Abstract
Melt quenching technique was used to prepare (30-x)Li2O–xNa2O–40B2O3–30GeO2 glasses (x = 0, 5, 10, 20 & 30 mol %). The room temperature infrared spectra of the powdered glass samples were recorded in the range of 400–2000cm−1. Raman measurements were performed in the range of 200–2000cm−1 at room temperature. Raman and Infrared spectroscopy was utilized for structural study of this glass system. Specific modes of vibration and peak position were identified from de-convolution of infrared and Raman spectra using Gaussian fitting. Ge–O–Ge bonds’ symmetrical stretching vibrations related to 3 members GeO4 rings were allotted to the IR band of 483 cm−1. The strongest Raman band was allotted to symmetrical stretching vibrations of Ge–O in GeO4 having one non-bridging oxygen with 3 bridging oxygens at 797 cm−1. The observed Raman and IR spectra of various specimens are utilized here to explain the structural characteristics of current glass.
1. Introduction
Germanium dioxide has been used for many years as a candidate for the formation of glass. Glasses made of GeO2 have excellent thermal stability, high phonon energy, and excellent infrared transmission. Germanium-based glasses are promising candidates for use in waveguides, laser amplifiers, sensors, light and other optical devices due to the relatively low energy losses in the mid-infrared region [Citation1,Citation2]. These glasses are extensively used in modern optical fibres because of their chemical resilience, mechanical strength, and high refractive index. Multi-components glass shows nonlinear changes in characteristics when one of the alkali component is replaced by some other species [Citation3,Citation4]. Nonlinear characteristics are also seen in mixed alkali-alkaline earth oxide glasses [Citation5–7]. This is called the mixed-alkali effect (MAE), and this phenomenon may be used to make low-loss electrical glass while also increasing the chemical robustness of the material. Many factors influence the MAE's strength, including temperature, concentration of total alkali, radius and mass difference of mixed alkali ions, and so on [Citation8–10]. MAE can be observed in phosphates, silicates, tellurite, tungstate, and borate glasses, among other glass systems [Citation11–13]. Moulton et al. discussed MAE of Both alkali and alkaline-earth silicate glasses and their numerous industrial applications in window glass, fibre optics and biomaterials [Citation14]. Glasses with a mixed alkali effect can be employed to develop materials with specific qualities and modify their features to meet specific industrial requirements. Addition of alkali oxide in GeO2 glass increases density, but only to a maximum before declining. This is called Germanate anomaly in the glass. This pattern may be seen in a variety of physical characteristics like refractive index, density, hardness, elastic moduli etc in the presence of alkali oxides [Citation10]. When the alkali oxide content of the GeO2 network rises, the transformation of GeO4 tetrahedra to GeO6 octahedra is predicted, resulting in a Germanate anomaly. When alkali oxide is added to GeO2, the density of the resultant glass increases with the amount of alkali oxide applied, rising at 10–20 mol% alkali oxide and subsequently declining. The type of the alkali oxide applied determines the anomaly maximum. Henderson [Citation15,Citation16] has addressed the nature of this behaviour as well as the possible structural mechanisms behind it. According to Raman investigations [Citation17], there is no alteration in the germanium/boron coordination in GeO2–B2O3 glasses.
The structural development of the glass network was investigated using Raman spectra and FT-IR absorption spectra because NIR emission sort of behaviour is shown to be responsive to glass network local structure [Citation18,Citation19]. Borate glasses have been shown to be good hosts for studying mixed alkali phenomena. Glasses containing B2O3 have been the subject of a number of investigations on combined alkali effects. To explore the influence of changes in the alkali fractions on the EPR parameters, EPR investigations were executed in the xK2O:(30–x)Li2O–10CdO–59B2O3–1MnO2 amorphous system [Citation20]. S Rahman et al. investigated the mass density, ionic conductivity, and glass transition temperature of (40-x)Na2O:xLi2O–50B2O3–10Bi2O3 and (40-x)Na2O:xK2O–50B2O3–10Bi2O3 borate glasses with Na2O concentrations ranging from 0 to 40% [Citation21]. Edukondalu et al. used the melt quench method to create xLi2O–(30-x)Na2O–10WO3–60B2O3 alkali borotungstate glasses and then investigated FT-IR and Raman spectroscopy [Citation22]. Although there is research on alkali germanate glasses, and there are few publications on the mixed alkali effect in borate glasses, including GeO2. Brian M. Walsh et al. published a spectroscopic investigation of Tm-doped germanate glass for luminescence decay, absorption, and emission [Citation23]. Density of states, DC conductivity and physical properties of Ag2S-Ge–Te–Se chalcogenide glassy system has been discussed by Chamuah et al. in the light of DC conductivity model and variable range hopping model [Citation24]. Franco et al. have investigated thermal, structural properties of Germanium–Aluminum–Borate glass (85-x)GeO2–(x)B2O3–10Na2O–4Al2O3–1PbO using DSC, Raman scattering and NMR spectroscopies [Citation25]. Liu et al. studied ultra broadband near-infrared emission in RO–Al2O3–B2O3–GeO2 with 0.005 mol% Bi2O3 doping under 460 nm excitation, principally consisting of 3 bands [Citation26]. As per our literature review, the structural characteristics of (30-x)Li2O–xNa2O–40B2O3–30GeO2 alkali borogermanate glasses are inadequately documented in the literature. Borate glasses with a wide range of composition and characteristics have an excellent scope in nonlinear as well as in linear optics. The goal of this article is to investigate the structure of (30-x)Li2O–xNa2O–40B2O3–30GeO2 (x = 0, 5, 10, 20, and 30) on glasses using two important spectroscopic methods: Raman and IR. This research is essential since the recent results will give knowledge about the fundamental units that make up this multi-component glass structure.
2. Experiment
Melt quenching was utilized to make a sequence of mixed alkali glasses (30-x)Li2O–xNa2O–40B2O3–30GeO2 (x = 0, 5, 10, 20, and 30 mol%). As starting ingredients, highly pure Na2CO3, Li2CO3, B2O3, and GeO2 (99.99% pure, Sigma-Aldrich) were used. A stoichiometric ratio of 20 g of these powders blended well in an agate mortar before being liquefied in a ceramic vessel placed in an electric oven at a high temperature range of 1100–1200 °C for about 30 min. The melt was periodically spun until a clear, bubble-free liquid was created. The resulting melt was warmed to 200 °C and pressed with a steel disc that had been preheated to the same temperature. Different glass compositions were annealed for around 12 h at 200 °C. Lack of any Bragg peaks in the x-ray diffraction patterns revealed that the glass samples were amorphous. The current materials were crushed and mixed with KBr to make pellets that were about 0.3 mm thick for FTIR examination. The IR absorption spectra at room temperature were obtained utilizing a Bruker Optics Spectrometer. The IR observations were taken between 400 and 2000 cm−1. A Renishaw (UK) spectrometer was utilized for investigation of micro Raman spectra at 514 nm excitation wavelength. An external CCD sensor was used in combination with an NIR-adjusted Olympus LMPl microscope objective, and 1800 lines/mm grating.
3. Results and discussion
The most suited approach for glass studies is infrared transmittance spectroscopy. Figure depicts the IR spectra of (30-x)Li2O–xNa2O–40B2O3–30GeO2 measured in the region of 400–2000cm−1. The apparent IR spectra of the present glass framework arises mainly from altered borate networks in active spectral range from 900 to 1600 cm−1 and from the altered germanate groups in the active spectral range of 450-770 cm−1. The IR spectra with overlapping broad spikes are de-convoluted using 6 peaks and Gaussian functions [Citation27–29]. The de-convoluted IR of (30-x)Li2O–xNa2O–40B2O3–30GeO2 glass network for various x are shown in Figure . The deconvoluted specifications of the FTIR spectra of (30-x)Li2O–xNa2O–40B2O3–30GeO2 are presented in Table .
Table 1. Deconvoluted parameters of the FT-IR spectra of the xNa2O–(30−x)Li2O–40B2O3–30GeO2 glasses under study. C is the component band center (cm−1), A is the component band's relative area (percentage) and W is full width at half maxima.
The boron ion is a cation for the formation of glass network that may be found at the center of oxygen triangles or in tetrahedral structures [Citation30]. The structure of boron oxide glass, as per Krogh Moe's model, is a randomized network of planar BO3 triangles with a specified fraction of 6 member rings [Citation31]. In general, between 600 and 1200 cm−1, the infrared spectra of vitreous B2O3 exhibits no active modes, while IR examination of modified borate glasses indicates discrete frequency areas in the mid-infrared range, where the vibrations of the B–O configuration dominate. IR absorption bands of borogermanate glasses are ascribed to bending vibrations of different borate units and stretching vibrations of germanate units. B–O–B stretching vibrations of polymerized BO3 are attributed to the infrared band approximately 1380 cm−1 of present glass, in which the boron atom is associated with 3 oxygen atoms in the BO3 unit [Citation32]. The B–O asymmetric stretching of tetrahedral BO4 units is allocated to the next band at 1098 cm−1 [Citation33]. In the Li2O–B2O3–GeO2 glass system, H. Doweider et al. detected IR bands at 1080 and 1390 cm−1, which were attributed to boron–oxygen bond vibrations and B–O–B stretching state of chemically bonded BO3 groups respectively [Citation33]. The symmetrical stretching oscillations of Ge–O–Ge bonds related to the three members of GeO4 rings were assigned to the IR band at 483 cm−1 in the current glasses. The intensity of 483 cm−1 decreases as Na2O concentration rises, and the IR band's core shifts to longer wavelengths. Sahar et al. discovered an IR band of range 448–493 cm−1 in Na2O–P2O5–GeO2 glasses, which they assigned to symmetric stretching states of Ge–O–Ge bonds [Citation34]. A moderate shoulder in the current glass system's infrared spectra was detected at 779 cm−1 and it was allotted to the stretching vibration of Ge–O in GeO4 [Citation35]. In Alumina-bearing lanthanide borogermanate glasses, Sigaev et al. detected an IR band at 800 cm−1, which they linked to stretching Ge–O vibration in GeO4 units [Citation35]. Stretching vibrations of B–O bonds in BO4 units from diborate groups are assigned to the 948 cm−1 IR band, while stretching vibrations of B–O bonds in BO4 units from pentaborate groups is attributed to the 1098 cm−1 band in the current glasses [Citation36]. In the present glass system, a weak IR band at 1555 cm−1 is coupled to asymmetric stretching vibrations with 3 non-bridging oxygen (NBO) of B–O–B groups [Citation37]. The IR band of about 1570 cm−1 in AgI–Ag2O–GeO2–B2O3 glasses is ascribed to the B–O–B stretching vibrational network for different “xˮ modes of polymerized BO3 units [Citation37]. Table assigns the Fourier transform of IR spectra of the (30-x)Li2O–xNa2O–40B2O3–30GeO2 glass scheme.
Table 2. Fourier Transform Infrared allocations in (30-x)Li2O–xNa2O–40B2O3–30GeO2 glass network.
The compositional dependency of IR band peak in the current investigation is depicted in Figure . The MAE is demonstrated by a nonlinear change in peak location for the IR bands located at approximately 1098 and 1555 cm−1. It is evident from these graphs that the IR peak positions with composition deviate from linearity. The IR reflectivity of mixed-alkali borate glasses was investigated by Kamitsos et al. who discovered that alkali mixing induced partial annihilation of BO4 groups in favour of their BO2O– isomeric triangles. They also discovered that fraction of NBO exhibited a positive deflection from linearity [Citation38]. By using IR spectroscopy along with nuclear magnetic resonance, Kamitsos et al. discovered that the alkali ion has a well-defined effect on the network structure: the proportion of tetrahedrally connected boron atoms reduces as the alkali ion size increases, or identically the ion's field strength increases [Citation39]. The Raman spectra of varios specimens were examined in order to better understand how the glass characteristics changed when Na2O was added. Figure shows Raman spectra of (30-x)Li2O–xNa2O–40B2O3–30GeO2 with various x. Using Gaussian functions, a Raman spectra with overlapped wide peak is de-convoluted into 5 peaks. Figure depicts the de-convolution of Raman spectra using a Gaussian fit of (30-x)Li2O–xNa2O–40B2O3–30GeO2 glass network for various x. Table shows the de-convoluted characteristics (band centre and relative percentage area of band) of the Raman spectra of the (30-x)Li2O–xNa2O–40B2O3–30GeO2 glass system.
Table 3. Deconvoluted parameters of the Raman spectra of the xNa2O–(30−x)Li2O–40B2O3–30GeO2 glasses under present investigation.
Different Raman bands of glass samples are approximated as 538, 797, 1040, 1396, and 1514 cm−1. The Raman band centered at 538 cm−1 should have originated from the bending vibration of Ge–O–Ge [Citation40,Citation41]. G.S. Henderson has also mentioned that the Ge–O–Ge bending modes are observed in the broad region between 500 and 620 cm−1 [Citation42]. The strongest Raman band was assigned with asymmetrical stretching vibrations of Ge–O in GeO4 having one non-bridging oxygen and three bridging oxygen at 797 cm−1 (Q3 species) of current glass [Citation43]. According to a literature study, the asymmetrical stretching vibration of four-fold coordinated Ge (i.e. stretching of Ge–O–Ge and O–Ge–O–, where O– is the non-bridging) is associated to the Raman signal between 700 and 900 cm−1 [Citation43]. As a consequence, the Ge–O stretching vibration was attributed to the Raman peak about 797 cm−1. For glasses, we detected a wide peak at 797 cm−1 and 1292 cm−1, indicating that there are several vibrational modes linked together. The peak's breadth might be related to structural heterogeneity, which varies depending on ring diameters and the number of NBOs. The detected Raman band with an interval of 1045–1096 cm−1 in the current glass network was ascribed to the stretching vibration associated with B–O bonds in BO4 unit [Citation44–46]. Raman band 1285–1396 cm−1 was assigned to the vibrating mode of B–O terminal bonds of BO3 triangles in this study [Citation45,Citation46]. In the current glass network, the B–O bond stretching vibration of polymerized BO3 unit was assigned to the Raman band at 1437–1514 cm−1 [Citation45]. Table shows the Raman band allocations of (30-x)Li2O–xNa2O–40B2O3–30GeO2 glass network. Subhashini et al. also detected band about 1350 cm−1 with shoulder around 1120–1250 cm−1 in Na2O–Li2O–K2O–ZnO–B2O3 glass system, which were attributed to the BO2O− triangles connected with BO units [Citation47].
Table 4. Wave numbers and allocations of Raman spectra of (30-x)Li2O–xNa2O–40B2O3–30GeO2 present glasses.
Figure illustrates a normalized change of ratio of intensity between the Raman bands situated at 797 and 538 cm−1, indicated as I797/I538, as well as a shifting of the peak of band 538 cm−1 with Na2O content. In the Raman spectra of glasses (Figure ), I797/I538 initially increases with x up to x = 0.20, then begins to drop. Table shows change of relative intensity I797/I538 with Na2O content. Raman spectroscopy investigations of alkali borate glasses for various Na2O contents suggest the possibility of two chemical processes distributed throughout the glasses. The first step, which occurs at lower Na2O content, produces boron in four fold coordination with Na+ ion providing local charge neutrality. The 2nd phase is the creation of NBO (O–) close to the Na+ ion. The two chemical processes mentioned above can also be used to explain N4 dependency.
Figure 6. Normalized change of the intensity ratio (I797/I538) between the Raman bands situated at 797 and 538 cm−1, as well as a shifting of the peak of band 538 cm−1 with Na2O content.
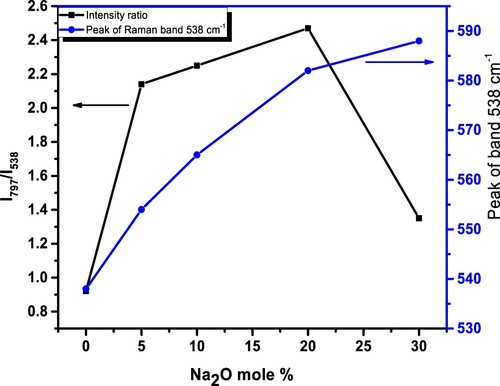
Table 5. Change of relative intensity I797/I538 with Na2O content.
4. Conclusions
The FTIR and Raman spectra of (30-x)Li2O–xNa2O–40B2O3–30GeO2 glass systems were analyzed to identify local structural variations. In the glass network, we allocated vibrational modes to various sorts of atomic motions. The examined glasses’ de-convoluted Raman and IR spectra demonstrated the existence of five Raman bands and six IR bands, respectively. IR absorption bands of the borogermanate glasses were allotted to bending oscillation of different borate sections and stretching oscillations of germanate unit. Symmetrical stretching vibrations of Ge–O–Ge bonds related to the IR band at 483 cm−1. The intensity of this band diminished and IR band moved to longer wavelengths with x. The MAE was observed by a nonlinear change in peak location for the IR bands at 1098 and 1555 cm−1. Different Raman bands of glass samples are approximated as 538, 797, 1040, 1396, and 1514 cm−1. The symmetric stretching vibrations of Ge–O–Ge bonds connected to 3 section rings of GeO4 tetrahedra were assigned to the Raman band centered at 538 cm−1.
Disclosure statement
No potential conflict of interest was reported by the author(s).
Additional information
Funding
References
- Pan Z, Morgan SH. Optical transitions of Er3+ in lead-tellurium-germanate glasses. J Lumin. 1975;75(4):301–308.
- Alqarni AS, Hussin R, Alamri SN, et al. Customized physical and structural features of phosphate-based glass-ceramics: role of Ag nanoparticles and Ho3+ impurities. J Taibah Univ Sci. 2020;14(1):954–962.
- Dietzel AH. J Phys Chem Glasses. 1983;24(6):172–180.
- Srinivas G, Ramesh B, Siva Kumar J, et al. Mixed alkali effect in the physical and optical properties of x K2O-(25-x)Na2O-12.5MgO-12.5BaO-50B2O3glasses. J Taibah Univ Sci. 2016;10(3):442–449.
- Roling B, Ingram MD. Mixed alkaline–earth effects in ion conducting glasses. J Non-Cryst Solid. 2000;265(2):113–119.
- Acharya A, Bhattacharya K, Ghosh CK, et al. Microstructures and charge carrier transport of some Li2O doped glassy ceramics. Mater Lett. 2020;265:127438.
- Ojha S, Roy M, Chamuah A, et al. AC conductivity and dielectric behavior of Cu-S-Te chalcogenide glassy system. Mater Lett. 2020;258:126792.
- Rao NS, Bale S, Purnima MK, et al. Mixed alkali effect in boroarsenate glasses. J Phys Chem Solids. 2007;68(7):1354–1358.
- Deb B, Bhattacharya S, Ghosh A. Broadband conductivity spectra of fast-ion-conducting silver selenite glasses: dependence on power law and scaling. EPL. 2011;96(3):37005.
- Bar AK, Bhattacharya K, Kundub R, et al. Electrical relaxation and grain boundary effect in CdI2 doped glass-nanocomposites. J Non-Cryst Solids. 2016;452:169–175.
- Vegiri A, Varsamis CPE, Kamitsos EI. Molecular dynamics investigation of mixed-alkali borate glasses: short-range order structure and alkali-ion environments. Phys Rev B. 2009;80(18):184202–184212.
- Padmaja G, Kistaiah P. Optical absorption and EPR spectroscopic studies of (30−x)Li2O–xK2O–10CdO–59B2O3–1Fe2O3: An evidence for mixed alkali effect. Solid State Sci. 2010;12(12):2015–2019.
- Subhadra M, Kishtaiah P. Infrared and Raman spectroscopic studies of alkali bismuth borate glasses: evidence of mixed alkali effect. Vib Spectrosc. 2012;62:23–27.
- Moulton Benjamin JA, Henderson GS. Glasses: alkali and alkaline-earth silicates. In: Pomeroy M, editor. Encyclopedia of materials: technical ceramics and glasses, vol. 2. Oxford: Elsevier; 2021. p. 462–482.
- Henderson GS. The germanate anomaly: what do we know? J Non-Cryst Solids. 2007;353:1695–1704.
- Henderson GS, Soltay LG, Wang HM. The germanate anomaly: what do we know? J Non-Cryst Solids. 2010;356:2480–2485.
- Chakraborty IN, Sr RAC. The vibrational spectra of B2O3-GeO2 glasses. J Non-Cryst Solids. 1986;81(2):271–284.
- Xue Y, Cao J, Zhang Z, et al. Manipulating Bi NIR emission by adjusting optical basicity, boron and aluminum coordination in borate laser glasses. J Am Ceram Soc. 2018;101(2):624–633.
- Kumar VS, Reddy BR, Babu S, et al. Thermal, structural and spectroscopic properties of Pr3+-doped lead zinc borate glasses modified by alkali metal ions. J Taibah Univ Sci. 2017;11(4):593–604.
- Gangapur P, Reddy TG, Puram K. EPR spectroscopic studies in (30−x) Li2O–xK2O–10CdO–59B2O3–1MnO2 multi-component glass system. Chem Phys. 2012;394(1):17–20.
- Rahman S, Bale S, Kumar RV, et al. Mixed cation effect in xR2O–(40−x)Na2O–50B2O3–10Bi2O3 (R = Li, K) glasses. Mater Res Bull. 2010;45(10):1533–1536.
- Edukondalu A, Rahman S, Siva Kumar K, et al. Vibrational spectra and structure of mixed alkali borotungstate glasses: evidence of mixed alkali effect. Vibr Spectrosc. 2014;71:91–97.
- Walsh BM, Barnes NP, Reichle DJ, et al. Optical properties of Tm3+ ions in alkali germanate glass. J Non-Cryst Solids. 2006;352(50):5344–5352.
- Chamuah A, Bhattacharya K, Ali MS, et al. Density of states, DC conductivity and physical properties of Ag2S-Ge–Te–Se chalcogenide glassy system. Appl Phys A. 2021;127:656.
- Franco DF, Fernandes RG, Santagneli SH, et al. Structural study of the germanium–aluminum–borate glasses by solid state NMR and Raman spectroscopies. J Phys Chem C. 2020;124(44):24460–24469.
- Liu C, Zhuang Y, Han J, et al. Multi-band near-infrared emission in low concentration bismuth doped alkaline earth alumino-boro-germanate glass. Ceram Int. 2020;46(10):15544–15553.
- Kundu RS, Dult M, Punia R, et al. Titanium induced structural modifications in bismuth silicate glasses. J Mol Struct. 2014;1063:77–82.
- Balaa M, Pawaria S, Deopa N, et al. Structural, optical, thermal and other physical properties of Bi2O3 modified Lithium Zinc Silicate glasses. J Mol Struct. 2021;1234:130160.
- Dult M, Kundu RS, Berwal N, et al. Manganese modified structural and optical properties of bismuth silicate glasses. J Mol Struct. 2015;1089:32–37.
- Motke SG, Yawale SP, Yawale SS. Infrared spectra of zinc doped lead borate glasses. Bull Mater Sci. 2002;25:75–78.
- Krogh-Moe J. J Phys Chem Glass. 1965;6:46.
- Yiannopoulos YD, Chryssikos GD, Kamitsos EI. Phys Chem Glasses. 2001;42(3):164–172.
- Doweider H, El-Egili K, Altawaf A. Structural units and properties of BaF2–PbF2–B2O3 glasses. J Non-Cryst Solids. 2017;464:73–80.
- Sahar MR, Wahab A, Hussein MA, et al. Structural characteristic of Na2OP2O5GeO2 glass systems. J Non-Cryst Solids. 2007;353(11):1134–1140.
- Sigaev VN, Orlova EV, Lotarev SV, et al. Structure and crystallization specifics of alumina-bearing lanthanide borogermanate glass. Glass Ceram. 2006;63:184–189.
- Samee MA, Edukondalu A, Ahmmad SK, et al. J Electron. 2013;42(8):2416–2524.
- Elokr MM, Ghany AA, Hafez FM, et al. Chalcogenide Lett. 2012;9(1):1–9.
- Kamitsos EI, Yiannopoulos YD, Varsamis CP, et al. Structure-property correlation in glasses by infrared reflectance spectroscopy. J Non-Cryst Solids. 1997;222(2):59–68.
- Kamitsos EI, Patsis AP, Chryssikos GD. Infrared reflectance investigation of alkali diborate glasses. J Non-Cryst Solids. 1993;152(2):246–257.
- Liu C, Zhuang Y, Han J, et al. Ceram. 2020;46(10):15544–15553.
- Hwa LG, Shiau JG, Szu SP. Polarized Raman scattering in lanthanum gallogermanate glasses. J Non-Cryst Solids. 1999;249:55–61.
- Henderson GS, Soltay LG, Wang HM. Q speciation in alkali germanate glasses. J Non-Cryst Solids. 2010;356:2480–2485.
- Miyaji F, Saka S. Structure of PbO·Bi2O3·Ga2O3 glasses. J Non-Cryst Solids. 1991;134(1):77–85.
- Meera BN, Ramakrishna J. Raman spectral studies of borate glasses. J Non-Cryst Solids. 1993;159:1–21.
- Koroleva ON, Shtenberg MV, Zainullina RT, et al. Vibrational spectroscopy and density of K2O–B2O3–GeO2 glasses with variable B/Ge ratio. Phys Chem Chem Phys. 2019;21(23):12676–12684.
- Yano T, Kunimine N, Shibata S, et al. Structural investigation of sodium borate glasses and melts by Raman spectroscopy. II. conversion between BO4 and BO2O− units at high temperature. J Non-Cryst Solids. 2003;321(3):147–156.
- Subhashini, Shashikala, HD, Udayashankar NK. Influence of Fe3+ ions on optical, structural, thermal and mechanical properties of Li2O–Na2O–K2O–ZnO–B2O3 based glass system. Ceram. 46 (4) (2020) 5213-5222.