
- Bhattarai KK, Atamian HS, Kaloshian I, Eulgem T. WRKY72‐type transcription factors contribute to basal immunity in tomato and Arabidopsis as well as gene‐for‐gene resistance mediated by the tomato R gene Mi‐1. Plant J. 2010;63:229–240. doi:10.1111/j.1365-313X.2010.04232.x.
- Atamian HS, Eulgem T, Kaloshian I. SlWRKY70 is required for Mi-1-mediated resistance to aphids and nematodes in tomato. Planta. 2012;235:299–309. doi:10.1007/s00425-011-1509-6.
- Iberkleid I, Sela N, Brown Miyara S. Meloidogyne javanica fatty acid- and retinol-binding protein (Mj-FAR-1) regulates expression of lipid-, cell wall-, stress- and phenylpropanoid-related genes during nematode infection of tomato. BMC Genomics. 2015;16:272. doi:10.1186/s12864-015-1426-3.
- Chinnapandi B, Bucki P, Braun Miyara S. SlWRKY45, nematode-responsive tomato WRKY gene, enhances susceptibility to the root knot nematode; M. Javanica Infect. Plant Signaling Behav. 2017;12:e1356530. doi:10.1080/15592324.2017.1356530.
- Shah SJ, Anjam MS, Mendy B, Anwer MA, Habash SS, Lozano-Torres JL, Grundler FMW, Siddique S. Damage-associated responses of the host contribute to defence against cyst nematodes but not root-knot nematodes. J Exp Bot. 2017;68:5949–5960. doi:10.1093/jxb/erx374.
- Wesley SV, Helliwell CA, Smith NA, Wang M, Rouse DT, Liu Q, Gooding PS, Singh SP, Abbott D, Stoutjesdijk PA, et al. Construct design for efficient, effective and high‐throughput gene silencing in plants. Plant J. 2001;27:581–590.
- Kachroo A, Kachroo P. Fatty Acid-derived signals in plant defense. Annu Rev Phytopathol. 2009;47:153–176. doi:10.1146/annurev-phyto-080508-081820.
- Ali MA, Wieczorek K, Kreil DP, Bohlmann H. The beet cyst nematode heterodera schachtii modulates the expression of WRKY transcription factors in syncytia to favour its development in arabidopsis roots. PLoS One. 2014;9.
- Ulker B, Somssich IE. WRKY transcription factors: from DNA binding towards biological function. Curr Opin Plant Biol. 2004;7:491–498. doi:10.1016/j.pbi.2004.07.012.
- Liu J, Chen X, Liang X, Zhou X, Yang F, Liu J, He SY, Guo Z. Alternative splicing of rice WRKY62 and WRKY76 transcription factor genes in pathogen defense. Plant Physiol. 2016: 01921.2015.
- Mao G, Meng X, Liu Y, Zheng Z, Chen Z, Zhang S. Phosphorylation of a WRKY transcription factor by two pathogen-responsive MAPKs drives phytoalexin biosynthesis in Arabidopsis. Plant Cell. 2011;23:1639–1653. doi:10.1105/tpc.111.084996.
- Bird AF. The inducement of giant cells by Meloidogyne javanica. Nematologica. 1962;8:1–10. doi:10.1163/187529262X00927.
- Giebel J. Biochemical mechanisms of plant resistance to nematodes: a review. J Nematol. 1974;6:175.
- Strader LC, Bartel B. Transport and metabolism of the endogenous auxin precursor indole-3-butyric acid. Mol Plant. 2011;4:477–486. doi:10.1093/mp/ssr006.
- Richardson L, Price N. Observations on the biology of Meloidogyne incognita and the diageotropica tomato mutant. Revue de nématologie. 1984;7:97–99.
- Karczmarek A, Overmars H, Helder J, Goverse A. Feeding cell development by cyst and root-knot nematodes involves a similar early, local and transient activation of a specific auxin-inducible promoter element. Mol Plant Pathol. 2004;5:343–346. doi:10.1111/j.1364-3703.2004.00230.x.
- Mazarei M, Lennon KA, Puthoff DP, Rodermel SR, Baum TJ. Expression of an Arabidopsis phosphoglycerate mutase homologue is localized to apical meristems, regulated by hormones, and induced by sedentary plant-parasitic nematodes. Plant Mol Biol. 2003;53:513–530. doi:10.1023/B:PLAN.0000019062.80459.80.
- Bostock RM. Signal crosstalk and induced resistance: straddling the line between cost and benefit. Annu Rev Phytopathol. 2005;43:545–580. doi:10.1146/annurev.phyto.41.052002.095505.
- Durrant WE, Dong X. Systemic acquired resistance. Annu Rev Phytopathol. 2004;42:185–209. doi:10.1146/annurev.phyto.42.040803.140421.
- Schluttenhofer C, Yuan L. Regulation of specialized metabolism by WRKY transcription factors. Plant Physiol. 2015;167:295–306. doi: 10.1104/pp.114.251769.
- Ishiguro S, Nakamura K. Characterization of a cDNA encoding a novel DNA-binding protein, SPF1, that recognizes SP8 sequences in the 5′ upstream regions of genes coding for sporamin and β-amylase from sweet potato. Mol Genl Genet MGG. 1994;244:563–571.
- Su T, Xu Q, Zhang FC, Chen Y, Li LQ, Wu WH, Chen YF. WRKY42 modulates phosphate homeostasis through regulating phosphate translocation and acquisition in Arabidopsis. Plant Physiol. 2015;167:1579–1591.
- Wang H, Avci U, Nakashima J, Hahn MG, Chen F, Dixon RA. Mutation of WRKY transcription factors initiates pith secondary wall formation and increases stem biomass in dicotyledonous plants. Proc Nat Acad Sci. 2010; 107:22338–22343. doi: 10.1073/pnas.1016436107.
- Schmutz J, Cannon SB, Schlueter J, Ma J, Mitros T, Nelson W, Hyten DL, Song Q, Thelen JJ, Cheng J, Xu D. Genome sequence of the palaeopolyploid soybean. Nature. 2010;463:178. doi:10.1038/nature08700.
- Sticher L, Mauch-Mani B, Métraux JP. Systemic acquired resistance. Annu Rev Phytopathol. 1997;35:235–270. doi:10.1146/annurev.phyto.35.1.235.
- Branch C, Hwang C-F, Navarre DA, Williamson VM. Salicylic acid is part of the Mi-1-mediated defense response to root-knot nematode in tomato. Molecular Plant-Microb Interact. 2004;17:351–356. doi:10.1094/MPMI.2004.17.4.351.
- Bhattarai KK, Xie QG, Mantelin S, Bishnoi U, Girke T, Navarre DA, Kaloshian I. Tomato susceptibility to root-knot nematodes requires an intact jasmonic acid signaling pathway. Mol Plant Microb In. 2008;21:1205–1214. doi:10.1094/MPMI-21-9-1205.
- Wubben MJ, Jin J, Baum TJ. Cyst nematode parasitism of Arabidopsis thaliana is inhibited by salicylic acid (SA) and elicits uncoupled SA-independent pathogenesis-related gene expression in roots. Mol Plant Microbe Interact. 2008;21:424–432. doi:10.1094/MPMI-21-4-0424.
- Molinari S. Salicylic acid as an elicitor of resistance to root-knot nematodes in tomato. XV Meeting of the EUCARPIA Tomato Working Group. 2005;789:119–126.
- Owen K, Green C, Deverall B. A benzothiadiazole applied to foliage reduces development and egg deposition by Meloidogyne spp. in glasshouse-grown grapevine roots. Australas Plant Pathol. 2002;31:47–53. doi:10.1071/AP01068.
- Nandi B, Kundu K, Banerjee N, Babu SPS. Salicylic acid-induced suppression of Meloidogyne incognita infestation of okra and cowpea. Nematol. 2003;5:747–752. doi:10.1163/156854103322746922.
- Borrego EJ, Kolomiets MV. Synthesis and functions of jasmonates in maize. Plants. 2016;5:41. doi:10.3390/plants5040041.
- Howe GA, Schilmiller AL. Oxylipin metabolism in response to stress. Curr Opin Plant Biol. 2002;5:230–236.
- Creelman RA, Mulpuri R, Rao Mulpuri. The oxylipin pathway in Arabidopsis. The Arabidopsis book. 2002. p. e0012.
- Feussner I, Wasternack C. The lipoxygenase pathway. Annu Rev Plant Biol. 2002;53:275–297. doi:10.1146/annurev.arplant.53.100301.135248.
- Hanano A, Burcklen M, Flenet M, Ivancich A, Louwagie M, Garin J, Blée E. Plant seed peroxygenase is an original heme-oxygenase with an EF-hand calcium binding motif. J Biol Chem. 2006;281:33140–33151. doi:10.1074/jbc.M605395200.
- Meesapyodsuk D, Qiu X. A peroxygenase pathway involved in the biosynthesis of epoxy fatty acids in oat. Plant Physiol. 2011;157:454–463. doi:10.1104/pp.111.178822.
- Greene JF, Newman JW, Williamson KC, Hammock BD. Toxicity of epoxy fatty acids and related compounds to cells expressing human soluble epoxide hydrolase. Chem Res Toxicol. 2000;13:217–226.
- Vellosillo T, Martinez M, Lopez MA, Vicente J, Cascon T, Dolan L, Hamberg M, Castresana C. Oxylipins produced by the 9-lipoxygenase pathway in Arabidopsis regulate lateral root development and defense responses through a specific signaling cascade. Plant Cell. 2007;19:831–846. doi:10.1105/tpc.106.046052.
- Enns MW, Cox BJ. Psychosocial and clinical predictors of symptom persistence vs remission in major depressive disorder. Can Journal Psychiatry Revue Canadienne De Psychiatrie. 2005;50:769–777. doi:10.1177/070674370505001206.
- Micheli F. Pectin methylesterases: cell wall enzymes with important roles in plant physiology. Trends Plant Sci. 2001;6:414–419.
- Mur LA, Kenton P, Atzorn R, Miersch O, Wasternack C. The outcomes of concentration-specific interactions between salicylate and jasmonate signaling include synergy, antagonism, and oxidative stress leading to cell death. Plant Physiol. 2006;140:249–262. doi:10.1104/pp.105.072348.
- De Medeiros HA, de Araújo Filho JV, De Freitas LG, Castillo P, Rubio MB, Hermosa R, Monte E. Tomato progeny inherit resistance to the nematode Meloidogyne javanica linked to plant growth induced by the biocontrol fungus Trichoderma atroviride. Sci Rep. 2017;7:40216. doi:10.1038/srep40216.
- Pan C, Ye L, Qin L, Liu X, He Y, Wang J, Chen L, Lu G. CRISPR/Cas9-mediated efficient and heritable targeted mutagenesis in tomato plants in the first and later generations. Sci Rep-Uk. 2016;6:24765. doi:10.1038/srep24765.
- Bailey TL, Williams N, Misleh C, Li WW. MEME: discovering and analyzing DNA and protein sequence motifs. Nucleic Acids Res. 2006;34:W369–W73. doi:10.1093/nar/gkl198.
- Remy S, Thiry E, Coemans B, Windelinckx S, Swennen R, Sági L. Improved T-DNA vector for tagging plant promoters via high-throughput luciferase screening. BioTech. 2005;38:763–770. doi:10.2144/05385RR01.
- Hussey RS, Baker KR. Comparison of methods of collecting inocula for Meloidogyne spp., including a new technique. Plant Dis Rep. 1973;57:1025–1028.
- Van Vuuren RJ, Woodward B. The response of cassava cultivars to root-knot nematode infestation: an in vitro method. Euphytica. 2001;120:109–113. doi:10.1023/A:1017524210671.
- Sijmons PC, Grundler FM, Mende N, Burrows PR, Wyss U. Arabidopsis thaliana as a new model host for plant‐parasitic nematodes. Plant J. 1991;1:245–254. doi:10.1111/j.1365-313X.1991.00245.x.
- Vancanneyt G, Schmidt R, O‘Connor-Sanchez A, Willmitzer L, Rocha-Sosa M. Construction of an intron-containing marker gene: splicing of the intron in transgenic plants and its use in monitoring early events in Agrobacterium-mediated plant transformation. Mol Genl Genet MGG. 1990;220:245–250.
- Shen WJ, Forde BG. Efflicient transformation of Agrobactenum spp. by high voltage electroporation. Nucleic Acid Res. 1989;17:8385. doi:10.1093/nar/17.20.8385.
- Mitchum MG, Sukno S, Wang X, Shani Z, Tsabary G, Shoseyov O, Davis EL. The promoter of the Arabidopsis thaliana Cel1 endo‐1, 4‐β glucanase gene is differentially expressed in plant feeding cells induced by root‐knot and cyst nematodes. Mol Plant Pathol. 2004;5:175–181. doi:10.1111/j.1364-3703.2004.00216.x.
- Bybd DW, Kirkpatrick T, Barker KR. An improved technique for clearing and staining plant tissues for detection of nematodes. J Nematol. 1983;15:142–143.
- Goetz M, Godt DE, Guivarc‘H A, Kahmann U, Chriqui D, Roitsch T Induction of male sterility in plants by metabolic engineering of the carbohydrate supply. Proc Nat Acad Sci. 2001; 98:6522–6527. doi: 10.1073/pnas.091097998.
- Vandesompele J, De Preter K, Pattyn F, Poppe B, Van Roy N, De Paepe A, Speleman F. Accurate normalization of real-time quantitative RT-PCR data by geometric averaging of multiple internal control genes. Genome Biol. 2002;3(research0034):1. doi:10.1186/gb-2002-3-7-research0034.
- Yang J, Schmelzer K, Georgi K, Hammock BD. Quantitative profiling method for oxylipin metabolome by liquid chromatography electrospray ionization tandem mass spectrometry. Anal Chem. 2009;81:8085–8093. doi:10.1021/ac901282n.
- Scala A, Allmann S, Mirabella R, Haring MA, Schuurink RC. Green leaf volatiles: a plant’s multifunctional weapon against herbivores and pathogens. Int J Mol Sci. 2013;14:17781–17811. doi:10.3390/ijms140917781.
- Christensen SA, Nemchenko A, Borrego E, Murray I, Sobhy IS, Bosak L, DeBlasio S, Erb M, Robert CAM, Vaughn KA, et al. The maize lipoxygenase, Zm LOX 10, mediates green leaf volatile, jasmonate and herbivore‐induced plant volatile production for defense against insect attack. Plant J. 2013;74:59–73. doi:10.1111/tpj.12101.
- Stumpe M, Carsjens JG, Stenzel I, Gobel C, Lang I, Pawlowski K, Hause B, Feussner I. Lipid metabolism in arbuscular mycorrhizal roots of medicago truncatula. Phytochemistry. 2005;66:781–791. doi:10.1016/j.phytochem.2005.01.020.