ABSTRACT
Height and leaf morphology are important agronomic traits of the major crop plant rice (Oryza sativa). In previous studies, the dwarf and narrow leaf genes (dnl1, dnl2 and dnl3) have identified in rice. Using the Ac/Ds knockout system, we found a new dwarf and narrow leaf (dnl) mutant and identified mutated gene. The dnl-4 mutant showed reduced plant height and leaf blade width compared to the wild type, and increased leaf inclination. The morphological defects of the mutant were caused by the suppressed expression of the DNL-4 gene, which encodes a pfkB carbohydrate kinase protein. These results suggest that DNL-4 expression is involved in modulating plant height and leaf growth. Furthermore, DNL-4 expression also affects productivity in rice: the dnl-4 mutant exhibited reduced panicle length and grain width compared with the wild type. To understand DNL-4 function in rice, we analyzed the expression levels of leaf growth-related genes, such as NAL1, NAL7, and CSLD4, in the dnl-4 mutant. Expression of NAL1 and NAL7 was downregulated in the dnl-4 mutant compared to the wild type. The observation that DNL-4 expression corresponded with that of NAL1 and NAL7 is consistent with the narrow leaf phenotype of the dnl-4 mutant. These results suggest that DNL-4 regulates plant height and leaf structure in rice.
Introduction
Rice (Oryza sativa) is one of the most important crops in the world. In plants, leaf shape and size are important traits that affect photosynthesis and transpiration and consequently plant growth and yield.Citation1–4 Therefore, the mechanisms underlying leaf development have been extensively studied.Citation5–7 Leaf development is modulated by cellular processes such as cell division and cell expansion, which are regulated by a number of genes in rice.Citation8–11 A rice outcurved leaf1 (oul1) and outermost cell-specific gene5 (Roc5) double knockout mutant showed abaxial leaf rolling and increases in the number and size of bulliform cells.Citation11 Plant height is an important agronomic trait for rice productivity and is influenced by leaf development. Dwarfism is often associated with defects in photosynthesis, cell wall deposition, and metabolism. Previous studies reported that the expression of leaf growth-related genes is associated with plant height and grain yield in rice. For example, NARROW LEAF 1 (NAL1) regulates leaf width and plant height by affecting vein patterning and cell division.Citation12 NARROW LEAF 7 (NAL7) controls leaf shape through its effects on auxin signaling.Citation13 A cellulose synthase‐like D4 (OsCSLD4), which encodes glycosyltransferase family 2 (GT2), affects vein patterning and plant height.Citation14 These indicate the important role of leaf growth-related genes in the plant growth. However, the functional relationship between dwarfism and leaf growth is not clearly understood.
Phosphofructokinase B-type (pfkB) carbohydrate kinases constitute a large protein family present in both prokaryotes and eukaryotes. These kinases, which in eukaryotes are located in the nucleus and cytoplasm, phosphorylate a variety of substrates, including fructose, fructose-6-phosphate, ribose, adenosine, phosphomethylpyrimidine, and tagatose-6-phosphate.Citation15–17 In rice and Arabidopsis thaliana, two genes encoding putative pfkB carbohydrate kinases, fructokinase-like 1 and 2 (FLN1 and FLN2), have been identified and their protein products localized to the chloroplast.Citation18–20 Based on the phenotypes of OsFLN1 and OsFLN2 knockout mutants, these kinases appear to regulate leaf and plant growth by controlling chloroplast biogenesis.Citation19,Citation20 However, the molecular functions of other plant pfkB carbohydrate kinases are poorly understood.
To gain insight into the mechanisms controlling leaf development, we used the Ac/Ds knockout mutant system to generate new loss-of-function rice mutants with leaf defects. The Ac/Ds knockout mutant system was developed by maize geneticists and enables easy identification of genes of interest by PCR and phenotypic analysis.Citation21–23 Using this system, we isolated a dwarf and narrow leaf (dnl) mutant and identified a novel gene, dwarf and narrow leaf4 (DNL-4), involved in plant height and leaf growth in rice. Analysis of the dnl-4 mutant revealed defects in the vascular pattern of the leaf blade. DNL-4 encodes a pfkB-like carbohydrate kinase with unknown function in plants. Based on these findings, we propose that DNL-4 plays important roles in leaf morphogenesis and plant growth in rice. Furthermore, our observations provide insight into the possible involvement of pfkB carbohydrate kinases in modulating plant height and leaf growth.
Materials and methods
Plant growth conditions
Ac/Ds knockout mutant seeds were obtained from the Department of Functional Crop (DFC), National Institute of Crop Science (NICS), Rural Development Administration (RDA), Milyang City, Korea and stored at – 20°C. Oryza sativa L. cv. Dongjin was used as the wild type. Rice seeds were germinated in distilled water and in a growth chamber (28°C, light/dark cycle of 16/8 h). After 3 days of germination, plants were transferred to soil and grown in greenhouse conditions. To analyze the mature plant morphology, dnl-4 knockout and wild-type plants were grown in a paddy field located at Kyungpook National University, Korea (128:34E/36:15 N).
Mutant genotyping and inverse PCR
Genomic DNA was extracted from the leaves of wild-type and dnl-4 mutant plants using CTAB (Sigma Aldrich, USA) as previously described with slight modification.Citation24 To isolate a homozygous mutant, a T-DNA right border primer (Ds-P1) and two gene-specific primers (P-F, P-R) were used.
To obtain flanking sequences, inverse PCR (IPCR)Citation25 was performed using 0.5 μg genomic DNA isolated leaves from the mutant plants. Genomic DNA was then digested with NlaIII (NEB) and the digested DNA was self-ligated by T4 DNA ligase (NEB) at 16°C. In the first IPCR, the ligated DNA was used as template and Ds 5–1 and Ds 5I-1 primers were used. In the second IPCR, the diluted first IPCR product (1:50) was used as template with the Ds 5I-3 and Ds 5I-4536 primers. Primer sequences are listed in Table S1.
RNA extraction and reverse transcription-quantitative polymerase chain reaction (RT-qPCR)
RT-qPCR analysis was performed using total RNA extracted from the indicated plants using TRIzol (Invitrogen) as previously described with slight modification.Citation26 For cDNA synthesis, 25 µL reactions were used containing 1 µg of total RNA and Tetro cDNA synthesis kit (Bioline). For quantitative PCR, a CFX384 Real-time PCR Detection machine (Bio-Rad) with 2X SYBR Premix Ex taq II (Takara) was used. PCR conditions were programmed according to the manufacturer’s instructions (initial denaturation at 95°C for 5 min followed by 45 cycles of denaturation at 95°C for 10 sec, annealing at 60°C for 10 sec, and extension at 72°C for 10 sec). OsActin1 (Os03g0718100) was used as an internal control. Primer sequences are listed in Table S1.
Phylogenetic analysis
Homologous sequences of DNL-4 were obtained by conducting a National Center for Biotechnology Information BLAST search. Based on the amino acid sequences, phylogenetic trees were constructed using ETE3 v3.0.0b32, implemented in GenomeNet (https://www.genome.jp/tools/ete/).
Cross section and microscopy
Leaf blades were fixed in FAA (45% ethanol, 2.5% formaldehyde, 2.5% acetic acid, 50% distilled water), dehydrated through an ethanol series (50%, 60%, 70%, 80%, 90%, 95%, 99%) at 4°C for 20 minutes at each concentration, and then embedded in Technovit 7100 resin (Heraeus Kulzer).Citation27 Samples were sectioned into 4 µm thick slices using a rotary microtome MICROM HM325 (Thermo Fisher Scientific) equipped with a Histoknife H (Heraeus Kulzer). Sections were stained with 0.5% Toluidine blue and observed using an Axioscope2 microscope (ZEISS).
Protoplast transformation and confocal microscopy
To analyze the localization of DNL-4, pHBT-DNL-4::GFP were generated by introducing amplified cDNAs into NotI/PstI-digested plasmids using the Gibson assembly system (New England Biolabs). Rice protoplasts were isolated from 10-day-old rice seedlings (O.sativaL. cv. Dongjin) were grown in 1 MS media in a growth chamber in the dark at 28°C and then transformed with pHBT-DNL-4::GFP as previously described with slight modification.Citation28 Around 2.5 × 106 protoplasts were transformed with 1 μg of pHBT-DNL-4::GFP The transformed protoplasts were incubated in the dark at 28°C for 10 h, and analyzed using SP8 STED laser scanning confocal microscope (Leica). The GFP was detected between 470 and 550 nm.
Results
A T-DNA insertion mutant exhibits stunted growth
To identify novel genes involved in plant height in rice, we obtained T-DNA insertion mutant seeds, generated by Kim et al.Citation29 using the Ac/Ds system, and screened for dwarf plants. We selected a mutant that was shorter than the wild type and characterized its phenotype (). Throughout development, the dnl-4 mutant plants were shorter than the wild-type controls (–c)). At the mature stage, the height of the dnl-4 mutant was about 40% of that of the wild-type plant ()). We also analyzed internode elongation, leaf blade length, and panicle length in the dnl-4 mutant ()). The lengths of the first, second, and third internodes in the mutant were about 57%, 66%, and 80% of those of the wild-type plants, respectively ()). In the dnl-4 mutant, panicle length, leaf blade length, tiller number and panicle number were decreased by 50%, 27%, 52%, and 53% compared with the wild-type plants, respectively ()). Thus, the dnl-4 mutant has a dwarf phenotype and the site of T-DNA insertion in the mutant is important for plant growth.
Figure 1. Analysis of dnl-4 mutant phenotypes
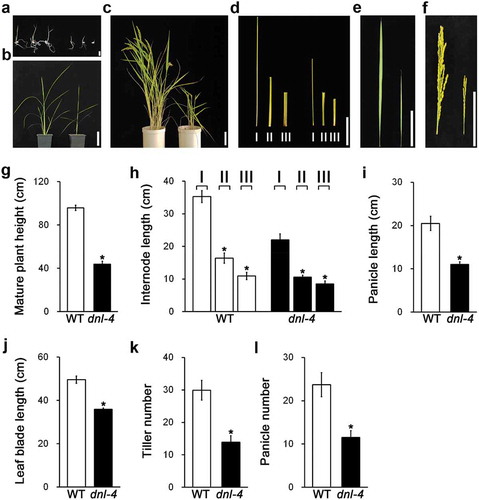
Expression of DNL-4 is suppressed in the dnl-4 mutant
To isolate the T-DNA insertion site in the dnl-4 mutant, we digested genomic DNA from the mutant with NlaIII and used the DNA in an inverse polymerase chain reaction (IPCR, Figure S1). We designed primers for the 5′ and 3′ ends of the Ds element such that a set of two PCR reactions produced overlapping PCR products (Figure S1(a)). The second PCR reaction yielded DNA fragments of 588 bp and 905 bp, which were analyzed by BLASTN to determine the position and orientation of the Ds element (Figure S1(b)). The sequence analysis revealed that the DS element in the dnl-4 mutant is located in the 3ʹUTR of the Os01g0105900 locus. To confirm the position of the Ds element, we performed PCR using a Ds border primer (Ds 3–4, )) paired with a P-F primer, located 464 bp from the 5′ end of the Ds element, or a P-R primer, located 546 bp from the 3′ end. Using these primers, the genomic DNA from mutant was expected to generate a PCR product of 589 bp. Indeed, we obtained PCR products of 506 bp from the homozygous mutant, 1,024 bp from the wild type, and 589 bp and 1,050 bp from the heterozygote. These results confirmed the existence of a T-DNA insertion in the 3ʹUTR of the Os01g0105900 locus in the dnl-4 mutant ()).
Figure 2. Analysis of dnl-4 mutant genotype and Os01g0105900 gene expression level
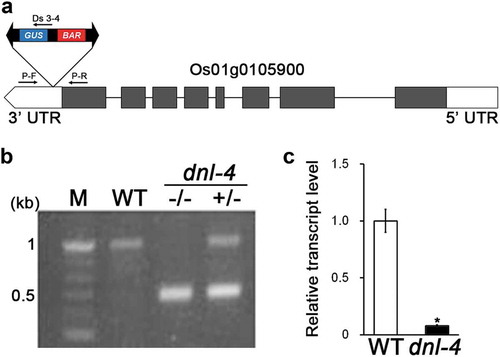
We next analyzed DNL-4 expression by reverse transcription-quantitative polymerase chain reaction (RT-qPCR). Expression was repressed in the homozygous dnl-4 mutant relative to the wild type ()). This result confirmed that the homozygous dnl-4 mutant lacks DNL-4 expression.
DNL-4 encodes a protein of unknown biochemical function
To understand the biochemical function of DNL-4 in plants, we identified DNL-4 like genes in other species (Arabidopsis [Arabidopsis thaliana], wheat [Triticum aestivum], ricinus [Ricinus communis], black cottonwood [Populous trichocarpa], and cacao tree [Theobroma cacao]) by a BLAST search of full amino acid sequences ()). The rice DNL-4 protein was closely related to phosphofructokinase B-type (pfkB) like carbohydrate kinase family proteins in maize and Arabidopsis (Protein numbers: NP_001148411.1 in Zea mays, NP_173390.1 and XP_002890348.1 in Arabidopsis). Recent studies reported that members of this family play roles in the development of photosynthetically competent chloroplasts in plants.Citation18,Citation30 For example, a mutant of rice that lacked expression of FRUCTOKINASE-LIKE PROTEIN 1 (OsFLN1) and OsFLN2 showed an albino phenotype and strongly inhibited seedling growth.Citation18,Citation31 In Arabidopsis, a nara5 knockout mutant showed defective growth and decreased Rubisco content.Citation30 However, the rice dnl-4 mutant did not show an albino phenotype and the DNL-4 sequence does not match that of family members of known biochemical function, such as OsFLN1, OsFLN2, and NARA5 (Figure S2).
Figure 3. Functional characterization of DNL-4. (a) Phylogenetic tree showing the relationship between DNL-4 homologs in rice, arabidopsis, wheat, ricinus, populous, and cacao. The red box corresponds to DNL-4. Full-length of amino acid sequences were used for these analyses. Protein number from NCBI. Red box indicates DNA-4. (b) Expression of DNL-4 in 14-week-old rice. Tissues: panicle (P), leaf blade (LB), leaf sheath (LS), internode (IN), and root (R). Total RNAs were extracted from the indicated samples, and the expression level was analyzed by quantitative RT-PCR. Data represent mean value of three biological replicates, and error bars indicate SD. OsActin1 (Os03g0718100) was used as an internal control and relative expression levels are shown in fold values. Asterisks indicate statistically significant differences between the corresponding samples and their control (p value < .01, t-test). (c) Subcellular localization of DNL-4. Transient expression of DNL-4-GFP fusion constructs in protoplast were carried out to determine the subcellular localization. Scale bars indicate 10 µm
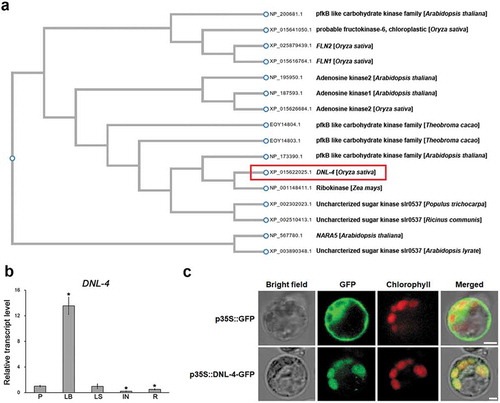
To investigate the organ specificity of DNL-4 expression in rice, we extracted total RNA from the panicle, leaf blade, leaf sheath, internode, and root and measured DNL-4 transcript levels by RT-qPCR ()). In mature plants, the expression of DNL-4 was higher in the leaf blade than in the other tissues. The internode and root exhibited lower expression of DNL-4 than the other tissues, indicating that expression of DNL-4 is tissue specific. To determine the subcellular localization of DNL-4, we investigated the subcellular localizations of fluorescent protein–tagged DNL-4 using a rice protoplast transient expression system ()). DNL-4 co-localized with chloroplast. pfkB carbohydrate kinases are in chloroplast locations to perform their specific function. These results, together with the chloroplast localization of DNL-4 and the analyzed expression pattern of DNL-4, suggest that DNL-4 functions during development of the leaf blade.
The dnl-4 mutant has narrow leaf blades
To investigate the role of DNL-4 in leaf blade development, we analyzed the morphology of leaf blades and the histology of leaf blade cross sections in the dnl-4 mutant (). The leaf blades of the dnl-4 mutant were 20% narrower than those of wild-type plants ()). We also analyzed the formation of leaf veins in the mutant. There was no significant difference in the number of large veins in the mutant compared with the wild type. However, the number of small veins was reduced by 8% in the dnl-4 mutant ()). These results indicated that expression of DNL-4 is necessary for normal formation of small veins in the leaf blade.
Figure 4. The dnl-4 mutant has defects in the vascular system of the leaf blade
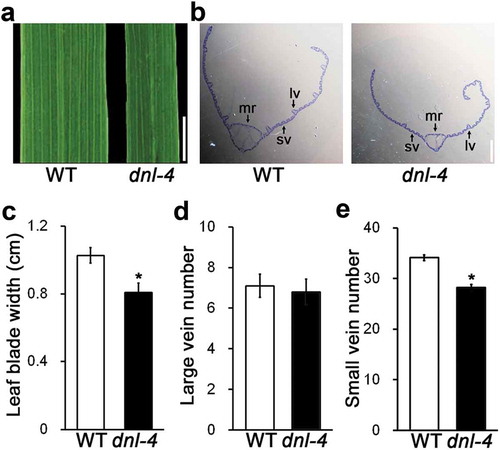
The dnl-4 mutant shows altered leaf inclination and bulliform cell patterning
Having demonstrated that DNL-4 influences leaf width, we then tested whether DNL-4 expression is required for normal leaf morphology. We analyzed the inclination of leaves of the dnl-4 mutant grown under field conditions ()). The leaf inclination of the dnl-4 mutant was increased by 3.75-fold compared with the wild type ()).
Figure 5. Analysis of leaf inclination and cellular composition of dnl-4 mutants at the ripening stage
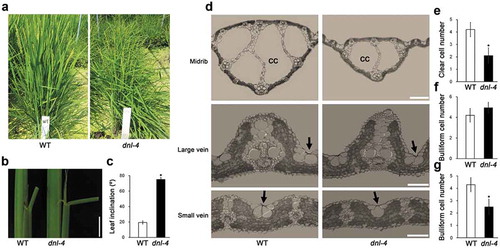
To further explore the effect of the loss of DNL-4 on the cellular composition of leaves, we analyzed the structure of the midrib and bulliform cells in leaf cross sections of the dnl-4 mutant ()). Rice leaves have clear cells, which are large locules, in the central region of the midrib.Citation32 The number of clear cells was reduced by 47% in the dnl-4 mutant ()). Bulliform cells are mainly located near the leaf surface in monocots and play important roles in water stress and leaf rolling.Citation33–36 We examined that number and distribution pattern of bulliform cells in the large vein and small vein areas of the dnl-4 mutant ()). The number of bulliform cells in the large vein area was not significantly different between the mutant and the wild-type plants. However, the number of bulliform cells in small vein area was reduced by 58% in the dnl-4 mutant. These results indicated that expression of DNL-4 affects leaf inclination and cellular composition, suggesting that this gene has a key role in photosynthesis and growth in rice.
The dnl-4 mutant shows delayed heading date and reduced seed width
Because leaf morphology strongly influences photosynthesis and the productivity of plants, we expected that expression of DNL-4 would also affect rice productivity. The dnl-4 mutant had fewer and shorter mature panicles than the wild type ()). Because heading date and seed size are significant agronomic traits associated with productivity, we analyzed these traits in the wild type and the dnl-4 mutant. The heading date of the dnl-4 mutant was delayed by over 15 days compared with that of the wild-type plants ()). The seeds of the dnl-4 mutant were the same length as wild-type seeds but were narrower ()). These results indicated DNL-4 expression affects heading date and seed size, suggesting that this gene influences rice productivity.
Figure 6. Analysis of heading date in wild-type and dnl-4 mutant
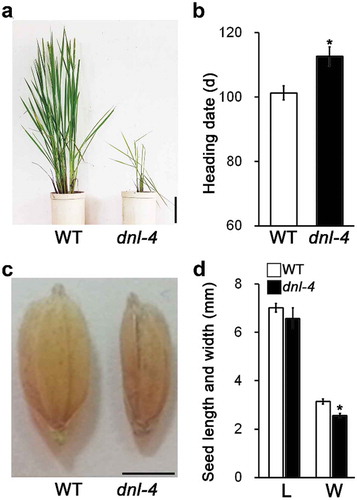
Discussion
A number of dwarf and narrow leaf mutants of rice have been produced by mutagenesis and the associated genes identified, such as nal1, nal2, nal3, nal4, nal5, nal6, nal7, dnl1, dnl2, and dnl3.Citation8,Citation12,Citation37–40 Some of the dwarf mutants have been reported to have reduced grain size, such as d11, d1, and d18, and others have been reported to form narrow or rolled leaves, such as d2, d6, tdd1, rl1, nal2, nal3, dtl1, and dnl1.Citation38,Citation39 Previous studies reported that the two type of tiller number associated with plant height in rice. The htd1, htd2, d10, d27, and d53 mutants have increased tiller number compared to the wild type,Citation37,Citation38,Citation40 whereas Oschr4-5 and Osdlt10 mutants have reduced tiller number compared to the wild type in association with auxin signaling pathway.Citation41,Citation42 The dnl-4 mutant exhibited reduced plant height, panicle size, grain width, tiller number and leaf width. These morphological characteristics of the dnl-4 mutant are similar to those of other mutants that exhibit dwarfism with a narrow-leaf and reduced tiller number. Plant height and tiller number is an important agronomic trait that affects rice yield. Furthermore, leaf morphology affects photosynthetic efficiency and plant growth and consequently plant growth and productivity.Citation38
A BLAST analysis of DNL-4 indicated that it was a member of the phosphofructokinase B-type (pfkB) like carbohydrate kinase family ()). However, the DNL-4 protein sequence did not match those of OsFLN1 and OsFLN2 (Figure S2) and the functions of most of the other pfkB carbohydrate kinase proteins that have been identified in plants have not yet been characterized. We showed that the dnl-4 mutant exhibits altered cellular composition in the leaf blade, defective grain formation, and dwarfism. These results will be useful for understanding the molecular function of pfkB carbohydrate kinases involved in determining leaf morphology and plant height.
In the rice nal1 mutant, the number of small veins in leaves is reduced by approximately 50%, whereas the number of large veins is only slightly reduced compared to the wild type.Citation12 Like the nal1 and nal2 mutants, dnl-4 showed a significant decrease in the number of small veins, whereas the number of large veins was not significantly decreased compared with the wild-type plants ()). Furthermore, we analyzed the expression level of dwarf and narrow leaf-related genes, such as NAL1, NAL7, and CSLD4 in the dnl-4 mutant (Figure S3). In the dnl-4 mutant, the expression of NAL1 and NAL7 were downregulated, whereas the expression of CSLD4 was not significantly different compared with the wild type. The correlation of DNL-4 expression with that of NAL1 and NAL7 suggests that NAL1 and NAL7 are involved in DNL-4 mediated leaf development in rice.
In this study, we also showed that lack of DNL-4 expression induces defective rice growth and grain formation under normal growth conditions. These observations suggest that DNL-4 plays an essential role in both rice growth and productivity. It is largely unknown how pfkB carbohydrate kinase proteins regulate growth and productivity in rice. The morphology of the dnl-4 mutant suggests that regulation of cellular formation in leaves, internode elongation, and/or panicle length might be the mechanism(s) by which this pfkB carbohydrate kinase protein mediates rice growth and productivity. Further molecular and biochemical studies will expand our understanding of the mechanisms underlying this process.
Disclosure of potential conflicts of interest
No potential conflicts of interest were disclosed.
Accession numbers
Sequence data from this article can be found in the Rice Annotation Project (RAP-DB) under the following accession numbers: DNL-4 (Os01g0105900), NAL1 (Os04g0615000), NAL7 (Os03g0162000).
Supplemental Material
Download MS Word (2.3 MB)Supplementary material
Supplemental data for this article can be accessed on the publisher’s website.
Additional information
Funding
Reference
- Walter A, Silk WK, Schurr U. Environmental effects on spatial and temporal patterns of leaf and root growth. Annu Rev Plant Biol. 2009;60(1):1–9. doi:10.1146/annurev.arplant.59.032607.092819.
- Govaerts YM, Jacquemoud S, Verstraete MM, Ustin SL. Three-dimensional radiation transfer modeling in a dicotyledon leaf. Appl Opt. 1996;35(33):6585–6598. doi:10.1364/AO.35.006585.
- Denning, G.L. & Mew, T.W., 1997. China and IRRI” Improving China's rice productivity in the 21st century. IRRI Discussion Papers 287591, International Rice Research Institute (IRRI).
- Tsukaya H. Mechanism of leaf-shape determination. Annu Rev Plant Biol. 2006;57(1):477–496. doi:10.1146/annurev.arplant.57.032905.105320.
- Lang YZ, Zhang ZJ, Gu XY, Yang JC, Zhu QS. Physiological and ecological effects of crimpy leaf character in rice (Oryza sativa L.) II. Photosynthetic character, dry mass production and yield forming. Acta Agron Sin. 2004;30:883–887.
- Zhang GH, Xu Q, Zhu XD, Qian Q, Xue HW. SHALLOT-LIKE1 is a KANADI transcription factor that modulates rice leaf rolling by regulating leaf abaxial cell development. Plant Cell. 2009;21(3):719–735. doi:10.1105/tpc.108.061457.
- Koch G, Rolland G, Dauzat M, Bédiée A, Baldazzi V, Bertin N, Guédon Y, Granier C. Leaf production and expansion: a generalized response to drought stresses from cells to whole leaf biomass—a case study in the tomato compound leaf. Plants. 2019;8(10):409. doi:10.3390/plants8100409.
- Adedze YMN, Wei XJ, Sheng ZH, Jiao GA, Tang SQ, Hu PS. Characterization of a rice dwarf and narrow leaf 2 mutant. Biologia Plantarum. 2016;61(1):85–94. doi:10.1007/s10535-016-0632-4.
- Weingartner M, Pelayo HR, Binarova P, Zwerger K, Melikant B, Torre C, Heberle-Bors E, Bögre L. A plant cyclin B2 is degraded early in mitosis and its ectopic expression shortens G2-phase and alleviates the DNA-damage checkpoint. J Cell Sci. 2003;116(3):487–498. doi:10.1242/jcs.00250.
- Zhao SQ, Hu J, Guo LB, Qian Q, Xue HW. Rice leaf inclination2, a VIN3-like protein, regulates leaf angle through modulating cell division of the collar. Cell Res. 2010;20(8):935–947. doi:10.1038/cr.2010.109.
- Zou LP, Sun XH, Zhang ZG, Liu P, Wu JX, Tian CJ, Qiu JL, Lu TG. Leaf rolling controlled by the homeodomain leucine zipper class IV gene Roc5 in rice. Plant Physiol. 2011;156(3):1589–1602. doi:10.1104/pp.111.176016.
- Qi J, Qian Q, Bu Q, Li S, Chen Q, Sun J, Liang W, Zhou Y, Chu C, Li X, et al. Mutation of the rice Narrow leaf1 gene, which encodes a novel protein, affects vein patterning and polar auxin transport. Plant Physiol. 2008;147(4):1947–1959. doi:10.1104/pp.108.118778.
- Fujino K, Mastuda Y, Ozawa K, Nishimura T, Koshiba T, Fraaije MW, Sekiguchi H. NARROW LEAF 7 controls leaf shape mediated by auxin in rice. Mol Genetics Genomics. 2008;279(5):499–507. doi:10.1007/s00438-008-0328-3.
- Li M, Xiong G, Li R, Cui J, Tang D, Zhang B, Pauly M, Cheng Z, Zhou Y. Rice cellulose synthase‐like D4 is essential for normal cell‐wall biosynthesis and plant growth. Plant J. 2009;60(6):1055–1069. doi:10.1111/j.1365-313X.2009.04022.x.
- Park J, Gupta RS. Adenosine kinase and ribokinase–the RK family of proteins. Cell Mol Life Sci. 2008;65(18):2875–2896. doi:10.1007/s00018-008-8123-1.
- Pego JV, Smeekens SC. Plant fructokinases: a sweet family get-together. Trends Plant Sci. 2000;5(12):531–536. doi:10.1016/S1360-1385(00)01783-0.
- Stein O, Granot D. Plant fructokinases: evolutionary, developmental, and metabolic aspects in sink tissues. Front Plant Sci. 2018;9:339. doi:10.3389/fpls.2018.00339.
- Gilkerson J, Perez-Ruiz JM, Chory J, Callis J. The plastid-localized pfkB-type carbohydrate kinases FRUCTOKINASE-LIKE 1 and 2 are essential for growth and development of Arabidopsis thaliana. BMC Plant Biol. 2012;12(1):102. doi:10.1186/1471-2229-12-102.
- He L, Zhang S, Qiu Z, Zhao J, Nie W, Lin H, Zhu Z, Zeng D, Qian Q, Zhu L. Fructokinase-like protein 1 interacts with TRXz to regulate chloroplast development in rice. J Integr Plant Biol. 2018;60(2):94–111. doi:10.1111/jipb.12631.
- Lv Y, Shao G, Qiu J, Jiao G, Sheng Z, Xie L, Wu Y, Tang S, Wei X, Hu P. White Leaf and Panicle 2, encoding a PEP-associated protein, is required for chloroplast biogenesis under heat stress in rice. J Exp Bot. 2017;68(18):5147–5160. doi:10.1093/jxb/erx332.
- Kolesnik T, Szeverenyi I, Bachmann D, Kumar CS, Jiang S, Ramamoorthy R, Cai M, Ma ZG, Sundaresan V, Ramachandran S. Establishing an efficient Ac/Ds tagging system in rice: large‐scale analysis of Ds flanking sequences. Plant J. 2004;37(2):301–314. doi:10.1046/j.1365-313x.2003.01948.x.
- Moreno MA, Chen J, Greenbaltt I, Dellaporta SL. Reconstitutional mutagenesis of the maize P gene by short-range Ac transpositions. Genetics. 1992;131:939–956.
- Pooma W, Gersos C, Grotewold E. Transposon insertions in the promoter of the Zea mays a1 gene differentially affect transcription by the Myb factors P and C1. Genetics. 2002;161:793–801.
- Clarke JD. Cetyltrimethyl ammonium bromide (CTAB) DNA miniprep for plant DNA isolation. Cold Spring Harbor Protoc. 2009:5177–5178. doi:10.1101/pdb.prot5177.
- Ochman H, Gerber AS, Hartl DL. Genetic applications of an inverse polymerase chain reaction. Genetics. 1988;120:621–623.
- Simms D, Cizdziel PE, Chomczynski P. TRIZOL™: A new reagent for optimal single-step isolation of RNA. Focus. 1993;15:99–102.
- Chieco C, Rotondi A, Morrene L, Rapparini F, Baraldi R. An ethanol-based fixation method for anatomical and micro-morphological characterization of leaves of various tree species. Biotech Histochem. 2012;88(2):109–119. doi:10.3109/10520295.2012.746472.
- Yang JW, Fu JX, Li J, Cheng XL, Li F, Dong JF, Liu ZL, Zhuang CX. A novel co-immunoprecipitation protocol based on protoplast transient gene expression for studying protein–protein interactions in rice. Plant Mol Biol Rep. 2014;32(1):153–161. doi:10.1007/s11105-013-0633-9.
- Kim CM, Piao HL, Park SJ, Chon NS, Je BI, Sun B, Park SH, Park JY, Lee EJ, Kim MJ, et al. Rapid, large-scale generation of Ds transposant lines and analysis of the Ds insertion sites in rice. Plant J. 2004;39(2):252–263. doi:10.1111/j.1365-313X.2004.02116.x.
- Ogawa T, Nishimura K, Aokin T, Takase H, Tomizawa KI, Ashida H, Yokota A. A phosphofructokinase B-type carbohydrate kinase family protein, NARA5, for massive expressions of plastid-encoded photosynthetic genes in Arabidopsis. Plant Physiol. 2009;151(1):114–128. doi:10.1104/pp.109.139683.
- Qiu Z, Kang S, He L, Zhao J, Zhang S, Hu J, Zeng D, Zhang G, Dong G, Gao Z, et al. The newly identified heat-stress sensitive albino 1 gene affects chloroplast development in rice. Plant Sci. 2018;267:168–179. doi:10.1016/j.plantsci.2017.11.015.
- Yamaguchi T, Nagasawa N, Kawasaki S, Mastuoka M, Nagato Y, Hirano HY. The YABBY gene DROOPING LEAF regulates carpel specification and midrib development in Oryza sativa. Plant Cell. 2004;16(2):500–509. doi:10.1105/tpc.018044.
- Li L, Shi ZY, Li L, Shen GZ, Wang XQ, An LS, Zhang JL. Overexpression of ACL1 (abaxially curled leaf 1) increased bulliform cells and induced abaxial curling of leaf blades in rice. Mol Plant. 2010;3(5):807–817. doi:10.1093/mp/ssq022.
- Jane WN, Chiang SHT. Morphology and development of bulliform cells in Arundo Formosana Hack. Taiwania. 1991;36(1):85-97. doi:10.6165/tai.1991.36.85.
- O’toole JC, Cruz RT, Singh TN. Leaf rolling and transpiration. Plant Sci Letters. 1979;16(1):111–114. doi:10.1016/0304-4211(79)90015-4.
- O’Toole JC, Cruz RT. Response of leaf water potential, stomatal resistance, and leaf rolling to water stress. Plant Physiol. 1980;65(3):428–432. doi:10.1104/pp.65.3.428.
- Wei XJ, Tang SQ, Shao GN, Chen ML, Hu YC, Hu PS. Fine mapping and characterization of a novel dwarf and narrow-leaf mutant dnl1 in rice. Genet Mol Res. 2013;12(3):3845–3855. doi:10.4238/2013.September.23.2.
- Shi L, Wei XJ, Adedze YMN, Sheng ZH, Tang SQ, Hu PS, Wang JL. Characterization and gene cloning of the rice (Oryza sativa L.) dwarf and narrow-leaf mutant dnl3. Genet Mol Res. 2016;15(3). doi:10.4238/gmr.15038731.
- Sazuka T, Kamiya N, Nishimura T, Ohmae K, Sato Y, Imamura K, Nagato Y, Koshiba T, Nagamura Y, Ashikari M, et al. A rice tryptophan deficient dwarf mutant, tdd1, contains a reduced level of indole acetic acid and develops abnormal flowers and organless embryos. Plant J. 2009;60(2):227–241. doi:10.1111/j.1365-313X.2009.03952.x.
- Zhao J, Wang T, Wang M, Liu Y, Yuan S, Gao Y, Yin L, Sun W, Peng L, Zhang W, et al. DWARF3 participates in an SCF complex and associates with DWARF14 to suppress rice shoot branching. Plant Cell Physiol. 2014;55(6):1096–1109. doi:10.1093/pcp/pcu045.
- Guo T, Wang D, Fang J, Zhao J, Yuan S, Xiao L, Li X. Mutations in the rice OsCHR4 gene, encoding CHD3 family chromatin remodeler, induce narrow and rolled leaves with increased cuticular wax. Int J Mol Sci. 2019;20(10):2567. doi:10.3390/ijms20102567.
- Wen X, Sun L, Chen Y, Xue P, Yang Q, Wang B, Yu N, Cao Y, Zhang Y, Gong K, et al. Rice dwarf and low tillering 10 (OsDLT10) regulates tiller number by monitoring auxin homeostasis. Plant Sci. 2020;297:110502. doi:10.1016/j.plantsci.2020.110502.