Abstract
The production of size-tunable Carbon microspheres (CMSs) from cheaply available materials using an environmentally friendly technique is highly appreciated. In this study, size-tunable CMSs were hydrothermally synthesized at 190°C using sucrose as carbon source, and citric acid as a catalyst. The effect of varying citric acid concentration on the size of the microspheres was investigated. Results indicated that under similar hydrothermal conditions, variation in the concentration of citric acid between 0 and 5 wt.% increased the size of CMSs ranging from 3.12 to 11.2 µm, as evidenced by SEM and particle size analyzer. TGA confirmed the purity of the carbonaceous particles in a single-step degradation with the presence of D-band and G-band in Raman spectra. FTIR and elemental analyzer confirmed the presence of hydrophilic oxygen functionalities such as –OH, –C=O, and COOH on the surface of CMSs. This study opens a novel and straightforward approach to produce size-tunable CMSs with functional groups.
1. Introduction
Among various forms of carbon materials, carbon microspheres (CMSs) have attracted substantial attention due to their particular physical properties, including minimal surface energies, controllable sizes, and diverse morphologies (hollow, hard-spherules, and beads) [Citation1]. The CMSs have been used for various applications such as hydrogen storage [Citation2], fuel cells [Citation3], catalysts [Citation4], adsorbents [Citation5], supercapacitors [Citation6] and drug delivery [Citation7] due to their remarkable chemical stability, high thermal conductivity, excellent heat resistance, electrical conductivity, and self-sintering properties [Citation8].
By taking these outstanding characteristics into account, a range of methods has been used to prepare the CMSs, including pyrolysis, chemical vapour deposition, and hydrothermal treatment [Citation9–11]. The hydrothermal treatment of CMSs has recently received a great deal of attention due to its moderate reaction conditions, one-step synthetic procedure, low cost, and environmental friendliness [Citation12]. The main advantage of the hydrothermal method is the high efficiency that can be achieved at mild temperature (< 300°C) inside a sealed autoclave where there is no need to use any hazardous solvents [Citation13,Citation14]. Therefore, spherical carbons with many surface oxygen groups (mainly -C = O and -OH) can be produced under the high pressure, and relatively moderate temperature exists in a hydrothermal chamber [Citation15,Citation16]. Moreover, by altering the temperature and composition, morphology (shape and diameter) and chemical functionalities of synthesized carbon could be simply controlled to comply with various applications [Citation17].
Many studies have shown the versatility of hydrothermal carbon synthesis. It has been found that the size of CMSs can be controlled by changing the type and concentration of starting materials and hydrothermal conditions [Citation18,Citation19]. Various types of mono-, di-, and polysaccharides, cellulosic carbohydrates, biopolymers, and biomass including glucose [Citation20], fructose [Citation21], sucrose [Citation22], cellulose [Citation23], starch [Citation24], yogurt [Citation13] and chitosan [Citation25] have been used as carbon precursors. The studies revealed that the diameter of CMSs by far relies on the type of saccharides used as precursors. Sevilla et al. [Citation19] found that the hydrothermal carbonization of sucrose and starch resulted in CMSs with larger diameters thanglucose under the same experimental condition, which was attributed to their capability to generate a more significant number of decomposed species during the hydrothermal treatment. A study by Krstic et al. [Citation26] indicated that the diameter of CMSs obtained by hydrothermal carbonization of fructose could be modulated by modifying the fructose concentration in solution. It was found that increasing the concentration of fructose leads to an increase in the mean diameter of the CMSs anddecreases the regularity of spherical particles.
Another main factor affecting the size of the CMSs is the pH of the solution. Zha et al. [Citation12] prepared CMSs from sucrose using hydrothermal carbonization and evaluated the effect of different pH values (0.1,1, 3, 5,7,10,12, and 14) on physicochemical properties of CMSs by adding hydrochloric acid (HCl) or sodium hydroxide (NaOH). The results revealed that while under acidic conditions, the particle size of CMSs appeared to be smaller, at alkaline pH, the size of CMSs was grown. Acidic and alkaline substances have also been used to create porous CMSs. Very recently, Zhao et al. reported synthesis of monodispersed cron-grade carbon spheres by hydrothermal carbonization of maltose in the presence of HCl as the catalyst and polyacrylic acid as dispersant [Citation27]. The porous structure was created by Hydrogen peroxide (H2O2) oxidation and subsequent calcination under air. The resultant CMSs were covalently quaternized with two layers of hyperbranched polymers by the amidation and repeated ring-opening reaction of epoxy and demonstrated high efficiency as anion exchangers for Ion chromatography (IC). Another study also revealed that carbon microspheres with contrallable pore size could be produced from waste Camellia Oleifera shells with a two-step process, namely hydrothermal carbonization and annealing [Citation28]. For creating a highly porous structure, potassium hydroxide (KOH) was impregnated onto CMSs and subjected to thermal treatment at 650°C. While the impregnation of CMSs with KOH, resulted in the formation of a predominantly mesoporous structure, the CMSs, which were mixed and ground with KOH exhibited only microporous structure. The CMSs showed a high potential for quick removal of phenolic organic pollutants from water.
Nevertheless, to date, there has been little attention paid to the effect of acid catalysis on tuning the size of the CMSs.
Citric acid as a potential catalyst for hydrothermal carbonization is expected to have a significant influence on the morphology and sphere size distribution. In a study by Zhao et al. [Citation13], citric acid was used to catalyze the CMSs derived from cellulose. It was found that the size of the CMSs increased with increasing citric acid concentration, reaction time and temperature. To the best of our knowledge, no study has been done evaluating the effect of citric acid on hydrothermal carbonization of sucrose to produce the CMSs.
This work investigates the catalytic effect of citric acid on synthesizing the CMSs from sucrose as a carbon precursor. The size and morphology of the synthesized CMSs were studied by changing the acid to precursor concentrations. The synthesized CMSs were characterized by using scanning electron microscopy (SEM), particle size analyzer, Fourier Transform Infra-Red spectroscopy (FTIR), Raman spectra, and elemental CHNS analyzer.
2. Materials and methods
2.1. Materials
Sucrose was purchased from a local supermarket (Prai, Malaysia), and food-grade anhydrous citric acid (Merck, 99%) was obtained from Bake With Yen Sdn. Bhd. (Malaysia). All materials were used without further purification.
2.2. Synthesis of the CMSs
Sucrose solution (15 wt.%) was prepared by dissolving sucrose in distilled water. Citric acid solutions of 1.25 to 5 wt.% were also prepared in distilled water. The sucrose solution was mixed with citric acid solutions at a weight ratio of 1:1 and magnetically stirred for 1 h before hydrothermal reaction. Five samples were prepared by varying citric acid concentrations of 0, 1.25, 2, 3.75, and 5 wt.% (hereafters called Su-CA-0, Su-CA-1.25, Su-CA-2, Su-CA-3.75, Su-CA-5). The prepared solutions were transferred into a 100 ml Teflon-lined stainless-steel autoclave and sealed tightly. The reactor was placed into a preheated oven for 6 h at 190°C and was then cooled naturally to room temperature. The slurry formed inside the Teflon liner was then washed three times with water followed by ethanol until the liquid residues turned colourless. The wet slurry was then dried in an oven at 60°C for 24 h to obtain the powder product. Figure illustrates the steps of preparation of the CMSs using the hydrothermal treatment.
2.3. Characterization of CMS powder
The microstructure of the CMSs was assessed using an SEM (Hitachi S3400N-II-Japan) at an acceleration voltage of 5 kV. Before conducting microstructural analysis, the samples were subjected to gold coating using a sputter coater (Quorum Q150R S, UK). The particle size distribution of the CMSs was measured by a laser diffraction technique in a Mastersizer 3000 (Malvern, UK). The powders were dispersed into ethanol and stirred at 700 rpm using Hydro EV accessories. Particle size distribution graphs were then obtained by plotting number density versus size. The textural characterization of the CMSs was measured using an N2 adsorption–desorption isotherm at 77 K with an ASAP 2020 instrument (Micromeritics, USA). The samples were outgassed at 200°C for 5 h. Surface areas were calculated by Brunauer Emmett–Teller (BET) method from N2 adsorption isotherms. The samples were examined using an X-ray diffractometer (XRD, Bruker D8 Advance TXS, Germany) at 40 kV and 40 mA current using Cu-Ka radiation. X-ray diffraction patterns were obtained at room temperature over the 2θ range from 5° to 80°. The effect of temperature on the CMSs was studied using a thermo-gravimetric analyzer (TGA Q-50, TA Instruments, USA). The samples were analyzed at a heating rate of 5°C/min between 25°C and 800°C in air atmosphere. FTIR test was performed to determine the chemical compositions and functional groups of the CMSs. The FTIR spectra were obtained in attenuated total reflectance mode (ATR-FTIR400, Perkin Elmer Instruments, USA) in the wavelength range of 4000 to 500 cm−1 (recorded at 0.4 cm−1 resolution and average of 64 scans) under transmittance mode using ATR module. Raman spectroscopy of the CMSs was recorded over the range of 3000 to 300 cm−1 using a Raman Spectrometer (LabRam HR Evolution, Japan). Raman spectra of the samples were obtained using a frequency-doubled Nd.VO4 diode-pumped solid-state laser with excitation of 532 nm. CHNS analysis was carried on the samples to determine basic elements (carbon, hydrogen, and Oxygen) containing in the CMSs. The test was conducted using the CHNS-932 elemental analyzer (LECO, USA) based on the test method.
3. Result and discussion
3.1. Physicochemical characteristics of CMSs
The microstructure of the synthesized CMSs has shown in Figure . The SEM illustrates that all the CMSs are in perfect spherical morphologies. The images show that the spheres are well-dispersed and have a high degree of uniformity. The comparison of SEM images revealed that the average particle size of the CMSs enhanced by increasing the citric acid concentration. These results were in good agreement with those reported by Liu et al. [Citation29], who found that larger CMSs can be obtained by increasing oxalic acid concentration in glucose solution.
Figure 2. SEM images of the CMSs. (a) Su-CA-0; (b) Su-CA-1.25; (c) Su-CA-2; (d) Su-CA-3.75 and (e) Su-CA-5.
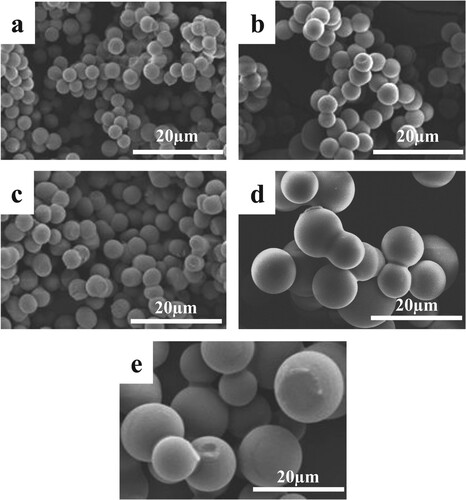
The particle size analyzer determined the average size and the size distribution of the CMSs prepared using various citric acid concentrations. Based on the size distribution results presented in Figure (a), sample Su-CA-0 without citric acid resulted in spheres with mean diameters around 3 µm, whereas samples synthesized in the presence of citric acid have larger mean diameters, comparatively. The average particle sizes increased from about 3 to 11 µm as the concentration of citric acid increased in the solution, which is consistent with the SEM results. The addition of citric acid to the sucrose solution led to the CMSs with slightly broader size distributions. A more significant particle size distribution obtained by the addition of citric acid could be due to the extra hydronium ions provided by citric acid, which catalyzes the hydrolysis of sucrose to fructose and glucose. Moreover, citric acid itself contains carbon molecules that could contribute to the nucleation of the CMSs. This result is consistent with the findings of Zhao et al. [Citation13].
Figure (b) displays the growth rate of spheres as a function of citric acid concentration. Su-CA-1.25 and Su-CA-3.75 were also synthesized to validate the size-controllability of the CMSs. Based on the results, particle sizes produced by Su-CA-1.25 and Su-CA-3.75 fit with an exponential trend line proposed on the study with a calculated marginal error of around 6%. The analyses of Figure suggest that the particle sizes of the CMSs can be controlled by altering the citric acid concentration.
Figure 3. (a) Particle size distribution of CMSs. (b) Average particle size as a function of citric acid concentration.
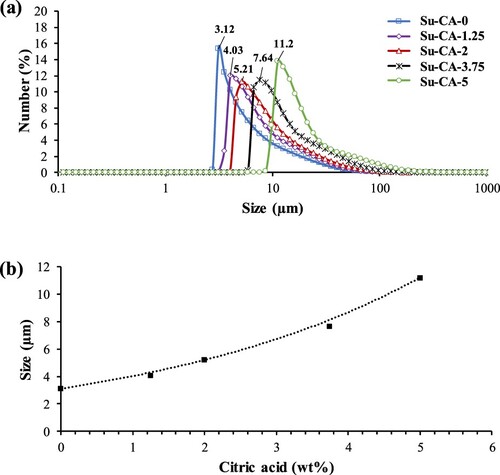
The specific surface area, pore-volume, and average pore diameter of the CMSs are listed in Table . The samples’ surface area was less than 3 m2g−1, which revealed that the synthesized microspheres have a reduced porosity. Similar results have been reported by Sevilla and Fuertes [Citation19]. Indeed, the reason for such a low surface area is that during the hydrothermal carbonization process, solubilization of organics and formation of tarry substances also arise so that these substances contaminate the hydrochars and plug their pores [Citation30]. Notably, the surface area, pore size, and pore volume of the CMSs decreased by increasing citric acid concentration, and it could be attributed to the enhancement in the particle size of the microspheres as indicated by SEM and particle size analysis.
Table 1. N2 adsorption/desorption parameters of the CMSs.
The XRD patterns of the two CMSs are demonstrated in Figure (a). The presence of two broad peaks at 2θ = 24.8° and 43.5° is as a result of reflections from the (002) plane and the (100) plane, respectively [Citation31]. These reflections can be indexed to the graphite characteristics of CMSs. The crystallinity structure was not detected due to the sharp peak’s absence suggesting that the CMSs are completely amorphous [Citation32]. These results show that all the samples are in amorphous form and containing aromatic structure.
TGA was used to investigate the thermal degradation behaviour of the CMSs synthesized with and without citric acid. Figure (b) indicates the degradation behaviours of Su-CA-0, Su-CA-2, and Su-CA-5. All samples showed a single-step degradation between 300°C and 550°C, revealing the characteristic of pure carbonaceous compound [Citation33]. A small weight loss of around 5% at the beginning of the curve (30–60°C) is probably due to the samples’ moisture loss. Synthesized CMSs were nearly completely degraded at temperature > 600°C. Therefore, the addition of citric acid does not change the degradation behaviour of carbon spheres.
The CMSs prepared via hydrothermal carbonization of sucrose were expected to show plenty of surface functional groups [Citation33]. For clarification, synthesized CMSs with and without citric acid have been analyzed under FTIR. As it can be seen in Figure (a), all the samples exhibited similar IR spectra. The broad bands at 3000–3700 cm−1 are attributed to -OH and -COOH functional groups. The peak at 1710 cm−1, is related to C = O stretching corresponding to carboxyl moieties [Citation34]. Peaks at 1470–970 cm−1 region are ascribed to C–O stretching or bending vibrations in hydroxyl, ester, or ether [Citation35]. The availability of these peaks suggests several oxygen groups (C = O and C–O) on the carbon sphere surface. The band also evidences the presence of aromatic rings at 1620 cm−1, assigned to C = C vibrations, and the bands in the 930–750 cm−1 region, attributed to aromatic C–H out-of-plane bending vibrations [Citation16,Citation36]. The spectra of CMSs also show a band at 3000–2850 cm−1, which assigned to the stretching vibrations of methylene groups confirming the presence of an aliphatic structure. All the bands comply with the characteristic of low temperature hydrothermal carbonaceous materials that exhibit vibrant oxygen surface groups (e.g. C–O and C = O). All synthesized samples additionally showed transmission Raman signal around 1000–2000 cm−1 range, as demonstrated in Figure (b), indicating the presence of carbonized materials with two distinct peaks, D-band at 1360 cm−1 and G-band at 1590 cm−1 [Citation37]. D-band is assigned to ring-breathing vibrations of A1g at Brillouin zone boundary K, and it is evidence for the presence of benzene or condensed benzene rings in amorphous carbon films.
Figure 5. (a) FTIR spectra of Su-CA-0, Su-CA-2, and Su-CA-5. (b) Raman spectra of CMSs in the range of 500–2500 cm−1.
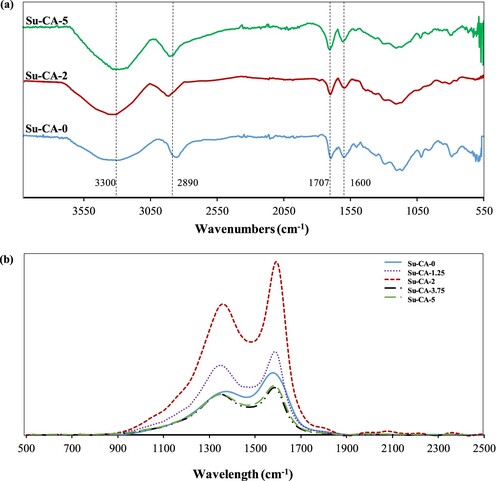
Moreover, the presence of D-band deduced the amorphous structure of the CMSs, which is in agreement with the XRD results. On the other hand, G-band shows in-plane bond-stretching motion pairs of C sp2 atoms in aromatic and olefinic molecules of amorphous carbon [Citation38]. Indeed, the degree of aromatic structure formation was affected by the amount of citric acid. The value of the relative intensity ratio (ID /IG) indicates the graphitization degree of carbon materials. In the present study, ID /IG increased from 0.651 for Su-CA-0 to 0.757 and 0.825 for Su-CA-2 and Su-CA-5, respectively. This result manifests that the graphitization degree has been increased with increment in citric acid concentration.
The increased intensity of these bonds could mean that higher amounts of aromatic groups have been formed in the CMS structure. The intensity of these peaks increases by rising citric acid concentration up to 2 wt.%. However, the addition of a higher concentration of citric acid (3.75 and 5 wt.%) resulted in a dramatic decrease in the intensity of the peaks. From this, it can be concluded that the samples prepared in the presence of a low concentration of citric acid contain more aromatic groups than those with higher citric acid concentrations.
CHNS analysis was conducted on the three samples,Su-CA-0, Su-CA-2, and Su-CA-5, and the results revealed that all the samples possess almost similar carbon, oxygen, and hydrogen contents. Table displays the elemental compositional of the CMSs. The spheres were found to contain about 61 wt.% carbon, 5 wt.% hydrogen, and 33 wt.% oxygen, implying incomplete dehydration of the sucrose. The higher oxygen content of Su-CA-2 and Su-CA-5 samples could be due to the interaction of acid functionalities of citric acid with hydroxyl groups of sucrose. The results are in good agreement with FTIR results, where the presence of oxygen-containing surface functional groups was also confirmed.
Table 2. The elemental components of CMSs.
3.2. The formation mechanism of CMSs
The proposed chemistry behind the formation of CMSs is illustrated in Figure . We assume that the mechanism of CMSs formation was initiated with the hydrolysis of sucrose to produce glucose and fructose. Hydrolysis of sucrose was made possible by hydronium ions generated by water autoionization [Citation39] and acid-catalyzed hydrolysis that provides even more hydronium ions [Citation40]. Disaccharides of sucrose were also hydrolyzed to form monomers that react with remaining H+ in the solution. In more acidic conditions, due to the increased amount of citric acid, the presence of H+ increases in the solution, leading to more intensified nucleation of the polysaccharide. The monomers are then polymerized by intermolecular dehydration to form soluble polymers [Citation41]. When the concentration of the polymers reached a critical supersaturation point, a short single nucleation burst occured, followed by further dehydration and aromatization [Citation42]. CMSs were found to have a polymer-like structure comprising of polyfuranic chains formed through polymerization or aromatization of furan-like molecules. Thus, the synthesized CMSs expected to have a core composed of hydrophobic polyfuran compounds, and a shell made up of hydrophilic functional groups such as carbonyl and carboxylic acid. It is expected that the addition of citric acid to the sucrose solution accelerates the hydrolysis process, which eventually caused the formation of CMSs with larger mean diameters as compared to pure sucrose.
4. Conclusion
The hydrothermal treatment of sucrose has successfully synthesized Size-controlled CMSs in the presence of various citric acid concentrations at a low temperature (190°C). The diameter of CMSs increased from about 3 µm to 11 µm by increasing citric acid concentration. The spherical morphology of CMSs was confirmed by SEM microscopy. All samples showed the pure characteristics of carbonaceous materials with single-stage degradation at a temperature range of 300–550°C. The obtained CMSs were contained a large number of oxygen-containing surface functional groups as deduced from FTIR and CHNS analyses. On the other hand, Raman spectroscopy confirmed the presence of amorphous and aromatic carbonaceous materials with two distinct peaks of D-band and G-band. More research is needed to study the formation mechanism and the influence of reaction temperature and time on the size of CMSs.
Acknowledgments
The author would like to gratefully acknowledge the Ministry of Science and Technology (MOSTI) for providing research funding under the e-Science scheme grant number 03-02-10-SF025. This research was also financially supported by the Ministry of Higher Education Malaysia (FRGS- FP076-2018A).
Disclosure statement
No potential conflict of interest was reported by the author(s).
Additional information
Funding
References
- Li M, Li W, Liu S. Hydrothermal synthesis, characterization, and KOH activation of carbon spheres from glucose. Carbohydr Res. 2011;346:999–1004. doi: 10.1016/j.carres.2011.03.020
- Ding XH, Chen SS, Liu J, et al. Synthesis of carbon molecular sieve microspheres via amino phenolic resin as precursor formed from in-situ reaction composite micelles. J Porous Mater. 2018;25:1373–1380. doi: 10.1007/s10934-017-0549-0
- Horri BA, Selomulya C, Wang H. Modeling the influence of carbon spheres on the porosity of SOFC anode materials. J Am Ceram Soc. 2012;95:1261–1268. doi: 10.1111/j.1551-2916.2011.05037.x
- Sánchez-Polo M, Von Gunten U, Rivera-Utrilla J. Efficiency of activated carbon to transform ozone into ·OH radicals: influence of operational parameters. Water Res. 2005;39:3189–3198. doi: 10.1016/j.watres.2005.05.026
- Yang K, Zhu L, Xing B. Adsorption of polycyclic aromatic hydrocarbons by carbon nanomaterials. Environ Sci Technol. 2006;40:1855–1861. doi: 10.1021/es052208w
- Liu J, Wang X, Gao J, et al. Hollow porous carbon spheres with hierarchical nanoarchitecture for application of the high performance supercapacitors. Electrochim Acta. 2016;211:183–192. doi: 10.1016/j.electacta.2016.05.217
- Dennis JS, Meng Q, Zheng R, et al. Carbon microspheres as network nodes in a novel biocompatible gel. Soft Matter. 2011;7:4170–4173. doi: 10.1039/c0sm01430c
- Cheng Y, Yang M, Fang C, et al. Controllable morphologies of carbon microspheres via green hydrothermal method using fructose and xylose. Chem Lett. 2017;46:1400–1402. doi: 10.1246/cl.170592
- Zhang M, Yang H, Liu Y, et al. Hydrophobic precipitation of carbonaceous spheres from fructose by a hydrothermal process. Carbon. 2012;50:2155–2161. doi: 10.1016/j.carbon.2012.01.024
- Yi Z, Liang Y, Lei X, et al. Low-temperature synthesis of nanosized disordered carbon spheres as an anode material for lithium ion batteries. Mater Lett. 2007;61:4199–4203. doi: 10.1016/j.matlet.2007.01.054
- Yang Y, Song J, Li Y, et al. Synthesis and optical property of P3HT/carbon microsphere composite film. J Mater Res. 2013;28:998–1003. doi: 10.1557/jmr.2012.363
- Zha G, Yu L. Influence of sucrose solution’s pH on hydrothermally synthesized carbon microspheres. Fuller Nanotub Car N. 2016;24:139–143. doi: 10.1080/1536383X.2015.1124419
- Zhao Y, Li W, Zhao X, et al. Carbon spheres obtained via citric acid catalysed hydrothermal carbonisation of cellulose. Mater Res Innov. 2013;17:546–551. doi: 10.1179/1433075X13Y.0000000108
- Zeimaran E, Pourshahrestani S, Shirazi SFS, et al. Hydrothermal synthesis and characterisation of bioactive glass-ceramic nanorods. J Non Cryst Solids. 2016;443:118–124. doi: 10.1016/j.jnoncrysol.2016.04.005
- Makowski P, Demir Cakan R, Antonietti M, et al. Selective partial hydrogenation of hydroxy aromatic derivatives with palladium nanoparticles supported on hydrophilic carbon. Chem Comm. 2008;8:999–1001. doi: 10.1039/b717928f
- Sun X, Li Y. Colloidal carbon spheres and their core/shell structures with noble-metal nanoparticles. Angew Chem Int Ed. 2004;43:597–601. doi: 10.1002/anie.200352386
- Sun X, Li Y. Ga2O3 and GaN semiconductor hollow spheres. Angew Chem Int Ed. 2004;43:3827–3831. doi: 10.1002/anie.200353212
- Falco C, Baccile N, Titirici MM. Morphological and structural differences between glucose, cellulose and lignocellulosic biomass derived hydrothermal carbons. Green Chem. 2011;13:3273–3281. doi: 10.1039/c1gc15742f
- Sevilla M, Fuertes AB. Chemical and structural properties of carbonaceous products obtained by hydrothermal carbonization of saccharides. Chem Eur. 2009;15:4195–4203. doi: 10.1002/chem.200802097
- Mi Y, Hu W, Dan Y, et al. Synthesis of carbon micro-spheres by a glucose hydrothermal method. Mater Lett. 2008;62:1194–1196. doi: 10.1016/j.matlet.2007.08.011
- Zhuang ZH, Yang ZG. Preparation and characterization of colloidal carbon sphere/rigid polyurethane foam composites. J Appl Polym Sci. 2009;114:3863–3869. doi: 10.1002/app.31064
- Zhao H, Lu X, Wang Y, et al. Effects of additives on sucrose-derived activated carbon microspheres synthesized by hydrothermal carbonization. J Mater Sci. 2017;52:10787–10799. doi: 10.1007/s10853-017-1258-4
- Sevilla M, Fuertes AB. The production of carbon materials by hydrothermal carbonization of cellulose. Carbon. 2009;47:2281–2289. doi: 10.1016/j.carbon.2009.04.026
- Haibo O, Cuiyan L, JianFeng H, et al. Synthesis of carbon/carbon composites by hydrothermal carbonization using starch as carbon source. RSC Adv. 2014;4:12586–12589. doi: 10.1039/C3RA47857B
- Simsir H, Eltugral N, Karagoz S. Hydrothermal carbonization for the preparation of hydrochars from glucose, cellulose, chitin, chitosan and wood chips via low-temperature and their characterization. Bioresour Technol. 2017;246:82–87. doi: 10.1016/j.biortech.2017.07.018
- Krstić S, Kaluđerović B, Dodevski V, et al. Structural properties of carbon microspheres obtained by hydrothermal treatment of fructose. Zaštita Materijala. 2015;56:155–158. doi: 10.5937/ZasMat1502155K
- Zhao Q, Tao S, Miao X, et al. A green, rapid, scalable and versatile hydrothermal strategy to fabricate monodisperse carbon spheres with tunable micrometer size and hierarchical porosity. Chem Eng J. 2019;372:1164–1173. doi: 10.1016/j.cej.2019.05.014
- Li K, Liu S, Shu T, et al. Fabrication of carbon microspheres with controllable porous structure by using waste camellia oleifera shells. Mater Chem Phys. 2016;181:518–528. doi: 10.1016/j.matchemphys.2016.06.089
- Liu J, Tian P, Ye J, et al. Hydrothermal synthesis of carbon microspheres from glucose: tuning sphere size by adding oxalic acid. Chem Lett. 2009;38:948–949. doi: 10.1246/cl.2009.948
- Elaigwu SE, Greenway GM. Chemical, structural and energy properties of hydrochars from microwave-assisted hydrothermal carbonization of glucose. Int J Ind Chem. 2016;7:449–456. doi: 10.1007/s40090-016-0081-0
- Tran HN, Lee CK, Nguyen TV, et al. Saccharide-derived microporous spherical biochar prepared from hydrothermal carbonization and different pyrolysis temperatures: synthesis, characterization, and application in water treatment. Environ Technol. 2017;39(21):1–14.
- Zheng M, Liu Y, Xiao Y, et al. An easy catalyst-free hydrothermal method to prepare monodisperse carbon microspheres on a large scale. J Phys Chem C. 2009;113:8455–8459. doi: 10.1021/jp811356a
- Joula MH, Farbod M. Synthesis of uniform and size-controllable carbon nanospheres by a simple hydrothermal method and fabrication of carbon nanosphere super-hydrophobic surface. Appl Surf Sci. 2015;347:535–540. doi: 10.1016/j.apsusc.2015.04.118
- Sun L. AgC core/shell structured nanoparticles: controlled synthesis, characterization, and assembly. Langmuir. 2005;21:6019–6024. doi: 10.1021/la050193+
- Kabyemela BM, Adschiri T, Malaluan RM, et al. Glucose and fructose decomposition in subcritical and supercritical water: detailed reaction pathway, mechanisms, and kinetics. Ind Eng Chem Res. 1999;38:2888–2895. doi: 10.1021/ie9806390
- Lua AC, Yang T. Effect of activation temperature on the textural and chemical properties of potassium hydroxide activated carbon prepared from pistachio-nut shell. J Colloid Interface Sci. 2004;274:594–601. doi: 10.1016/j.jcis.2003.10.001
- Cuesta A, Dhamelincourt P, Laureyns J, et al. Raman microprobe studies on carbon materials. Carbon. 1994;32:1523–1532. doi: 10.1016/0008-6223(94)90148-1
- Schwan J, Ulrich S, Batori V, et al. Raman spectroscopy on amorphous carbon films. J Appl Phys. 1996;80:440. doi: 10.1063/1.362745
- Tanger JC, Pitzer KS. Calculation of the ionization constant of H2O to 2,273 K and 500 MPa. AICHE J. 1989;35:1631–1638. doi: 10.1002/aic.690351007
- Dawber JG, Brown DR, Reed RA. Acid-catalyzed hydrolysis of sucrose: A student study of a reaction mechanism. J Chem Educ. 1966;43:34. doi: 10.1021/ed043p34
- Qi Y, Zhang M, Qi L, et al. Mechanism for the formation and growth of carbonaceous spheres from sucrose by hydrothermal carbonization. RSC Adv. 2016;6:20814–20823. doi: 10.1039/C5RA26725K
- Li M, Li W, Liu S. Control of the morphology and chemical properties of carbon spheres prepared from glucose by a hydrothermal method. J Mater Res. 2012;27:1117–1123. doi: 10.1557/jmr.2011.447