Abstract
In this investigation, N,N′-(Pyromellitoyl)-Bis-D-4-hydroxyphenylglycine dimethyl ester as a bioactive diphenolic monomer was prepared in three steps. The intelligent design of the novel aromatic diol which is bearing biodegradability, thermal stability, and polar parts in the structure has been led to obtained polymers with modified characteristics. This monomer can be regarded as a new biodegradable diol which may be employed as replacements for the industrially used toxic diphenols such as bisphenol-A (BPA) in the design of biodegradable materials. Also based on quantum chemistry calculations, we can estimate properties of new compounds by cyberspace reaction path (computational chemistry) before entering the lab to pursue the reaction. We used computational methods, especially DFT and basic functions and 6-311G** method, to estimate properties of novel synthesized nontoxic diphenolic monomer derived from D-4-hydroxyphenylglycine and compared with BPA by means of their geometric and electronic structures, the corresponding energies (HOMO, LUMO, and ΔEgap), and heat resistance. The comparison between theoretical and experimental results showed that the novel nontoxic synthesized aromatic diol has good properties compared to BPA. The polycondensation of the monomer with various aromatic and aliphatic diisocyanates such as 4,4′-methylenebis-(4-phenylisocyanate) (6a), toluylene-2,4-diisocyanate (6b), and hexamethylene diisocyanate (6c) was carried out under traditional polymerization conditions. The novel diphenolic monomer and resulted poly(urethane-imide)s were characterized with Fourier Transform Infrared Spectroscopy (FT-IR), 1H-NMR, elemental, and thermo-gravimetric analysis techniques. Soil burial test of the diol 5, and the obtained PUs and soil enzymatic assay, showed that the synthesized diol and its polymer are biologically active and probably biodegradable in soil conditions.
1. Introduction
Bisphenols, (BPs) such as bisphenol-A (BPA, 2,2-bis(4-hydroxydiphenyl)propane), are a group of chemical materials which consist of two phenolic rings joined together through a bridging carbon or other chemical structures.[Citation1] BPA is an organic compound composed of two phenol rings connected by a methyl bridge, with two methyl functional groups attached to the bridge. BPA is frequently used in manufacturing high-performance materials, since their aromatic backbone structures can significantly increase the stiffness and mechanical strength of obtained polymers. BPA is used as a material for the production of phenol resins, polyacrylates, and polyesters, but mainly for the production of epoxy resins and polycarbonate plastics.[Citation2] The epoxy resins are used as food-contact surface lacquer coatings for cans, metal jar lids, protective coatings and finishes, automobile parts, adhesives, aerospace applications and as a coating for PVC pipes. Recently, the annual production of BPA in the world has been increased. However, due its more demand, its application is being increased year by year. Therefore, the discharge of a variety of BPs into the environment is estimated to be increased, and it seems that various BPs as well as BPA becomes widespread environmental pollutants in the near future. As mentioned above, several researchers have focused on the toxicity of BPA, especially its estrogenic activity, and many data are available at present.[Citation3–5]
BPA and other industrially used diphenols are toxic and may not be suitable candidate as monomers in medical implant and biodegradable materials. So, there is a significant need for a nontoxic diphenolic monomer that could be used as a building block in the design of biodegradable materials. This need was addressed by the development of some tyrosine-based monomers.[Citation6–9] D-4-hydroxyphenylglycine is the natural nutrient amino acid containing an aromatic hydroxyl group. Optically active D-amino acids are widely used in the pharmaceutical industry as intermediates for the production of semisynthetic antibiotics, pesticides, and new drugs.[Citation10–13] Activated forms of D-4-hydroxyphenylglycine are also employed in the enzymatic synthesis.[Citation14,15]
Density functional theory (DFT) methods are often considered to be ab initio methods for determining the molecular electronic structure, even though many of the most common functional parameters are derived from empirical data, or from more complex calculations.[Citation16,17] In DFT, the total energy is expressed in terms of the total one-electron density rather than the wave functions. In this type of calculation, there is an approximate Hamiltonian and an approximate expression for the total electron density. DFT methods can be very accurate for little computational cost. Some methods combine the density functional exchange with the Hartree–Fock exchange term and are known as hybrid functional methods.[Citation17]
The task of this investigation is to use aromatic hydroxyl group of D-4-hydroxyphenylglycine amino acid as new chiral bioactive diphenolic monomer candidate for the preparation of biodegradable polymers. In planning of this monomer, some notification is considered. Biological activity is due to the presence of D-4-hydroxyphenylglycine amino acids in the main chain, thermal stability is due to the presence of imide linkages, and solubility is because of the existing of pendant and polar methyl ester group in the side chain of the synthetic materials. Therefore, the combination of these different properties within one single design of the aromatic diol backbone was led to synthesis of N,N′-(pyromellitoyl)-bis-D-4-hydroxyphenylglycine dimethyl ester in three steps which may be considered as nontoxic diphenolic monomer derived from D-4-hydroxyphenylglycine. The use of this novel aromatic diol in direct polycondensation, instead of significantly toxic and industrial diol, such as BPA, is one of the main and important prerequisites for the study of biodegradable polymers. In order to test this hypothesis, our goal was to synthesize a series of biodegradable poly(urethane-imide) in which natural D-amino acids were linked together by amide bonds. DFT with B3LYP functional and the 6-31G basis set was applied to determine their geometric and electronic structures and the corresponding energies (HOMO, LUMO, and ΔEgap) of novel diphenolic monomer and BPA. The calculated spectroscopy data such as Fourier Transform Infrared Spectroscopy (FT-IR) and 1H-NMR by DFT theory will be compared with the experimental data of novel synthetic diol and BPA.
2. Experimental
2.1. Chemicals
All chemicals were purchased from Fluka Chemical Co. (Buchs, Switzerland), Aldrich Chemical Co. (Milwaukee, WI), and Merck Chemical Co. Pyromellitic dianhydride (benzene-1,2,4,5-tetracarboxylic dianhydride) (1) (from Merck Chemical Co) was used without purification. N,N-dimethylacetamide (DMAc). DMF and N-methyl-2-pyrrolidone (NMP) were dried over barium oxide (BaO) and then were distilled under reduced pressure. Triethylamine (TEA) was also dried over BaO and then was distilled.
2.2. Instruments
The purity of compounds was checked by thin layer chromatography (TLC) using cyclohexane/ethyl acetate (1:1 v/v) as an eluent. Nuclear Magnetic Resonance (1H NMR, 400 and 500 MHz) spectra and also Carbon Nuclear Magnetic Resonance (13C-NMR, 125 MHz) spectrum were recorded in dimethyl sulfoxide-d6 (DMSO-d6) solution using a Bruker (Germany), at Kashan and Sharif Universities, Esfahan and Tehran, respectively, Iran. FT-IR spectra were recorded on Perkin Elmer (Germany) C88731 spectrophotometer. The spectra of solids were obtained using KBr pellets. The vibrational transition frequencies are reported in wave numbers (cm−1). Band intensities are assigned as weak (w), medium (m), shoulder (sh), strong (s), and broad (br). Elemental analysis was performed using a Bruker (Germany) EQUINOX55 instrument. Inherent viscosities were measured by a Cannon-Fenske Routine Viscometer (Germany) at the concentration of 0.5 g dL−1 at 25 °C. Solubility of the polymers was tested in various polar and nonpolar solvents. A sample of 5 mg of the polymer was added to 1 ml of different solvents. Thermal gravimetric analysis (TGA) was performed using a Perkin Elmer instrument under nitrogen atmosphere at a rate of 10 °C min−1, and differential scanning calorimetry (DSC) was performed using a DSC-PL-1200 instrument at a heating rate of 20 °C min−1 under N2 atmosphere. Cultured samples in Petri plate were imaged with a confocal digital camera (Canon DS126181, Japan).
2.3. Monomer synthesis
Synthesis and characterizations of novel bioactive aromatic diol 5 is reported in detail.
2.3.1. Synthesis of D-4-hydroxyphenylglycine methyl ester hydrochloride (D-PhGlyOMe.HCl) (2)
3 g (1.79 × 10−2 mol) of D-4-hydroxyphenylglycine (D-4-HO-PhGlyOH) (1) was added in 15 mL of dry methanol containing thionyl chloride (3.6 mL, 5.37 × 10−2 mol) at −10 °C. The solution was stirred at room temperature for 12 h until the suspension was converted to a clear solution and then was concentrated under vacuum to remove the methanol in excess. The precipitated was washed with diethyl ether (15 mL) three times to obtain white powdery D-4-HO-PhGlyOH.HCl (2), which was used for the next step, without further purification. Yield 3.45 g (88%); mp 178–180 °C, Lit 179 °C.[Citation14]
FT-IR KBr (cm−1): 3341 (s), 2884 (s), 2624 (w), 1743 (s), 1614 (m), 1591 (m), 1514 (s), 1448 (m), 1396 (w), 1348 (w), 1313 (w), 1291 (m), 1274 (m), 1284 (s), 1225 (s), 1198 (m), 1175 (w), 1141 (w), 1107 (m), 1059 (m), 990 (m), 934 (w), 908 (w), 865 (w), 839 (s), 793 (m), 731 (m), 599 (w), 513 (w), 467 (m).
13C-NMR (125 MHz, DMSO-d6): 53.41(O–CH3), 55.37 (CH, chiral center), 116.13 (2CH, aromatic), 123.03 (C, aromatic), 130.05 (CH, aromatic), 159.04 (C, aromatic), 169.64 (C=O, ester).
1H-NMR (400 MHz, DMSO-d6): δ 3.66 (s, 3H), 5.067 (s, 1H), 6.80–6.82 (d, 2H, Ar–H, J = 8 Hz), 7.25–7.27 (d, 2H, Ar–H, J = 8 Hz), 8.96 (s, 3H, NH3+), 9.97 (s, 1H, OH).
2.3.2. Synthesis of D-4-hydroxyphenylglycine methyl ester (D-4-HO-PhGlyOMe) (3)
D-4-HO-PhGlyOH.HCl (3 g, 1.37 × 10−2 mol) was suspended in 20 mL of dry dichloromethane together with triethylamine (2.86 mL, 2.06 × 10−2 mol). After 1.5 h of magnetic stirring at 0 °C under nitrogen, the organic solution was removed under reduced pressure to obtain the free amines. Then, the residual mass was washed with H2O (60 mL), followed by recrystallization from DMF to obtain a colorless solid, D-4-HO-PhGlyOMe. Yield: 2 g (80.32%); mp: 141–143 °C, Lit 143 °C.[Citation14]
FT-IR KBr (cm−1): 3355 (s), 3300 (w), 2605–3009 (w),1744 (s), 1613 (m), 1597 (s), 1515 (m), 1480 (m), 1443 (m), 1382 (w), 1302 (w), 1258 (s), 1221 (m), 1196 (s), 1178 (s), 1146 (s), 1104 (w), 1020 (s), 992 (w), 959 (w), 908 (m), 872 (w), 838 (s), 795 (w), 732 (w), 637 (w), 574 (w), 512 (w), 460 (w), 421 (w).
13C-NMR (125 MHz, DMSO-d6): 52.12(O–CH3), 58.07 (CH, chiral center), 115.47 (2CH, aromatic), 128.38 (2CH, aromatic), 131.78 (C, aromatic), 157.16 (C, aromatic), 175.33 (C=O, ester).
1H-NMR (400 MHz, DMSO-d6): δ 2.41 (s, 2H, NH2), 3.54 (s, 3H,O–CH3), 4.37 (s, 1H), 6.66–6.68 (d, 2H, Ar–H, J = 8 Hz), 7.11–7.13 (d, 2H, Ar–H, J = 8 Hz), 9.36 (s, 1H, OH).
2.3.3. N,N′-(Pyromellitoyl)-Bis-D-4-hydroxyphenylglycine dimethyl ester (N,N′-PMB- D-4-HO-PhGlyOMe) (5)
Into a 25-ml round-bottomed flask, 0.50 g (2.29 × 10−3 mol) of pyromellitic dianhydride (4), 0.82 g (4.58 × 10−3 mol) of D-4-HO-PhGlyOMe (3), 8.0 ml of DMF and 3.0 ml toluene, and then a stirring bar, were placed. The reactions were set up for reflux for 8 h through a Dean-Stark trap using a slow nitrogen atmosphere and mechanical stirring. Toluene was used as a water-azeotroping solvent. The yellow solution was distillated under reduced pressure. The residue was poured into in a mixture of 50 mL/5 mL of cold water/concentrated HCl. The yellow precipitate was collected by filtration and washed off thoroughly with water and dried at 70 °C for 8 h to yield 0.8 g (61%) of diol 5 mp: 138–140 °C.
FT-IR (KBr): 3468 (m, br), 2995 (w), 1772(m), 1725 (s), 1618 (w), 1599 (w), 1521 (s), 1440 (m), 1390 (s), 1366 (s, br), 1255 (m), 1129 (m), 1021 (m), 802 (m), 7544 (m).
1H-NMR (400 MHz, DMSO-d6): δ 3.68 (s, 6H,O–CH3), 5.48 (s, 2H), 6.66–6.68 (d, 2H, Ar–H, J = 8 Hz), 7.11–7.13 (d, 2H, Ar–H, J = 8 Hz), 8.24–8.25 (s, 2H, Ar–H), 9.26 (s, 2H, OH).
13C-NMR (125 MHz, DMSO-d6): 53.61 (CH, chiral center), 59.98 (CH3–O), 120.02 (4CH, aromatic), 124.38 (2CH, aromatic), 132.48 (2CH, aromatic), 134.38 (4CH, aromatic), 140.04 (4C, aromatic), 159.08 (2C, aromatic), 167.74 (4C=O, imidic), 173.74 (2C=O, ester).
Elemental analysis calculated for (C28H20N2O10)n(544.47 g mol−1): C, 62.94%; H, 4.23%; N, 4.89%; Found: C, 61.77%; H, 3.70%; N, 5.15%.
2.4. Polymer synthesis
The PUI7a–PUI7c were prepared by the following general procedure (with PUI7a as an example): A three-necked flask containing diol 5 (0.10 g, 1.83 × 10−4 mol) and triethyl amine (Et3 N) (0.01 g, 1.58 × 10−5 mol) as catalyst and 2 ml of freshly distilled NMP was stirred under nitrogen atmosphere at room temperature for 30 min. A solution of (0.046 g, 1.83 × 10−4 mol) MDI in 1.5 ml of freshly distilled NMP was added drop wise through a dropping funnel in 10 min. The mixture was vigorously stirred at room temperature for 1 h and then was heated at 80 °C for 8 h, and then, it was heated gradually from 100 to 120 °C for 2 h. The mixture was cooled down to room temperature, poured into 20 mL of methanol/distillated water (10/10), and the precipitate was flocculated. The product was filtered, washed thoroughly with hot water and methanol, and collected by filtration and dried at 100 °C under reduced pressure. A 86% yield was obtained, and inherent viscosity of this polymer was 0.42 dL/g, measured with a polymer concentration of 0.5 g/dL in DMF at 25 °C. The other PIU7b–PUI7c were synthesized in a procedure similar to that described above.
PUI7a: FT-IR (KBr, cm−1): 3323 (m, br), 2964 (m), 2854 (m), 1778 (m), 1724 (s), 1645 (m), 1600 (m), 1447 (m), 1384 (s), 1242 (m, br), 1124 (m), 1017 (m), 843 (m), 725 (m). 1H-NMR (500 MHz, DMSO-d6): δ 3.96 (s, 6H, O–CH3), 4.38 (s, 2H, CH2), 5.32 (s, 2H, CH2), 7.02–7.03 (d, 4H, Ar–H, J = 8.00 Hz), 7.32–7.34 (d, 4H, Ar–H, J = 8.00 Hz), 7.48–7.50(d, 4H, Ar–H, J = 10.00 Hz), 7.73–7.75 (d, 4H, Ar–H, J = 10.00 Hz), 8.47(s, 1H, Ar–H), 9.36 (s, 2H, N–H).
PUI7b: FT-IR (KBr, cm−1): 3521 (m), 3339 (m), 2964 (m), 2956 (m), 2858 (m), 1776 (m), 1725 (s), 1617 (m), 1577 (m, br), 1517 (m), 1452 (m), 1385 (s), 1366 (m), 1255 (m, br), 1217 (m), 1234 (m), 1116 (m), 838 (w).
PUI7c: FT-IR (KBr, cm−1): 3328 (m, br), 2936 (s), 2859 (m), 1776 (m), 1725 (s), 1622 (s), 1578 (m), 1536 (m), 1459 (m, br), 1384 (s), 1255 (m, br), 1124 (m), 1115 (m), 916 (m), 833 (m), 724 (w), 629 (w,br).
1H-NMR (500 MHz, DMSO-d6): δ 1.26 (s, 8H, 4 CH2), 2.94–2.96 (m, 4H, CH2–N), 3.69 (s, 6H, O–CH3), 5.28 (s, 2H, CH), 6.67–6.68 (d, 2H, Ar–H, J = 8 Hz), 7.11–7.13 (d, 2H, Ar–H, J = 8 Hz), 8.25 (s, 2H, Ar–H), 9.13 (s, 2H, N–H).
2.5. Soil burial test
In aerobic soil condition and in the presence of natural microorganisms, a sample of 12 mg from each 2, 3, and 5 and PUI7a compounds were mixed with 1 g of clay loam soil. Buried Materials were put in capped 1 ml plastic vials in which the relative humidity maintained at 60–70% by spraying water, and the temperature was thermostated at 28–30 °C in a humidity chamber (Ogawa Co., Japan). The buried compounds were monitored for 6 months at regular interval of 10 days, and then, 0.1 g of the soil around samples was excavated and mixed with 1 ml de-ionized sterile water. After 1 min agitation with vortex, the 100 μl of supernatant was sampled and streak-cultured on PDA. The colony forming units (CFUs) of bacteria and fungi were counted on each Petri plate and reported per 100 μl of water extract.
2.6. Soil dehydrogenase activity
Soil dehydrogenase activity was calculated by the reduction of triphenyl tetrazolium chloride (TTC) to triphenyl formazan (TPF) in the soil containing different compounds. In this study, 1 g of each soil sample was incubated for 24 h at 37 °C in 1 ml of TTC solution (5 g L−1 in 0.2 mol L−1 Tris–HCl buffer, pH 7.4). One drop of concentrated H2SO4 was added, and the samples was then blended with 1 ml of toluene to extract TPF and shaken for 30 min at 250 rpm (25 °C), followed by centrifugation at 5000 rpm for 5 min. The supernatant was removed, and absorbance in the extract was measured at 492 nm (Beckman 7200 Spectrophotometer). Soil dehydrogenase activity was then reported as μg TPF g−1 dry soil.[Citation18]
2.7. Computational method
Computational chemistry is a branch of chemistry that uses principles of computer science to assist in solving chemical problems. It uses the results of theoretical chemistry, incorporated into efficient computer programs to calculate the structures and properties of molecules. Examples of such properties are structure (i.e. the expected positions of the constituent atoms), absolute, and relative (interaction) energies, electronic charge distributions, dipoles and higher multiple moments, vibrational frequencies, reactivity, or other spectroscopic quantities, and cross sections for collision with other particles.[Citation17]
In this study, using computational methods, especially DFT method and basic functions theory including 6-311G** method, were studied for some properties such as IR spectroscopy, heat resistance and geometric and electronic structures, and the corresponding energies (HOMO, LUMO, and ΔEgap) of the novel synthetic nontoxic diphenolic monomer derived from D-4-HO-PhGlyOH and toxic industrial BPA were compered. At first, we optimized BPA and N,N′-PMB- D-4-HO-PhGlyOMe molecule structures using B3LYP method and 6-31G, 6-311G** basis site and subsequently the energy of two molecules were optimized. Then, we used optimum molecules to calculate IR, heat resistance, and thermal resistance of two molecules and compared it with experimental results.
3. Results and discussion
3.1. Monomer synthesis
N,N′-PMB- D-4-HO-PhGlyOMe (5) was manufactured as shown in Scheme . Thus, D-4-HO-PhGlyOH (1) was added to a methanol solution having thionyl chloride to obtain off white, powdery, D-PhGlyOMe.HCl (2) which deprotected with TEA to obtain D-PhGlyOMe (3). Reaction of compound 3 with pyromellitic dianhydride gives new optically active diphenolic monomer 5 in high yield. The progress of the reaction was monitored by TLC with cyclohexane, ethyl acetate (1:1) as the eluting solvent and visualized under UV light. The chemical structure of the compounds 2, 3, 5 were proved using elemental analysis, FT-IR, 1H-NMR, 13C-NMR spectroscopic techniques. The FT-IR spectrum of compound 5 showed a broad and strong peak at 3468 cm−1, which was assigned to the OH group of phenolic diol and two absorption bands at 1775 and 1725 cm−1 due to the symmetrical and asymmetrical imides-carbonyl stretching vibrations (Figure (a)). The 1H-NMR and 13C-NMR spectra (400 MHz) of compounds 2 and 3 showed good agreement with their structures (Figure ).
Figure 2. 1H-NMR (400 MHz) spectrum of compounds (a) 2, (c) 3, and 13C-NMR (125 MHz) spectrum of compounds (b) 2 and (d) 3 in DMSO-d6 at RT.
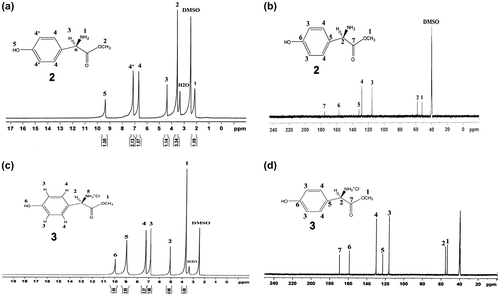
1H-NMR spectrum of diol 5 is shown in Figure . The 1H-NMR of this diol showed the characteristic resonance of C–H chiral center as singlet at 5.48 ppm; peak at 3.68 ppm as a singlet was assigned to the CH3 protons of methoxy groups. Singlet peak at 9.26 ppm was assigned to the two phenolic O–H. Moreover, 13C-NMR spectrum of monomer 5 (Figure ) showed CH chiral center carbon at 53.61 ppm. In addition, the presence of five types of different aromatic carbons and three carbons for carbonyl groups in this spectrum confirmed the structure of monomer 5. The chemical structure of diol 5 was also verified from the elemental analysis results, and the data corresponded well with the calculated values.
3.2. Polymer synthesis
The polymerization reactions of diol (5) with diisocyanates were performed under conventional heating techniques in the presence of Et3 N catalyst to furnish PUI7a–PUI7c (Scheme ). In this method, the reaction mixture was heated gradually from R.T up to 120 °C. The resulting polymers were obtained in good yields and inherent viscosities. Under graduate heating conditions, the polymerization reactions between diol 5 and different diisocyanates such as hexamethylene diisocyanate (HDI), toluylene-2,4-diisocyanate (TDI), and methylene diisocyanate (MDI) were also repeated in NMP in the presence of Et3 N . The reaction yields and some physical data are listed in Table .
Table 1. Physical properties of PUI7a–PUI7c.
3.3. Polymer characterization
The obtained polymers were characterized by FT-IR, 1H-NMR spectroscopy techniques, and elemental analyses. The FT-IR spectra of resulting PUIs showed the presence of the characteristic peaks for carbamate carbonyl, imide functions, and the absence of the original peaks arising from the OH and NCO groups in the corresponding diol and diisocyanate precursors. Absorption of urethane N–H bonds appeared around 3323–3521 cm−1 (hydrogen band) and the peak at 1590–1650 cm−1 confirmed the presence of urethane group. No isocyanate absorption was observed at 2260 cm−l. All of the PUIs exhibited absorption at 1370–1380 and 715–725 cm−1 that show the presence of the imide heterocycle in the polymers. Elemental analysis data of the resulting polymers are also in good agreement with calculated values of carbon, hydrogen, and nitrogen in the polymers (Table ). The 1H-NMR spectrum (500 MHz) of PUI7a is shown in Figure . In the 1H-NMR spectrum of PUI7a, appearances of the N–H protons of urethane group at 9.17 ppm as single peak indicate the presence of urethane group in the polymers main chain. The resonance peaks of aromatic protons appeared in the range of 6.54–8.25 ppm. The resonance of the O–CH3 proton groups appeared as a sharp single peak at 3.69 ppm.
Table 2. Elemental analysis of PUI7a and PUI7c.
3.4. Thermal properties
The thermal properties of some PUIs were evaluated by means of thermal gravimetric analysis (TGA). Thermal stability of the polymers was studied based on 5 and 10% weight loss (T5, T10) of the polymers. The thermo-analysis data of these polymers are summarized in Table . According to Table , it can be concluded that the resulting polymers are thermally stable. The differential scanning calorimetry (DSC) technique was used to determine the Tg of the polymers. The heating rate was kept at 20 °C/ min under a flow of nitrogen.
Table 3. Thermal behavior of PUI7a and PUI7c.
3.5. Solubility of PUI7s
The solubility of PUI7a–PUI7c was tested quantitatively in various solvents. The solubility properties of PUIs were studied in different solvents. The presence of ester groups in the side chain as well as main chain (as pendant and polar groups) in obtained polymers gives an effective way for improving solubility in common solvents. Polymers are soluble in organic polar solvents, such as DMF, N-methyl pyrolidine (NMP), and in sulfuric acid at R.T and are insoluble in solvents such as tetrahyrofuran, cyclohexane, chloroform, methylene chloride, and acetone. These polymers showed good solubility (Table ).
Table 4. Solubility properties of PUIs.Table Footnotea
3.6. Computational method
The optimized molecule structures of BPA and N,N′-PMB- D-4-HO-PhGlyOMe calculated using of B3LYP method and 6-31G, 6-311G** basis set in accordance with the atom labeling scheme is shown in Figure (a) and (b), respectively. The optimized energies of two molecules were listed in Table . Then we used optimum molecules to calculate IR, heat resistance and thermal resistance of two molecules by B3LYP method and 6-311G** bases site and compared with experimental results. The vibrational analysis of BPA and diol 5 were performed based on the characteristic vibrations of hydroxyl groups and aromatic rings. The vibrational band assignments of diol 5 by B3LYP with 6-31G, 6-311G** basis set are presented in Figure (b). The hydroxyl stretching vibrations are generally observed in the region around 3600 cm−1 [Citation19] (Figure (a)) in agreement with calculated hydroxyl stretching vibration by B3LYP with 6-31G, 6-311G** basis set . The real peak is broad and its intensity is higher than that of a free O–H vibration (calculated by DFT theory), which indicates the involvement of an intra-molecular hydrogen bond . The FT-IR spectrum in the high wave number region shows a broad band at 3499 and 3489 cm−1 attributed to hydrogen bonded O–H stretching vibration. The in-plane O–H deformation vibration usually appears as strong band in the region of 1260–1440 cm−1 in the spectrum, which is shifted to higher wave number in the presence of hydrogen bonding. Susi and Ard [Citation20] identified the C=O stretching mode at 1720 and 1775 cm−1. Referring to the above findings and on the basis of the results of the normal coordinate analysis, the IR bands appeared at 1725 and 1775 cm−1 which have been designated to C=O stretching modes for diol 5 in this study. The aromatic structure shows the presence of C–H stretching vibrations in the region 3069, 3087, 3110 and 3159 cm−1, which is the characteristics region for the ready identification of carbon–hydrogen stretching vibrations which is in good agreement with calculated data of C–H stretching vibrations.[Citation21] The four in-plane bending vibrations appear in the range of 1000–1300 cm−1 in the substituted benzenes, and four out-of-plane vibrations occur in the frequency range of 700–1000 cm−1.[Citation22] The comparison between theoretical and experimental results of corresponding energies such as HOMO, LUMO, ΔEgap, heat resistance, and thermal stability showed that the novel diol 5 have better properties than BPA (Table ).
Table 5. Total energies of BPA and Diol 5 calculated at HF and B3LYP with 6-31G, 6-311G** level of theory (At temperature of 500 K and pressure of 1 atm).
The HOMO–LUMO energy gap refers to the potential energy difference between the highest occupied molecular orbital (HOMO) and the lowest unoccupied molecular orbital (LUMO). Basically, it is how much energy has to be fed into the molecule to kick it from the ground (most stable) state into an excited state. The HOMO–LUMO energy gap of BPA and diol 5 were calculated at the B3LYP/6-311G** level and the energy gap reflects the chemical activity of the molecule. The LUMO as an acceptor represents the ability to obtain an electron, and HOMO represents the ability to donate an electron. The frontier orbitals of BPA and diol 5 are shown in Figure . The electronic transition corresponds to the transition from the ground to the first excited state, which is mainly described by an electron excitation from the highest molecular orbital (HOMO) and the lowest unoccupied molecular orbital (LUMO). The HOMO and LUMO energies of BPA and diol 5 calculated by the DFT level are listed in Table . The HOMO–LUMO energy gap of diol 5 and BPA are −0.169055 and −0.11462, respectively. The energy gap of HOMO–LUMO explains the eventual charge transfer interaction within the molecule which influences the biological activity of the molecule and also energy serves as a measure of the excitability of a molecule. If the energy gap is smaller, then the molecule will be excited easily. It could be concluded that diol 5 is more stable than BPA.
3.7. Fungal and bacterial biodegradation
Results of counting fungal and bacterial colonies showed that the highest number of bacterial colonies was counted from water extracts of soil containing compound 3 (Table ). It could be concluded that this compound is more biologically active than other compounds. This can be related to simpler and smaller structure of this compound which makes nitrogen more available for the growth of microorganism. Interestingly, there was significant negative correlation between the presence of high bacterial colonies and the absence of fungal colonies in compounds 2 and 3 (Figure ). It is being reported that intense bacterial competition could deprive the fungi of their energy sources and could result in a decrease in fungal diversity and population.[Citation23] It seems that compounds 2 and 3 have more attraction for bacterial degradation; however, in compounds 5, the degradation may proceed by both bacterial and fungal colonies (Table ). Results of counting fungal and bacterial colonies also showed that the number of colonies from water extracts of soil containing novel diphenolic monomer (5) derived from D-4-hydroxyphenylglycine moiety are higher than BPA. Based on this result, it could be concluded that this compound is more biologically active than BPA. This can be related to presence of nitrogen atom in the structure of diol 5 compared to BPA which makes nitrogen more available for the growth of microorganism.[Citation24]
Table 6. Dehydrogenase activities (mean ± SE) in soil containing different compounds after 100 days of soil burial test (μg TPF g−1 dry soil). Also the number (±Standard deviation) of bacterial and fungal colony forming units (CFUs) in water extract of soil containing 2, 3, 5, BPA, and PUI7a.
Regarding to biodegradability of diol 5, we also expected synthesized polymers to be biodegradable. This is in agreement with the structure of synthesized PUIs, because these types of polymers contain naturally occurring amino acids containing nitrogen atom in the backbone which is needed for the growth of microorganisms (Figure , Table ). Diphenolic monomer 5 and compound 3 were disappeared up to 50% after 1 month, and it was almost disappeared under experimental condition after 2 months in soil. In contrast, PUI7a was not completely disappeared in the soil. This observation shows that diol 5 is biologically active and biodegradable under soil burial more than BPA. It is also concluded the novel diol 5 may be employed as a replacement for industrially used diphenols such as BPA in the design of biodegradable and biological materials.
3.8. Dehydrogenase activity
After 100 days of soil burial test, the dehydrogenase activity was 24.75 and 7.68 μgTPFg−1 of dry soil in soil containing diol 5 and BPA, respectively (Table ). The dehydrogenase activities of treated soils were three times higher than control soil and the soil containing BPA as a reference, indicating that the abundance and activities of the microbial communities in the treated soils were enhanced by the presence of 2,3, diol 5, and obtained polymers (Table ). This may indicate that our synthetic polymer and their intermediates might serve as a carbon source for microbial growth and this will result in biodegradability of polymers and higher dehydrogenase activities.
4. Conclusions
This work showed that N,N′-(Pyromellitoyl)-Bis-D-4-hydroxyphenylglycine dimethyl ester (5) is an interesting diphenolic monomer containing d-amino acid and can be regarded as a replacement for industrially used diphenols such as BPA in the design of biodegradable and biological materials. DFT with B3LYP functional and the 6-31G basis set showed that the properties such as the geometric and electronic structures, the corresponding energies (HOMO, LUMO, and △Egap), heat resistance, and thermal stability of novel diphenolic monomer are better than BPA. Novel biologically active PUIs were synthesized via step growth polymerization of a nontoxic diphenolic precursor and different diisocyanate under conventional polycondensation reactions. The chemical structure of the diol 5 and resulted PUIs were determined by detailed analyses with FTIR, 13C-NMR, 1H-NMR spectroscopy, and elemental analysis. All polymers had high solubility in various polar solvents while maintaining good thermal stability. TGA and DSC analyses revealed that the polymers are potentially thermally processable at lower temperatures. To make sure real bioactivity and safe incorporation of these materials in the geochemical life cycle, soil burial and in vitro toxicity tests were performed. The results illustrated that synthesized polymers could be decomposed by soil microorganisms and, therefore, these materials could be classified as environmentally friendly polymers.
Acknowledgement
We wish to express our gratitude to the Research Affairs Division, Islamic Azad University, Shahrekord Branch, for partial financial support. Further financial support from Young Researchers and Elites Club is gratefully acknowledged.
References
- Chen MY, Ike M, Fujita M. Acute toxicity, mutagenicity, and estrogenicity of bisphenol-A and other bisphenols. Environ. Toxicol. 2002;17:80–86.10.1002/(ISSN)1522-7278
- Saal FSV, Akingbemi BT, Belcher SM, Birnbaum LS, Crain DA, Eriksen M, Farabollini F, Guillette LJ, Hauser R, Heindel JJ, Ho SM, Hunt PA, Iguchi T, Jobling S, Kanno1 J, Keri1 RA, Knudsen KE, Laufer H, LeBlanc GA, Marcus M, McLachlan JA, Myers JP, Nadal A, Newbold RR, Olea N, Prins GS, Richter CA, Rubin BS, Sonnenschein C, Soto AM, Talsness CE, Vandenbergh JG, Vandenberg LN, Walser-Kuntz DR, Watson CS, Welshons WV, Wetherill Y, Zoeller RT. Chapel hill bisphenol a expert panel consensus statement: integration of mechanisms, effects in animals and potential to impact human health at current levels of exposure. Reprod. Toxicol. 2007;24:131–138.
- Vandenberg LN, Hauser R, Marcus M, Olea N, Welshons WV. Human exposure to bisphenol A (BPA). Reprod. Toxicol. 2007;24:139–177.10.1016/j.reprotox.2007.07.010
- Nakagawa Y, Tayama S. Metabolism and cytotoxicity of bisphenol a and other bisphenols in isolated rat hepatocytes. Arch. Toxicol. 2000;74:99–105.10.1007/s002040050659
- Tsai WT. Human health risk on environmental exposure to bisphenol-a: a review. J. Environ. Sci. Health., Part A. 2006;24:225–255.10.1080/10590500600936482
- Pulapura S, Kohan J. Tyrosine-derived polycarbonates: backbone-modified pseudo-poly (amino acids) designed for biomedical applications. Biopolymers. 1992;32:411–417.10.1002/(ISSN)1097-0282
- Parth NS, Rachel LM, Stephanie TL, Yang HY. Electrospinning of L-tyrosine polyurethanes for potential biomedical applications. Polymer. 2009;50:2281–2289.
- Mallakpour S, Tirgir F, Sabzalian MR. Synthesis, characterization and in vitro antimicrobial and biodegradability study of pseudo-poly(amino acid)s derived from N,N′-(pyromellitoyl)-bis-l-tyrosine dimethyl ester as a chiral bioactive diphenolic monomer. Amino Acids. 2011;40:611–621.10.1007/s00726-010-0686-0
- Aamer KA, Genson KL, Kohn J, Becker ML. Impact of polymer-bound iodine on fibronectin adsorption and osteoblast cell morphology in radiopaque medical polymers: tyrosine-derived polycarbonate blends as a model system. Biomacromolecules. 2009;10:2418–2426.10.1021/bm900327b
- Tsume Y, Incecayir T, Song X, Hilfinger JM, Amidon GL. The development of orally administrable gemcitabine prodrugs with d-enantiomer amino acids: enhanced membrane permeability and enzymatic stability. Eur. J. Pharm. Biopharm. 2014;86:514–523.10.1016/j.ejpb.2013.12.009
- Rodríguez SM, Martinez-Gomez AI, Rodriguez-Vico F, Clemente-Jimenez JM, Heras-Vazquez FJL. Natural occurrence and industrial applications of d-amino acids: an overview. Chem. Biodivers. 2010;7:1531–1548.10.1002/cbdv.200900245
- Hou J, Liu Y, Qiang Li, Yang J High activity expression of d-amino acid oxidase in Escherichia coli by the protein expression rate optimization. Protein Expression Purif. 2013;88:120–126.10.1016/j.pep.2012.11.020
- Huang J, Chen X, Guo C, WangYX. Contributions of spinal d-amino acid oxidase to bone cancer pain. Amino Acids. 2012;43:1905–1918.10.1007/s00726-012-1390-z
- Basso A, Braiuca P, Martin LD, Ebert C, Gardossi L, Lindada P. Phenylglycine and d-4-hydroxyphenylglycine methyl esters via penicillin G acylase catalysed resolution in organic solvents. Tetrahedron. 2000;11:1789–1796.10.1016/S0957-4166(00)00129-4
- Kim GJ, Kim HS. Optimization of the enzymatic synthesis of d-p-hydroxyphenylglycine from dl-5-substituted hydantoin using d-hydantoinase and N-carbamoylase. Enzyme Microb. Technol. 1995;17:63–67.10.1016/0141-0229(94)00040-X
- Jeyavijayan S, Arivazhagan M. FTIR and FT-Raman spectra, molecular geometry, vibrational assignments, first-order hyperpolarizability, ab initio and DFT calculations for 3,4-dimethoxybenzonitrile. Spectrochim. Acta, Part A. 2011;81:466–474.10.1016/j.saa.2011.06.039
- Xavier RJ, Raj SA. Ab initio, density functional computations, FT-IR, FT-Raman and molecular geometry of 4-morpholine carbonitrile. Spectrochim. Acta, Part A. 2013;101:148–155.10.1016/j.saa.2012.09.081
- Kumar S, Chaudhuri S, Maiti SK. Soil dehydrogenase enzyme activity in natural and mine soil – A review. Middle-East J. Sci. Res. 2013;13:898–906.
- Colthup NB, Daly LH, Wiberley SE. Introduction to infrared and Raman spectroscopy. New York (NY): Academic Press; 1990.
- Susi H, Ard JS. Planar valence force constants and assignments for pyrimidine derivatives. Spectrochim. Acta, Part A. 1971;27:1549–1562.10.1016/0584-8539(71)80211-8
- Varyansi, G Assignment for vibrational spectra of seven hundred benzene derivatives. Vols. 1–2, Budapest: AcatemicKiaclo; 1973.
- Socrates G. Infrared and Raman characteristic frequencies. 3rd ed. Chichester: Wiley; 2001.
- de Boer W, Folman LB, Summerbell RC, Boddy L. Living in a fungal world: impact of fungi on soil bacterial niche development. FEMS Microbiol. Rev. 2005;29:795–811.10.1016/j.femsre.2004.11.005
- Mallakpour S, Asadi P, Sabzalian MR. Synthesis of biodegradable chiral poly(ester-imide)s derived from valine-, leucine- and tyrosine-containing monomers. Amino Acids. 2011;41:1215–1222.10.1007/s00726-010-0799-5