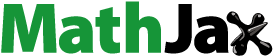
Abstract
Waves characteristic of solitary damped forms in plasmas fluids contain two polarity ions, nonextensivity positrons and electrons have been studied. The damped equation of Kadomtsev–Petviashvili (DKP) equation has been derived. The critical plasma density condition of DKP equation is introduced for parameters concerning Earth's ionosphere. The impacts of densities, ionic mass ratio, index of nonextensivity and frequencies of collision parameters on the nonlinear structures have been investigated. It is mentioned that the consequences obtained from this study may be applied in space plasmas.
1. Introduction
The physical phenomena description using equations with damped nonlinear structural solutions and their applications are one of the important intractable issues in the dynamics of fluid and plasmas [Citation1–5]. Dynamical and collisional features of DIAWs have been studied in nonextensive electron dusty plasma via novel approximated analytical structures for the DKdV equation [Citation6]. In space models and experimental labs, the damped noises observed may cause considerable puzzles in localized soliton behaviours [Citation7–12]. The distorted and dissipated structures in soliton shapes were studied in various studies via numerous physics ways, such as particles collision [Citation13–17], fluctuation in charges [Citation4,Citation18], fluids kinematics viscosity [Citation19,Citation20]. The assistances of strength and frequencies on the damped KdV equation with a forced term that describe dust superthermality plasma have been introduced [Citation5]. They found that the force strength and frequency were significantly modulated the wave structures of the damped soliton. Also, the realizations in models containing pairs of ions (PI) becomes one of the motivating investigations for the propagation of ion acoustic-soliton (IAS) application in many astrophysics models and laboratories [Citation21–28]. Abdelwahed et al. [Citation29] inspected the effects of electron superthermal parameters and mass ratios on the waves of rogue profiles in ion pair system. However, plasma of electrons-positrons-ions (E-P-I) is observed in astrophysics, quantum hydrodynamics environments and industry of semiconductors [Citation30–39]. Furthermore, the double-layer existence in the model with two temperature ions and superthermality electrons has been inspected [Citation40]. The criticality state is based on the electrons index and thermal ionic ratio. Also, large soliton amplitudes in electronegative fluid have been studied by pseudo-potential technique [Citation41]. It was noted that the positive–negative potentials were formed by nonextensively electrons. The effects of frequencies of collisions on damped 3D cylindrical solitary forms in plasma of the dust-negative-positive model have been studied [Citation42]. They recited that the collision frequencies and radial effects substantially influenced the damped structures. On the other hand, plasmas usually contain particles having distributions in non-Maxwellian forms such as nonthermal, trapped, and nonextensive electron-ion distributions [Citation25,Citation43–47]. Moreover, nonextensive distribution has many applications in astrophysics, auroral plasma and galaxy clusters [Citation46–50]. The ionization and nonextensive effects on dynamical transition and solitary excitations have been investigated in a dusty collisional plasma model [Citation51]. El-Taibany investigated both the 3D IAWs Modulation instability and highly energetic freak IAW waves in Earth's Ionosphere model having non-Maxwellian (r,q) distributions of electrons (positrons) via 3D NLSE. It was discussed the stabe-unstabe conditions depend mainly on obliqueness angle and the (r,q) distributions parameters [Citation52]. So, we aim to investigate the damping structure's dependence on physical parameters such as the ionic mass ratio , the frequencies of collision parameters (
and the index of nonextensive (q).
2. Equations of model
In this system, a collisional four-components unmagnetized plasma contains negative and positive fluids, nonextensive electrons and positrons are suggested. Normalized model is given by:
(1a)
(1a)
(1b)
(1b)
(1c)
(1c)
(2a)
(2a)
(2b)
(2b)
(2c)
(2c)
which is supplemented by Poisson's equation:
(3)
(3)
Where
denotes the density of negative and positive ions normalized by
are the fluid velocities of ions (normalized by
), potential φ (normalized by
),
(normalized by
and
, respectively).
is the frequency for loss in positive ion momentum due to the recombination on negative one and the collisions between them,
are the ionization frequency of plasma. These collision frequencies are normalized by
.
is the mass ratio of positive–negative ions,
where
are charges number. Here,
and
are electrons temperature and Boltzmann constant. The electron and positron density (
and
) expressions through the nonextensive velocity distribution function can be written as:
(4)
(4)
where
and
is the positron (electron) temperature. The q-nonextensive distributed electrons and positrons are used on similar notations in [Citation53,Citation54] wherein the algebraic calculations are given in details.
Where q>−1. Thus, condition equilibrium reads
(5)
(5)
with
and
.
3. Damped KP equation
We used the new stretched coordinates as:
(6)
(6)
where
is a small expansion value (velocity of IAWs), (we assumed that
,
and
. All dependent system variables are expanded in ϵ as:
(7)
(7)
The boundary conditions are
By the use of Equations (Equation6
(6)
(6) ) and (Equation7
(7)
(7) ) in Equations (Equation1a
(1a)
(1a) )–(Equation3
(3)
(3) ), equating same coefficients of ϵ order. Lowest orders gives:
(8)
(8)
(9)
(9)
With condition:
(10)
(10)
The higher orders in ϵ reads:
(11)
(11)
(12)
(12)
and
(13)
(13)
where
.
After several algebraic steps with the aids of Equations (Equation8(8)
(8) )–(Equation10
(10)
(10) ), and (Equation11
(11)
(11) )–(Equation13
(13)
(13) ) gives the DKP equation:
(14)
(14)
Where
Analytically, Equation (Equation14
(14)
(14) ) is not solved exactly. So, in a weakly dissipation using the transformation:
(15)
(15)
where L and M are X and Y cosine directions, so, the approximated solution of Equation (Equation14
(14)
(14) ) can be obtained as [Citation42,Citation55]:
(16)
(16)
With
(17)
(17)
(18)
(18)
(23)
(23)
where the amplitude and width
and
and
is the amplitude for C = 0.
4. Results and discussion
The IAW properties in our model are explained. The model equations are reduced to nonlinear DKP equations with the damped term. Using parameters corresponding to D-F-Earth's ionosphere, the damped wave properties have been examined [Citation25,Citation28,Citation56]. Since, our intent is to check some effects of the frequencies of collision parameters ( the ionic mass ratio
, and the index of nonextensive (q) on the IAW formation. The studied system supports the coexistence of compressive and rarefactive damped soliton kind as in Figure .
As clear in Figure , at value of Q i.e.
a positive potential is existed, whereas at
i.e.
a negative potential is existed. To show the potency of wave formation on Q parameter, the potential time evolutions are given in Figure . It was shown that the compressive potential damped with time, but rarefactive potential growing with time. The sensitivity of potential properties due to the parameters q and the model frequency parameters
,
and
on the potential amplitude and width are investigated in Figures –. The amplitudes of both compressive and rarefactive potential decrease by q also their width decreases as depicted in Figures and . On the other hand, it is apparent from Figures that the potential amplitudes magnitude of compressive and rarefactive generations decreases by
and increases with
and
. Accordingly, the two types of width raise with
and decrease by
and
.
5. Conclusions
Nonextensive positron plasma mode has been investigated by the damped nonlinear DKP equation. Both damped compressive and rarefactive solitons are obtained. It was reported that the properties of existing solitons are damping or growing with time in the ionosphere plasma model and affecting by the parameter of nonextensivity and each positive and negative ion masses.
Acknowledgment
This project was supported financially by the Academy of Scientific Research and Technology (ASRT), Egypt [grant number 6493] under the project Science Up. (ASRT) is the 2nd affiliation of this research.
Disclosure statement
No potential conflict of interest was reported by the authors.
References
- Popel SI, Golub' AP, Losseva TV. Weakly dissipative dust-ionacoustic solitons. Phys Rev E. 2003;67(5):Aritcle ID 056402.
- Ghosh S. Effect of ionization on ion acoustic solitary waves in a collisional dusty plasma. J Plasma Phys. 2005;71(4):519–526.
- Sultana S, Kourakis I. Electron-scale dissipative electrostatic solitons in multi-species plasmas. Phys Plasmas. 2015;22(10):Article ID 102302.
- Wang Y, Guo X, Lu Y, et al. The nonadiabatic dust charge variation on dust acoustic solitary and shock waves in strongly coupled dusty plasmas. Phy Let A. 2016;380(1–2):215–221.
- Chatterjee P, Ali R, Saha A. Analytical solitary wave solution of the dust ion acoustic waves for the damped forced Korteweg–de Vries equation in superthermal plasmas. Zeitschrift fur Naturforschung a. 2018;73:2.
- Sharma P, Das A, Tamang J, et al. Dynamical properties of dust-ion-acoustic wave solutions in a nonextensive collisional dusty plasma. J Taibah Univ Sci. 2021;15(1):710–720.
- Nakamura Y, Odagiri T, Tsukabayashi I. Ion-acoustic waves in a multicomponent plasma with negative ions. Plasma Phys Control Fusion. 1997;39(1):105–115.
- Horányi M. Charged dust dynamics in the solar system. Ann Rev Astron Astrophys. 1996;34(1):383–418.
- Popel SI, Tsytovich VN, Yu MY. Shock structures in plasmas containing variable-charge macro particles. Astrophys Space Sci. 1997;256(1/2):107–123.
- Moolla S, Bharuthram R, Baboolal S. Reduction of dust acoustic soliton amplitude due to dust charge variation. Phys Plasmas. 2005;12(4):Article ID 042310.
- Gill TS, Saini NS, Kaur H. The Kadomstev-Petviashvili equation in dusty plasma with variable dust charge and two temperature ions. Chaos Solitons Fract. 2006;28(4):1106–1111.
- Tang RA, Xue JK. Stability of oblique modulation of dust-acoustic waves in a warm dusty plasma with dust charge variation. Phys Plasmas. 2003;10(9):3800–3803.
- Moslem WM. Linear and nonlinear properties of dust-acoustic waves in collisional, magnetized dusty plasmas. Phys Plasmas. 2003;10(8):3168–3173.
- Vranjes J, Petrovic D, Pandey BP, et al. Electrostatic modes in multi-ion and pair-ion collisional plasmas. Phys Plasmas. 2008;15:Article ID 072104.
- Losseva TV, Popel SI, Golub' AP, et al. Weakly dissipative dust-ion-acoustic solitons in complex plasmas and the effect of electromagnetic radiation. Phys Plasmas. 2012;19(1):Article ID 013703.
- El-Labany SK, El-Shewy EK, Abd El-Razek HN, et al. Cylindrical three-dimensional dust-ion acoustic propagation in plasmas. Commun Theor Phys. 2018;70(3):325.
- El-Taibany WF, EL-Labany SK, El-Helbawy AS. Dust-acoustic solitary and periodic waves in magnetized self-gravito-electrostatic opposite polarity dusty plasmas. Eur Phys J Plus. 2022;137(2):261.
- El-Labany SK, Moslem WM, Mowafy AE. Effects of trapped electron temperature, dust charge variations, and grain radius on the existence of the dust-ion-acoustic waves. Phys Plasmas. 2003;10(11):4217–4223.
- El-Hanbaly AM, Sallah M, El-Shewy EK, et al. Kinematic dust viscosity effect on linear and nonlinear dust-acoustic waves in space dusty plasmas with nonthermal ions. J Exp Theor Phys. 2015;121(4):669–679.
- Zaghbeer SK, Salah HH, Sheta NH, et al. Effect of nonextensive electron and ion on dust acoustic rogue waves in dusty plasma of opposite polarity. Astrophys Space Sci. 2014;353(2):493–500.
- Jacquinot J, McVey BD, Scharer JE. Mode conversion of the fast magnetosonic wave in a deuterium-hydrogen tokamak plasma. Phys Rev Lett. 1977;39(2):88–91.
- Lakhina GS, Verheest F. Alfveénic solitons in ultrarelativistic electron-positron plasmas. Astrophys Space Sci. 1997;253(1):97–106.
- Oohara W, Hatakeyama R. Electrostatic waves in a paired fullerene-ion plasma. Phys Rev Lett. 2005;95(17):Article ID 175003.
- Oohara W, Hatakeyama R. Basic studies of the generation and collective motion of pair-ion plasmas. Phys Plasmas. 2007;14(5):Article ID 055704.
- Elwakil SA, El-Shewy EK, Abdelwahed HG. Envelope ionacoustic solitary waves in a plasma with positive-negative ions and nonthermal electrons. Phys Plasmas. 2010;17(5):Article ID 052301.
- El-Labany SK, Moslem WM, El-Bedwehy NA, et al. Rogue wave in Titan's atmosphere. Astrophys Space Sci. 2012;338(1):3–8.
- Saleem H. A criterion for pure pair-ion plasmas and the role of quasineutrality in nonlinear dynamics. Phys Plasmas. 2007;14:Article ID 014505.
- Sabry R, Moslem WM, Shukla PK. Fully nonlinear ionacoustic solitary waves in a plasma with positive-negative ions and nonthermal electrons. Phys Plasmas. 2009;16: Article ID 032302.
- Abdelwahed HG, El-shewy EK, Zahran MA, et al. On the rogue wave propagation in ion pair superthermal plasma. Phys Plasmas. 2016;23:Article ID 022102.
- Tandberg-Hansen E, Emslie AG. The physics of solar flares. Cambridge: Cambridge University Press; 1988.
- Popel SI, Vladimirov SV, Shukla PK. Ion-acoustic solitons in electron-positron-ion plasmas. Phys Plasmas. 1995;2(3):716–719.
- Roy K, Misra AP, Chatterjee P. Ion-acoustic shocks in quantum electron-positron-ion plasmas. Phys Plasmas. 2008;15(3):Article ID 032310.
- Markowich PA, Ringhofer CA, Schmeiser C. Kinetic transport models for semiconductors 83. Vienna: Semiconductor Equations Springer; 1990.
- Jung YD. Quantum-mechanical effects on electron– electron scattering in dense high-temperature plasmas. Phys Plasmas. 2001;8(8):3842–3844.
- Haas F, Manfredi G, Goedert J. Nyquist method for Wigner-Poisson quantum plasmas. Phys Rev E. 2001;64(2):Article ID 026413.
- Shokri B, Rukhadze AA. Quantum surface wave on a thin plasma layer. Phys Plasmas. 1999;6(9):3450–3454.
- Misra AP, Roy Chowdhury A. Modulation of dust acoustic waves with a quantum correction. Phys Plasmas. 2006;13:Article ID 072305.
- Chowdhury NA, Mannan A, Hasan MM, et al. Heavy ion-acoustic rogue waves in electron-positron multi-ion plasmas. Chaos Interdisci J Nonlinear Sci. 2017;27(9):Article ID 093105.
- Ferdousi M, Sultana S, Mamun AA. Oblique propagation of ionacoustic solitary waves in a magnetized electron-positron-ion plasma. 2015 Phys Plasmas. 2017;22(3):Article ID 032117.
- Shaha MG, Rahmanb MM, Hossenc MR, et al. Heavy ion-acoustic solitary waves and double layers in a multi-ion plasma. Plasma Phys Rep. 2018;44(9):861–869.
- Hatami MM, Tribeche M, Mamun AA. Effects of positive ion temperature and negative ion number density on arbitrary amplitude ionacoustic solitary waves in nonextensive electronegative plasma. Astrophys Space Sci. 2019;364(1):21.
- El-Shewy EK, El-Rahman AA. Cylindrical dissipative soliton propagation in nonthermal mesospheric plasmas. Phys Scr. 2018;93(11):Article ID 115202.
- Saha A, Prasad PK, Banerjee S. Bifurcation of ion-acoustic superperiodic waves in auroral zone of Earth'smagnetosphere. Astrophys Space Sci. 2019;364(10):180.
- Kumar R, Malik HK. Modified Korteweg-de Vries soliton reflection in a magnetized plasma with dust grains and trapped electrons. Phys Plasmas. 2013;20(3):Article ID 032112.
- Malik HK, Kumar R, Lonngren KE, et al. Collision of ion acoustic solitary waves in a magnetized plasma: effect of dust grains and trapped electrons. Phys Rev E. 2015;92(6):Article ID 063107.
- Tribeche M, Djebarni L, Amour R. Ion-acoustic solitary waves in a plasma with a q-nonextensive electron velocity distribution. Phys Plasmas. 2010;17(4):Article ID 042114.
- Saha A, Chatterjee P. Solitonic, periodic, quasiperiodic and chaotic structures of dust ion acoustic waves in nonextensive dusty plasmas. Eur Phys J D. 2015;69(9):395.
- Bacha M, Gougam LA, Tribeche M. Ion-acoustic rogue waves in magnetized solar wind plasma with nonextensive electrons. Physica A Stat Mech Appl. 2017;466:199–210.
- Bains AS, Tribeche M, Saini NS, et al. Modulation instability and rogue wave structures of positron-acoustic waves in q-nonextensive plasmas. Physica A Stat Mech Appl. 2017;466:111–119. d.
- Saha A, Chatterjee P. Electron acoustic blow up solitary waves and periodic waves in an unmagnetized plasma with kappa distributed hot electrons. Astrophys Space Sci. 2014;353(1):163–168.
- Tamang J, Sarkar K, Saha A. Solitary wave solution and dynamic transition of dust ion acoustic waves in a collisional nonextensive dusty plasma with ionization effect. Phys A Stat Mech Appl. 2018;505:18–34.
- El-Taibany WF, El-Bedwehy NA, El-Shafeay NA, et al. Three-dimensional rogue waves in Earth's ionosphere. Galaxies. 2021;9(3):48.
- Silva R, Plastino AR, Lima JAS. A Maxwellian path to the q-nonextensive velocity distribution function. Phys Lett A. 1998;249(5–6):401–408.
- El-Awady EI, Moslem WM. On a plasma having nonextensive electrons and positrons: rogue and solitary wave propagation. Phys Plasmas. 2011;18(8):Article ID 082306.
- Karpman VI, Maslov EM. Perturbation theory for solitons. Sov Phys JETP. 1977;46:281.
- Nakamura Y, Tsukabayashi I. Observation of modified Korteweg-de Vries solitons in a multicomponent plasma with negative ions. Phys Rev Lett. 1984;52(26):2356–2359.