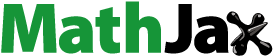
Abstract
Non-Newtonian fluids flow with mixed convection has got the researcher’s attention due to its widespread application in engineering and manufacturing systems. Furthermore, the thermal radiation in convective thermal transmission plays a key role in regulating the thermal transmission. That’s why the authors’ interest comes to the point to present a detailed analysis of the mixed convective flow of Casson and Oldroyd-B fluids through a linearly stratified stretching sheet. Also, the flow of Casson and Oldroyd-B fluids are considered as chemically reactive, thermally radiative, and magnetized by a strong magnetic field. The mathematical model of the present analysis is presented in PDEs (partial differential equations) format which is transformed into ODEs (ordinary differential equations) by employing suitable variables. The transformed ODEs are treated with an analytical technique called HAM (Homotopy analysis method) which provides an analytical solution for both linear and nonlinear differential equations. Through the use of diagrams, the converging exploration of HAM is depicted. An interesting result has been introduced that, the streamlines of Casson liquid are highly affected by a strong magnetic field as compared to Oldroyd-B liquid. The strong magnetic field and buoyancy ratio parameter moderate the velocity profile, while an opposite trend is found in the motion of liquid by using mixed convectional factors. Additionally, the impacts of magnetic and buoyancy ratio parameters are greater for Oldroyd-B fluid as associated with Casson liquid. On the other hand, the mixed convection parameter is stronger for Casson liquid as equated to Oldroyd-B fluid. The present results have a great agreement with previously published results.
Nomenclature
= | Components of velocity | |
= | Coordinate axes | |
= | Gravitational force | |
= | Coefficients of thermal and Concentration expansion | |
= | Casson parameter | |
= | Constants | |
= | Deborah numbers with respect to relaxation and retardation times | |
= | Nonlinear thermal radiation | |
= | Magnetic parameter | |
= | Prandtl number | |
= | Schmidt number | |
= | Thermophoresis | |
= | Chemical reaction parameter | |
= | Time | |
= | Dynamic viscosity | |
= | Relaxation time | |
= | Retardation time | |
= | Density | |
= | Kinematic viscosity | |
= | Electrical conductivity | |
= | Radiative heat flux | |
= | Coefficients of Brownian motion and thermophoresis | |
= | Thermal diffusivity | |
= | Temperature | |
= | Concentration | |
= | Reference temperature | |
= | Reference concentration | |
= | Free stream temperature | |
= | Free stream concentration | |
= | Temperature ratio parameter | |
= | Brownian motion | |
= | Thermal stratification parameter | |
= | Concentration stratification parameter |
1. Introduction
In recent years, there have been several experiments on mixed convectional transfer of non-Newtonian liquids via thermal and solutal stratifications. This is due to their widespread use in engineering and manufacturing systems. Heat release into the atmosphere such as rivers, oceans, lakes, and solar ponds are a few examples of such processes. The accumulation or formation of layers in a liquid happens as effect of thermal fluctuations, variations in concentration, or the interaction of various fluids. The analysis of thermal and concentration stratification of hydrogen and oxygen reservoirs is significant although it openly affects the rate of increase of all cultured species. The boundary layer flow and thermal transmission through an extending surface was initially investigated by Sakiadis [Citation1,Citation2]. Meanwhile different properties of liquid flows through an extending surface considering diverse physical circumstances were examined by many investigators. Tsou et al. [Citation3] presented the empirical and quantitative exploration of fluid motion through a moving sheet. Far ahead in 1970, Crane [Citation4] introduced the boundary layer flow concept and investigated the incompressible flow of liquid through a linear extending surface. Investigating the flow properties, the steady flow in an extending cylinder and extending channel was adopted by Brady and Acrivos [Citation5]. The stagnation point of dense liquid dynamics across an extending surface was investigated by Chaim [Citation6]. The impression if buoyancy forces on viscous liquid through an extending surface was presented by Chamkha [Citation7]. The stagnation movement of viscoelastic and viscid liquids past a stretched plate was explored by Mahapatra and Gupta [Citation8,Citation9]. The fluid flow of dime-dependent boundary layer over an extending flat surface has scrutinized by Nazar et al. [Citation10]. The thermal profiles of a steady fluid flow through an extending surface were offered by Ishak et al. [Citation11]. Furthermore, Ishak et al. [Citation12] examined the heat transmission of liquid motion through a stretchable sheet. Patil et al. [Citation13] presented the quadratic convective steam of power law liquid through a stretched surface. The numerical study of steady fluid motion through a stretched media has probed by Zaimi and Ishak [Citation14]. Further related works can be study in [Citation15–22].
In industrial developments where the value of the products is dependent on factors of thermal control, the thermal radiation shows an imperative character in regulating thermal transmission. Mat et al. [Citation23] presented the analysis of magnetic effects on nanofluid flow with thermal radiation consequence. Hady et al. [Citation24] considered the viscous nanofluid flow through a nonlinear extending sheet. The thermal transmission in a mixed convective liquid through an extending sheet was probed by Aldawody and Elbashbeshy [Citation25]. The stagnation point flow with thermal radiation consequence on a mixed convective flow was studied by Makinde [Citation26]. The influence of radiation on chemically reactive steam of fluid over an spreading surface was investigated by Kahar et al. [Citation27]. The effect of temperature transformation in a nanofluid over a stretchable surface has evaluated by Shankar and Ibrahim [Citation28]. Kumar et al. [Citation29] have explored Casson liquid motion over an expanding plate. Yusuf et al. [Citation30] addressed the entropy production in MHD Williamson motion over a permeable wall and impact of chemical interaction. Mabood et al. [Citation31] used asymmetric radiant heat and exergy destruction modelling to study the water-based Cu-Al2O3 hybrid nanofluid movement. Yusuf et al. [Citation32] investigated the 3D motion of Cu-TiO2/H2O hybrid nanoliquid through an extending medium. Ferdows et al. [Citation33] studied the H2O -based nanofluid flow under the influence of an applied magnetic field quantitatively. The H2O -based CNTs nanofluid across rotatory discs was studied by Mabood et al. [Citation34].
Krishna et al. [Citation35] examined the magnetohydrodynamic Casson hybrid nanofluid movement past an infinite exponentially stretching medium. Entropy production in micropolar liquid dynamics including slip condition was demonstrated by Yusuf et al. [Citation36]. Magnetohydrodynamics Oldroyd 8-constant flow pattern in a channel having irregular convective and chemical processes consequences was explored by Yusuf et al. [Citation37]. The entropy study in MHD couple stress flow of nanofluid including melt heat transmission, thermal radiation, and slip condition was presented by Mabood et al. [Citation38]. Ali et al. [Citation39] examined the magnetohydrodynamic Maxwell and tangent hyperbolic nanofluids flow through a bi-directional spreading medium. Yusuf et al. [Citation40] used activation energy to explore the Magnetohydrodynamic stagnation steam of Casson nanofluid. Krishna et al. [Citation41] presented the radiated MHD convectional nanofluids motion through a vertically extending surface. Mabood et al. [Citation42] examined the energy and mass transformation analysis of nanofluid flow over a rotating frame with MHD and entropy generation. Ahamad et al. [Citation43] analysed the Dufour and Soret influences on magnetohydrodynamic nanofluid motion across a semi-infinite sliding surface with thermal radiation and uniform energy source. Further related studies and mathematical methods can be analyse in [Citation44–53].
Motivated by the above literature survey, the authors have come into the point to present the heat and mass transmission in a flow of Casson and Oldroyd-B fluids through a linearly extending sheet. Also, the flow of Casson and Oldroyd-B fluids is considered as mixed convective, chemically reactive, thermally radiative, and highly magnetized by a strong magnetic field. Our focus is to present the comparative analysis of Casson and Oldroyd-B fluids via different embedded parameter. The present analysis is divided section wise as section 2 represents the problem formulation, section 3 displays the analytical solution, section 4 represents the convergence of HAM, and section 5 indicates the results and discussion. Section 6 includes the final comments. By the end of this study, the authors have to answer the following research questions:
How do the Casson and Oldroyd-B fluids behave against a magnetic field parameter and for which fluid does the magnetic parameter have a dominant impact?
How do the Casson and Oldroyd-B fluids behave against the buoyancy proportion and mixed convectional factors and for which fluid do the buoyancy ratio and mixed convective factors have a dominant impact?
How does the magnetic parameter affect the streamlines of the Casson and Oldroyd-B fluids flows?
2. Formulation of problem
Suppose the time independent, chemically reactive, and stratified flow of Casson and Oldroyd-B fluids through a linearly stretched surface. The velocity of extending surface is , where
denotes the stretching rate constant. Magnetic effect with strength
is pragmatic normal to the fluid flow.
denotes surface temperature and
signifies surface concentration of the fluids flow. The ambient temperature and concentration of the fluids flow are signified by
and
, respectively. Furthermore, the nonlinear thermal radiation, Brownian motion coefficient, thermophoresis coefficient, and mixed convection are also considered. The flow geometry of the modelled problem is exhibited in Figure .
The basic equations for Oldroyd-B fluid are:
(1)
(1)
(2)
(2) where
and
are defined as:
(3)
(3) The following is the rheological model for Casson fluid:
(4)
(4)
(5)
(5) Therefore, the leading equations are defined as [Citation54–56]:
(6)
(6)
(7)
(7)
(8)
(8)
(9)
(9) with boundary conditions [Citation57,Citation58]:
(10)
(10) The similarity variables are defined as [Citation58]:
(11)
(11) Continuity equation is obvious; momentum, energy, and concentration are reduced as:
(12)
(12)
(13)
(13)
(14)
(14) with transformed boundary conditions:
(15)
(15) where
(16)
(16) All of the above dimensional and non-dimensional parameters are defined in the nomenclature.
Physical quantities of interest and
, are defined as:
(17)
(17) Using the similarity variables defined in Equation (11), we have
(18)
(18) where
is signified the local Reynolds number.
3. HAM solution
Homotopy analysis method (HAM) is a semi-analytical method capable of solving both linear and highly nonlinear differential equations. Additionally, HAM is free of variables choices. In order to present the analytical solution of the current framework, HAM is applied.
The linear operators and initial guesses are:
(19)
(19)
(20)
(20)
with
4. HAM convergence
The current model is treated with an analytical approach called HAM which provides an analytical solution for equations of the modelled problem. Here, in this section, the convergence of HAM is presented. HAM has the power to govern and regulates the convergence of the problem. Figures show the convergence areas of velocity, thermal, concentration profiles of the flow for both Casson and Oldroyd-B fluids. The convergence areas for velocity profile is
, thermal profile
is
, and concentration profile
is
for both Casson and Oldroyd-B fluids.
5. Results and discussion
The present portion deals with the graphical outcomes and physical discussion of the mixed convective, thermally radiative, and chemically reactive Casson and Oldroyd-B fluids through a stratified linearly stretching sheet is presented. The analytical explanation of the present model is represented by employing HAM scheme. The flow of Casson and Oldroyd-B fluids is treated using strong magnetic field. In this regards, Figures are displayed. The predefine setting of the embedded factors are chosen as ,
,
,
,
,
,
,
,
and
.
Figure indicates the change in velocity profile via . It is perceived that the greater
reduces the velocity profile for both fluids. Physically, the fluid velocity drops with higher
because of Lorentz force, which yields an opposing force to the fluid flow. Such conflicting intensity generates opposition to the velocity of the fluid particles. Thus, the fluid velocity reduces with the increasing
. Additionally, the reducing impact of magnetic parameter is superior for Oldroyd-B liquid as equated to the Casson liquid. Figure exhibits the variation in
profile via
. Actually, the buoyancy forces heighten with higher
. This increasing buoyancy forces escalates the velocity of the fluids particles that causes the heightening influence in velocity field. Additionally, the increasing impact of mixed convection parameter is dominant for Casson fluid as compared to Oldroyd-B liquid. Figure reveals the variation in
field via buoyancy ratio factor
. The greater
decreases the velocity profile. Physically, the larger
increases the viscosity of the fluids flow which causes to slow down the fluid’s particles movement and consequently the velocity profile reduces. Furthermore, the diminishing influence of buoyancy ratio factor is superior for Oldroyd-B fluid as equated to Casson fluid. Figure displays the change in temperature profile via thermal stratification parameter
. Greater
reduces the thermal profile. Actually, the higher
reduces the thermal alteration between the static flow and sheet surface which results the reducing influence in thermal profile. Figure exhibits the change in thermal profile via thermal radiation parameter
. The greater values of
increases the thermal profile. Greater
upsurges the heat flux forms the sheet which results the heightening effect on thermal profile. That’s why the greater
increases the temperature profile. Figure displays the change in thermal profile via temperature ratio parameter
. Greater
increases the thermal profile. The greater
means supplementary heat will generate to the fluids flow which consequently rises the temperature of the fluids. Figure indicates the variation in concentration profile via concentration stratification parameter
. It is found that the higher
reduces the concentration profile. Figure exhibits the change in concentration profile via Schmidt number
. Greater
reduces the concentration profile. In fact, there is an inverse relation between molecular diffusivity and
. So, the higher
reduces the concentration profile. Figure displays the variation in concentration profile due to chemical reaction parameter
. Larger
reduces the concentration profile. Actually, the greater
creates contrasting force to the fluid flow molecules. The reduced molecular movement of the fluids diminishes the concentration profile. Figures (a–d) and 15(a–d) symbolize the analysis of streamlines for both Casson and Oldroyd-B fluids via magnetic field parameter, respectively. Figures (a) and 15(a) show the non-magnetized fluids flow. Here, we have seen that the streamlines are distinct from each other because of no magnetic field. Figures (b–d) and 15(b–d) represent the streamlines of magnetized Casson and Oldroy-B fluids flow respectively. Here, it is pragmatic that the higher
reduces the fluids flow velocity. As
increase, the streamlines become closer to each other which mean that the velocity profile reduces. This impact is actually as of Lorentz force that causes opposing force to the fluids flow. Furthermore, this impression is leading for Casson liquid as related to Oldroyd-B liquid. The relationship of the present results with the finding already reported is displayed in Table . Here, a great agreement with previously published is found. The influences of the common parameters on
and
numbers have depicted in Tables and for the Casson and Oldroyd-B fluids respectively. The outputs are the same for the Casson and Oldroyd-B fluids. The larger values of
,
,
,
,
and
improving the heat transfer rate and the influence is comparatively better using the Oldroyd-B fluid. While the
number reduces with the larger values of
. Similarly, the Sherwood number upsurges with the larger amount of
,
,
,
and
, and declines with the decreasing value of
and
.
Table 1. Correlation of the current and previous outcomes.
Table 2. Physical parameters versus and
for Casson fluid.
Table 3. Physical parameters versus and
for Oldroyd-B fluid.
6. Final comments
The Mixed convective, thermally radiative, and chemically reactive flow of Casson and Oldroyd-B fluids through a stratified linearly stretching sheet is described in this investigation. The transformed ODEs are treated with an analytical technique called HAM (Homotopy analysis method) which provides an analytical solution for both linear and nonlinear differential equations. The convergence investigation of applied scheme HAM is portrayed using graphs. The variations in the fluids flow profiles via embedded parameters are analysed and debated in depth. The concluding remarks of the current analysis are listed as:
The strong magnetic field and buoyancy ratio parameter moderate the velocity distribution while an opposite trend is found in velocity field via mixed convectional factors. Additionally, the impacts of magnetic and buoyancy ratio parameters are leading for Oldroyd-B liquid as equated to Casson fluid. On the other hand, the mixed convection parameter is dominant for Casson liquid as compared to Oldroyd-B liquid.
The thermal profile reduces with thermal stratification parameter, while increase with nonlinear thermal radiation and temperature ratio parameters.
The concentration profile reduces with concentration stratification parameter, while an opposite behaviour in concentration profile is perceived via Schmidt number and chemical reaction parameter.
Greater reduction in streamlines is exhibited for highly magnetize fluid flows as compared to non-magnetized fluid flows. Additionally, such impact is superior for Casson liquid as associated to Oldroyd-B fluid.
The present results have great agreement with previously published results.
Acknowledgements
The authors gratefully acknowledge the support of the University of Tabuk, Ministry of Education in Saudi Arabia.
Disclosure statement
No potential conflict of interest was reported by the author(s).
Data availability statement
All data used in this manuscript have been presented within the article.
References
- Sakiadis BC. Boundary-layer behavior on continuous solid surfaces: I. Boundary-layer equations for two-dimensional and axisymmetric flow. AIChE J. 1961;7:26–28.
- Sakiadis BC. Boundary-layer behavior on continuous solid surfaces: II. The boundary layer on a continuous flat surface. AIChE J. 1961;7:221–225.
- Tsou FK, Sparrow EM, Goldstein RJ. Flow and heat transfer in the boundary layer on a continuous moving surface. Int J Heat Mass Transf. 1967;10:219–235.
- Crane LJ. Flow past a stretching plate. Z Für Angew Math Phys. 1970;21:645–647.
- Brady JF, Acrivos A. Steady flow in a channel or tube with an accelerating surface velocity. An exact solution to the Navier—Stokes equations with reverse flow. J Fluid Mech. 1981;112:127–150.
- Chiam TC. Stagnation-point flow towards a stretching plate. J Phys Soc Japan. 1994;63:2443–2444.
- Chamkha AJ. Hydromagnetic three-dimensional free convection on a vertical stretching surface with heat generation or absorption. Int J Heat Fluid Flow. 1999;20:84–92.
- Mahapatra TR, Gupta AS. Heat transfer in stagnation-point flow towards a stretching sheet. Heat Mass Transf. 2002;38:517–521.
- Mahapatra TR, Gupta AS. Stagnation-point flow of a viscoelastic fluid towards a stretching surface. Int J Non Linear Mech. 2004;39:811–820.
- Nazar R, Amin N, Filip D, et al. Unsteady boundary layer flow in the region of the stagnation point on a stretching sheet. Int J Eng Sci. 2004;42:1241–1253.
- Ishak A, Nazar R, Pop I. Mixed convection boundary layers in the stagnation-point flow toward a stretching vertical sheet. Meccanica. 2006;41:509–518.
- Ishak A, Nazar R, Pop I. Mixed convection on the stagnation point flow toward a vertical, continuously stretching sheet (2007).
- Patil PM, Roy S, Chamkha AJ. Mixed convection flow over a vertical power-law stretching sheet. Int J Numer Methods Heat Fluid Flow. Vol. 20 No. 4, 2010 pp. 445–458.
- Zaimi K, Ishak A. Stagnation-point flow towards a stretching vertical sheet with slip effects. Mathematics. 2016;4:27.
- Alzahrani EO, Shah Z, Dawar A, et al. Hydromagnetic mixed convective third grade nanomaterial containing gyrotactic microorganisms toward a horizontal stretched surface. Alexandria Eng J. 2019;58:1421–1429. doi:10.1016/J.AEJ.2019.11.013.
- Hayat T, Kiran A, Imtiaz M, et al. Hydromagnetic mixed convection flow of copper and silver water nanofluids due to a curved stretching sheet. Results Phys. 2016;6:904–910. doi:10.1016/J.RINP.2016.10.023.
- Sharada K, Shankar B. Effect of partial slip and convective boundary condition on MHD mixed convection flow of Williamson fluid over an exponentially stretching sheet in the presence of joule heating. Glob J Pure Appl Math. 2017;13:5965–5975. ISSN 0973
- Anantha Kumar K, Sugunamma V, Sandeep N. Physical aspects on unsteady MHD-free convective stagnation point flow of micropolar fluid over a stretching surface. Heat Transf Res. 2019;48:3968–3985.
- Wakif A. A novel numerical procedure for simulating steady MHD convective flows of radiative Casson fluids over a horizontal stretching sheet with irregular geometry under the combined influence of temperature-dependent viscosity and thermal conductivity. Math Probl Eng. 2020;2020:1–20.
- Dawar A, Shah Z, Tassaddiq A, et al. A convective flow of Williamson nanofluid through cone and wedge with non-isothermal and non-isosolutal conditions: a revised Buongiorno model. Case Stud Therm Eng. 2021;24. doi:10.1016/j.csite.2021.100869.
- Soomro FA, Haq RU, Algehyne EA, et al. Thermal performance due to magnetohydrodynamics mixed convection flow in a triangular cavity with circular obstacle. J Energy Storage. 2020;31:101702.
- Kumar MS, Raju CSK, Sherif E-SM, et al. A comprehensive physical insight about enhancement in thermo physical features of newtonian fluid flow by suspending of metallic oxides of single wall carbon nano tube structures. Surf Interfaces. 2021;23:100838.
- Mat NAA, Arifin NM, Nazar R, et al. Radiation effect on Marangoni convection boundary layer flow of a nanofluid. Math Sci. 2012;6:1–6.
- Sha Z, Dawar A, Alzahrani EO, et al. Hall effect on couple stress 3D nanofluid flow over an exponentially stretched surface with cattaneo christov heat flux model. IEEE Access. 2019;7. doi:10.1109/ACCESS.2019.2916162.
- Elbashbeshy EMA, Aldawody DA. Effects of thermal radiation and magnetic field on unsteady mixed convection flow and heat transfer over a porous stretching surface. Int J Nonlinear Sci. 2010;9:448–454.
- Makinde OD. Heat and mass transfer by MHD mixed convection stagnation point flow toward a vertical plate embedded in a highly porous medium with radiation and internal heat generation. Meccanica. 2012;47:1173–1184.
- Abdul-Kahar R, Kandasamy R. Scaling group transformation for boundary-layer flow of a nanofluid past a porous vertical stretching surface in the presence of chemical reaction with heat radiation. Comput Fluids. 2011;52:15–21.
- Ibrahim W, Shankar B. MHD boundary layer flow and heat transfer of a nanofluid past a permeable stretching sheet with velocity, thermal and solutal slip boundary conditions. Comput Fluids. 2013;75:1–10.
- Kumar KA, Sugunamma V, Sandeep N. Effect of thermal radiation on MHD Casson fluid flow over an exponentially stretching curved sheet. J Therm Anal Calorim. 2020;140:2377–2385.
- Yusuf TA, Mabood F. Slip effects and entropy generation on inclined MHD flow of Williamson fluid through a permeable wall with chemical reaction via DTM. Math Model Eng Probl. 2020;7:1–9.
- Mabood F, Yusuf TA, Khan WA. Cu–Al2O3–H2O hybrid nanofluid flow with melting heat transfer, irreversibility analysis and nonlinear thermal radiation. J Therm Anal Calorim. 2021;143:973–984. doi:10.1007/S10973-020-09720-W.
- Yusuf TA, Mabood F, Khan WA, et al. Irreversibility analysis of Cu-TiO2-H2O hybrid-nanofluid impinging on a 3-D stretching sheet in a porous medium with nonlinear radiation: Darcy-Forchhiemer’s model. Alexandria Eng J. 2020;59:5247–5261.
- Ferdows M, Adesanya SO, Alzahrani F, et al. Numerical investigation of a boundary layer water-based nanofluid flow with induced magnetic field. Phys A Stat Mech Appl. 2021;570:125492.
- Mabood F, Berrehal H, Yusuf TA, et al. Carbon nanotubes-water between stretchable rotating disks with convective boundary conditions: Darcy-Forchheimer scheme. Int J Ambient Energy. 2021: 1–14. doi:10.1080/01430750.2021.1874527.
- Krishna MV, Ahammad NA, Chamkha AJ. Radiative MHD flow of Casson hybrid nanofluid over an infinite exponentially accelerated vertical porous surface. Case Stud Therm Eng. 2021;27:101229.
- Yusuf TA, Kumar RN, Prasannakumara BC, et al. Irreversibility analysis in micropolar fluid film along an incline porous substrate with slip effects. Int Commun Heat Mass Transf. 2021;126:105357.
- Yusuf TA, Mabood F, Gbadeyan JA, et al. Nonlinear convective flow for MHD Oldroyd 8-constant fluid in a channel with chemical reaction and convective boundary condition. J Therm Sci Eng Appl. 2020;12:1–13.
- Mabood F, Yusuf TA, Bognár G. Features of entropy optimization on MHD couple stress nanofluid slip flow with melting heat transfer and nonlinear thermal radiation. Sci Rep. 2020;10:1–13.
- Ali B, Thumma T, Habib D, et al. Finite element analysis on transient MHD 3D rotating flow of Maxwell and tangent hyperbolic nanofluid past a bidirectional stretching sheet with Cattaneo Christov heat flux model. Therm Sci Eng Prog. Volume 28, 2022, 101089.
- Yusuf TA, Akaje TW, Salawu SO, et al. Arrhenius activation energy effect on a stagnation point slippery MHD Casson Nanofluid flow with entropy generation and melting heat transfer. In: Defect Diffus. Forum, Trans Tech Publ, 2021. pp. 1–18.
- Krishna MV, Ahamad NA, Chamkha AJ. Radiation absorption on MHD convective flow of nanofluids through vertically travelling absorbent plate. Ain Shams Eng J. 2021;12(3):3043–3056.
- Mabood F, Yusuf TA, Rashad AM, et al. Effects of combined heat and mass transfer on entropy generation due to MHD nanofluid flow over a rotating frame. C Mater Contin. 2021;66:575–587.
- Ameer Ahamad N, Veera Krishna M, Chamkha AJ. Radiation-absorption and Dufour effects on magnetohydrodynamic rotating flow of a nanofluid over a semi-infinite vertical moving plate with a constant heat source. J Nanofluids. 2020;9:177–186.
- Ali B, Naqvi RA, Haider A, et al. Finite element study of MHD impacts on the rotating flow of Casson nanofluid with the double diffusion Cattaneo—Christov heat flux model. Mathematics. 2020;8:1555.
- Ali B, Raju CSK, Ali L, et al. G-Jitter impact on magnetohydrodynamic non-Newtonian fluid over an inclined surface: finite element simulation. Chinese J Phys. 2021;71:479–491.
- Iqbal M, Seadawy AR, Khalil OH, et al. Propagation of long internal waves in density stratified ocean for the (2 + 1)-dimensional nonlinear Nizhnik-Novikov-Vesselov dynamical equation. Results Phys. 2020;16:102838.
- Seadawy AR, Iqbal M, Lu D. The nonlinear diffusion reaction dynamical system with quadratic and cubic nonlinearities with analytical investigations. Int J Mod Phys B. 2020;34:2050085.
- Iqbal M, Seadawy AR, Lu D, et al. Construction of bright–dark solitons and ion-acoustic solitary wave solutions of dynamical system of nonlinear wave propagation. Mod Phys Lett A. 2019;34:1950309.
- Seadawy AR, Iqbal M, Lu D. Propagation of long-wave with dissipation and dispersion in nonlinear media via generalized Kadomtsive–Petviashvili modified equal width-Burgers equation. Indian J Phys. 2020;94:675–687.
- Seadawy AR, Iqbal M, Lu D. Applications of propagation of long-wave with dissipation and dispersion in nonlinear media via solitary wave solutions of generalized Kadomtsev–Petviashvili modified equal width dynamical equation. Comput Math Appl. 2019;78:3620–3632.
- Lu D, Seadawy AR, Iqbal M. Mathematical methods via construction of traveling and solitary wave solutions of three coupled system of nonlinear partial differential equations and their applications. Results Phys. 2018;11:1161–1171.
- Algehyne EA, Saeed T, Ibrahim M, et al. Investigation of dissimilar laser welding of stainless steel 304 and copper using the artificial neural network model. J Laser Appl. 2021;33:022010.
- Mahmoud EE, Alqarni MM, Algehyne EA, et al. Nanoparticles shape effect on the efficiency of microheat sinks with tightly packed pin-fins. Chem Eng Commun. 2021:1–11. doi:10.1080/00986445.2021.1948408.
- Elanchezhian E, Nirmalkumar R, Balamurugan M, et al. Heat and mass transmission of an Oldroyd-B nanofluid flow through a stratified medium with swimming of motile gyrotactic microorganisms and nanoparticles. J Therm Anal Calorim. 2020;141:2613–2623.
- Dawar A, Shah Z, Alshehri HM, et al. Magnetized and non-magnetized Casson fluid flow with gyrotactic microorganisms over a stratified stretching cylinder. Sci Rep. 2021;11:1–14.
- Shehzad SA, Alsaedi A, Hayat T, et al. Three-dimensional flow of an Oldroyd-B fluid with variable thermal conductivity and heat generation/absorption. PLoS One. 2013;8:e78240.
- Dawar A, Saeed A, Islam S, et al. Electromagnetohydrodynamic bioconvective flow of binary fluid containing nanoparticles and gyrotactic microorganisms through a stratified stretching sheet. Sci Rep. 2021;11:1–29.
- Wakif A, Animasaun IL, Khan U, et al. Dynamics of radiative-reactive Walters-b fluid due to mixed convection conveying gyrotactic microorganisms, tiny particles experience haphazard motion, thermo-migration, and Lorentz force. Phys Scr. 2021. doi:10.1088/1402-4896/AC2B4B.
- Ramesh GK, Kumar KG, Shehzad SA, et al. Enhancement of radiation on hydromagnetic Casson fluid flow towards a stretched cylinder with suspension of liquid-particles. Can J Phys. 2018;96:18–24.