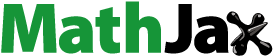
Abstract
The concentration level of a certain metal in soil is often the guideline for evaluating its contamination level. In such a process, ecological standards are the guidelines for assessing ecological risks. In the presence of certain conditions, the risk of contamination can expand beyond the concerned area creating a threat that is based on mobility. Such threat cannot be overlooked and needs to be taken into consideration, as such the work below aims to assess the mobility of metallic trace elements M.T.Es (Pb, Cu, Cd, As, Ni, Cr and Zn) in different soils and mine tailing around an abandoned Pb Zeïda mine in Morocco. The sequential extraction followed a standard BCR four-step procedure and revealed that all M.T.Es were largely associated with the exchangeable and reducible fractions, exhibiting higher mobility susceptibility and bioavailability, except for As. This was consistent with the physico-chemical and mineralogical properties of the local geological composition.
1. Introduction
Contamination by metallic trace elements (M.T.Es) are still one of the most important concerns in mining districts [Citation1–3]. Indeed, M.T.Es could be transferred through mine tailings by different transport mechanisms [Citation4]. They could also accumulate progressively in the soils then persist for a long period of time; Cd, Hg and As for instance can last for 380 years, 1000 years and even 4000 years, respectively [Citation5]. This accumulation of metallic trace elements in the soil is a long-term threat to the environment [Citation6]. Therefore, ecological risk and pollution indices are needed to assess the risk of contamination by metallic trace elements in the soil. Over the last decades, different ecological risk and pollution indices have been developed, such as the Geo-accumulation Index (Igeo) and the Contamination Factor (CF), which can present an integrated overview of soil metallic trace elements contamination by determining either metallic trace elements in the soil as natural phenomena or anthropogenic activities [Citation7].
Previous studies conducted in Zeïda area had been based on preliminary indices, using total content to assess soils contamination, which did not specifically highlight the mobility. In fact, the mobility of M.T.Es was highly dependent on their specific mineralogical fraction (metal oxide, carbonates, to cite just a few), binding and affinities [Citation8,Citation9], which required sequential extraction. BCR Sequential extraction (The Community Bureau of Reference) procedure had been widely used, considering its accuracy, reliability and good recovery values [Citation10]. In this procedure, the first fraction called “extractable” was the fraction of metals weakly bounding to clays (exchangeable fraction), amorphous phases and carbonate fractions. The second fraction called “reducible” was bound to metal oxides while the third fraction called “oxidizable” was bound to organic matter. The remaining fraction was referred to as the residual fraction [Citation11]. The M.T.Es mobility could be also influenced by other essential factors such as cation exchange capacity, conductivity, grain size, mineralogy and so on. These are factors which could promote or prevent the formation of soluble complexes [Citation12,Citation13].
The key objective of the research described in the present article was to assess the mobility of M.T.Es (As, Cd, Cu, Ni, Pb, Zn and Cr) in soils and mining tailing. To achieve this objective, several approaches were combined: (a) measurement of M.T.Es total contents, (b) identification of physico-chemical and mineralogical properties of mine tailing and soils, (c) fractionation in mine tailing and soils using the BCR procedure and (d) identification of M.T.Es sources by a multivariate statistical analysis including PCA, heatmap and correlation analysis.
2. Materials and methods
Zeïda is a village, hence the name of the studied mining area, located in the North-Eastern region of Morocco. The mine is located thirty kilometres west of the city of Midelt, on the banks of the Moulouya River (Figure ). Geologically, the study area was a part of a plumbiferous district of the High Moulouya, its mineralization appeared as stratabound layers in subhorizontal Permo-Triassic arkosic sandstones, unconformably overlying the Palaeozoic basement composed of Hercynian granitoids and metamorphic schist, as shown in Figure S1 (Supplementary Material). The Pb content levels at Zeïda are mainly related to the properties and nature of the arkose enriched in barytic cement, the mineralisation is mainly composed of cerussite (PbCO3) which is mainly found in the Triassic arkoses and sandstones [Citation14]. The paragenetic association consisted mainly of cerussite (70% of extracted Pb), abundant of pink barite and galena, with accessory supergene alteration minerals as anglesite, wulfenite [Citation15]. The local climate in the study area is semi-arid with two temperatures maximum: 6.5°C and 32°C in January and July, respectively, with an average annual rainfall of less than 400 mm. The High Moulouya is characterized by winds, often violent, reaching a maximum speed of 50 m/s.
Four stations were chosen (S1, S2, S3 and S4) aligned along the direction of the most prevailing wind; S1 was the tailing station while S2, S3 and S4 were the soils located, respectively, at a distance of 8, 16 and 20 km from station S1. In each sampling station, three depths 0–1 cm, 1–3 cm and 3–12 cm were chosen. The choice of these depths was based primarily on the different properties of the local climatic context of the study area and on in situ field observation. The first depth (0–1 cm) was very useful to evaluate recent superficial deposits that were more sensitive to erosion, especially that the study area was characterized by year-round winds. Generally, these deposits were presented as a very distinct whitish film, however, the second depth (1–3 cm) was characterized by a red color. The last depth (3–12 cm) was distinguished by a darker color, a slightly hard consistency, and the absence of organic matter and roots. The area was characterized by frequent, short and heavy rainfall, which meant that water did not have time to infiltrate the soil, and thus there was no significant leaching of M.T.Es at great depths, which explained the choice of 12 cm as the final depth. All samples were gathered with a stainless-steel shovel and placed into clear self-sealing polyethylene bags, labeled and transported to the laboratory for further analysis. All samples were air-dried indoors at ambient temperature for four weeks, gently disaggregated and sieved through 2 mm nylon screen to remove stones, coarse materials and plant roots, before a homogenization of the total amount with a porcelain mortar [Citation16].
Soil pH was determined at a soil to water ratio of 1:2.5 using the standard method NF X31-10 [Citation17]. The conductivity (EC) and salinity were measured according to Tian et al. [Citation18] with a soil to water ratio of 1:2.5 using HANNA HI 9828, (Instrument Ltd., Germany). The cation exchange capacity (CEC) method was taken from Rhoades study [Citation19]. The carbonate content (CaCO3) was carried out by the volumetric method (Bernard calcimeter) following the standard (AFNOR NF P94-048 1996) taken from Nasri et al. [Citation20]. Total nitrogen (TN) and organic carbon (CO) were measured using dry combustion (Thermo Flash 2000 NC Analyzer, Austria) according to Harris et al. [Citation21]. Soil organic matter (MO) content is determined by the loss on ignition method [Citation22]. Inorganic carbon (CI) is estimated by the formula 0.12 × [CaCO3] as indicated by Caria et al. [Citation23].
Besides the total mineralogical fraction of soil, clay fraction was further studied to identify the principal clay minerals using a Philips X’Pert X-ray diffractometer- Holland in configuration θ–2θ under the following conditions: CuKα radiation, 40 kV, 45 mA, rotation speed of 1rps, step scanning at 0.02°/1.00 s per step in the range 3°–70° (2θ). These properties were measured in El Servicio Interdepartamental de Investigación de la Universidad Autónoma de Madrid, Spain (SIdI-UAM).
The metallic trace elements (As, Cd, Cu, Cr, Pb, Zn and Ni) were determined using inductively coupled mass spectrometry ICP-MS, after microwave-assisted aqua regia (1 HNO3 and 3 HCl) digestion by UltraWAVE microwave, Milestone Co. The BCR four-step sequential extraction procedure was adopted in this work [Citation24]. Its scheme consisted of four extraction steps outlined below B1 through B4: B1, fraction soluble/exchangeable in weak acid, extraction with 0.11 mol/l acetic acid; B2, fraction associated with Fe-Mn oxy/hydroxides, extraction with 0.5 mol/l hydroxylamine hydrochloride at pH 1.5; B3, oxidizable, sulphide and/or associated organic fraction, reaction with 8.8 mol/l H2O2 followed by extraction with 1.0 mol/l ammonium acetate at pH 2, and finally B4, residual fraction, which was calculated by subtracting the non-residual fraction from the total metal contents. At the end of each procedure, the leachate was removed from the mixture with a syringe and immediately filtered through a 0.45 μm Millipore syringe filter membrane, and then the solutions were stored at 4°C. The metal contents in the filtrates of total and sequential extractions of samples were submitted to the SIdI-UAM to be analyzed by ICP-MS NexION 300XX (Perkin Elmer Inc., Hopkinton, MA, USA).
The contamination factor (CF) (Equation 1) and the geo-accumulation Index (Igeo) (Equation 2) both indicated anthropogenic contribution to metal pollution. The equations for this part can be found in Table S2 (Supplementary Material). The potential leaching risk was estimated through the concentration of leached M.T.Es. This index was calculated from the metal trace elements content and the leached portion (η) of different species of metallic trace elements, using the following equation [Citation18].
(3)
(3) Where, Ci and ηi are the M.T.Es content and the “leached portions” of four different M.T.Es fractionations respectively. In this work, the “leached portions” η1, η2, η3, and η4 values of every metal were employed based on the works of Bao et al. [Citation25].
In the present work, the correlation matrix between M.T.Es content and physicochemical properties of soils and mine tailing was based on the Pearson’s correlation coefficients using R software 3.3.1. The heatmap combined with clustering method was used in this work, to group samples together based on the similarity of their geochemical proprieties. These were properties such as pH, clay, silt, total M.T.Es contents (Pb, As, Cu, Cd, Ni, Cr and Zn), their fractionations (B1, B2, B3) and their leaching risk potential in the tailing and soils. This kind of grouping served well in identifying properties that are commonly associated with tailing and soils using the R [Citation26].
3. Results and discussion
3.1. Textural and physicochemical properties
According to Brady and Weil [Citation27], the tailing had a silty clay loam texture; this is due to the fact that it is a relatively finely ground and crushed material, so no particles larger than 2 mm in diameter were found in the tailing samples, these results were also found in the Argane [Citation28] study. This is in contrast to the soil samples which generally have a sandy texture that becomes more prominent towards the Hassan II dam (downstream) as was outlined in Table . The pH of the tailing samples was alkaline with an average of 8.5. This alkalinity was due to the geology of the study area and also to the mined ore which was rich in carbonates in the form of lead carbonate (PbCO3) that often favored the retention of most metallic trace elements by precipitation and adsorption [Citation29,Citation30]. The pH of the soils samples showed neutral to slightly alkaline values with an average of 7.9 mainly due to the abundance of silicates detected in the soils of the analyzed samples. These results were in perfect agreement with those of El Hachimi et al. [Citation31]. The average CEC values were 2.1–2.8 cmol/kg for the tailing and downstream soils, respectively. These values were low (≈ 2.5 cmol/kg) and were consistent with predominantly silty soils characterized by fairly easy exchange [Citation32]. The CE had an average of 157.4 and 129.7 μs/cm in tailing and soils, respectively, this was in agreement with the results found by El Azhari et al. [Citation33]; El Hachimi et al. [Citation31]. The high pH values and average EC values are mainly due to the limestone environment. These results were similar to those obtained at the Ahangaran Pb-Zn mine in Iran [Citation30] and the San Quentin Pb-Zn mine in Spain [Citation34]. The CO, TN and MO showed the same pattern along the stations; they were very low in tailing (S1) with average values of 0.7, 0.1 and 0.91 g/kg, respectively, while they increased in the soils (S2, S3 and S4), with average values of 4.2, 0.4 and 6.97 g/kg. These results were consistent with the fact that the mine tailing came from different steps of ore processing and was, therefore, an ultimate, hostile and highly mineralized material that could not contain nutrients, whereas the downstream soils were relatively rich in fauna and flora and were, therefore, more enriched in CO, TN and MO. These results were in line with those already obtained by Iavazzo et al. [Citation15]. The maximum values of CaCO3 and CI were 31.9 and 3.6 g/kg while the minimum values were 11.6 and 1.5 g/kg, respectively. The low carbonate levels were noted in the tailing and this may be attributed to the granitic and sandstone composition of the host rock. In addition, the abundance of schist and granite in the geological outcrops of the sample area may account for the relatively low carbonate content in most soils. Vertically, pH, CO, TN and MO values decreased slightly from surface to depth in all stations, while CaCO3 and CI increased slightly. These results agree with the study by Iavazzo et al. [Citation15]. The CE and CEC values differed between stations.
Table 1. Physico-Chemical properties and total content of metallic trace elements of studied mine tailing and soils.
3.2. Total contents of metallic trace elements
The horizontal distribution of Cd, Pb, Cu, Zn and As showed a similar spatial distribution in all stations. The highest values were detected in the tailing area and the lowest ones were observed far away from there: S1>>>> S2> S3> S4 in most cases. Contrary to the metals mentioned above, the peak contents of Ni and Cr was not in the tailing, they showed a varied distribution throughout the area. The M.T.Es concentration was compared to three soil quality guidelines (Table S1); the reference station (SR), which was sampled 4.5 km northeast of the mine site, the geochemical background calculated by normal Q-Q plots from the Zeïda area [Citation33]. As well as Bowen’s global average of uncontaminated soils [Citation35]. The results indicated that the average concentrations of M.T.Es (Pb, As, Cu, Zn, Cd, Cr, and Ni) in the tailing were about 315, 14, 5, 4, 1, 1, and 1 times higher than the soil quality guidelines. The Pb concentration was abnormally high in the tailing as it was the metallic signature of the past mining. This was similar to the Faj Lahdoum mine in northwestern Tunisia, which had average Pb concentrations of 10,460 mg/kg in its tailings [Citation36]. These concentrations were in the same range as those in our study. In soils only, the average concentration of Zn, Pb, Cr and Ni was about 1 time higher than the soil quality guidelines. This indicated that the concentration level of all M.T.Es, except Ni and Cr, decreased with increasing distance from the tailing and tended to return to background geochemical level, indicating that their presence was directly related to tailing and therefore these M.T.Es were of anthropogenic origin; mining. These results were similar to those obtained at the Au-Ag mine in Imcheon, Korea [Citation37] and the Pb-Zn mine in You’xi in southeast China [Citation38].
As far as the vertical distribution is concerned, the As total content increased with depth in all sampling sites, unlike Pb’s content which decreased. The Cd and Zn content varied from one depth to the other and from one station to the other. As for Cu, the total content increased with depth for all sampling sites except in tailing, where things were quite different. It increased in the first and second depths (0–3 cm) then decreased in the next one (3–12 cm). The content of Ni and Cr decreased with depth in tailing (S1), however, it increased in areas far from it. Over all, the vertical distribution of Ni, Cr and Cu in the tailing appeared antagonistic with that of the other stations.
3.3. Mineralogical analyses
A total of 12 crystalline phases were identified and quantified in the samples from the Zeïda area (Figure ). The Zeïda tailing (S1) was particularly rich in K-feldspars (14% on average) and quartz (42%). It should also be noted that, due to their low content, sulphide minerals were not detected by X-ray diffraction. Most of the sulfur analyzed was in the form of barite (BaSO4) in the three depths of the mine tailing with an average of 11%. The cerussite (9%) was the major host mineral of Pb in its structure with 9% of schultenite. The clay minerals represented 13% which included 5%, 1% and 7% of montmorillonite, illite and kaolinite, respectively. The S2 and S3 were characterized by 61% of quartz, 1% of hematite, a moderate value of K-feld and NaCa-feld and 3% of phyllosilycates. The last station (S4) which was near the dam, was characterized by the appearance of minerals such as magnetite (8%) and hematite (1%). The phyllosilycate level increased in relation to depth with an average of 7% of illite, 1% of kaolinite and 1% of chlorite which was unique to this station. Furthermore, the station was characterized by an average of 49% of quartz, 12% K-feld and 21% of NaCa-feld.
3.4. Metallic trace elements fractionation
Figure depicted the percentages of M.T.Es distributed in the acid soluble (B1), reducible (B2), oxidizable (B3), and residual (B4) fractions of tailing and soils (S2 + S3 + S4) downstream the mining district.
Figure 3. Percentage distribution of M.T.Es in the chemical fractions sequentially extracted from studied mine tailing and soils.
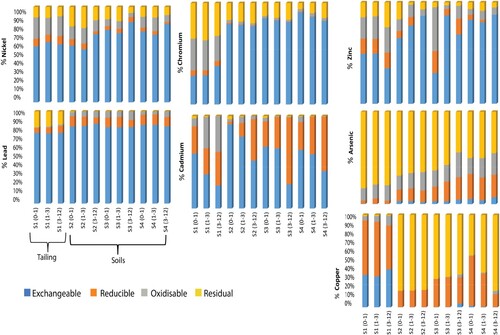
The percentage of As content into the various fractions followed the order B4 >> B3 > B2 > B1.
The distribution of As showed a stronger presence in the residual fraction (B4) for all stations; more than 80% of As in tailings (S1) and 57% in soils were presented in B4 with an average of 62.46 and 1.5 mg/kg, respectively. These results are in line with the work of Wenzel et al. [Citation39], who found that about 80% of As was present in residual form in mine contaminated soils. Kim et al. [Citation40] attributed this to the fact that As was bound to the crystalline matrices of silicates, which were refractory soil components and could only be dissolved by strong acids. This high proportion fraction indicated the low mobility and bioavailability of As in the environment [Citation41]. An average of 15% and 20% of As bound to organic substances and sulfides was released in tailing and soils, respectively. Rates of 4% and 19% of As were extracted in the tailings and downstream soils, respectively in the fraction B2, indicating the low tendency of As to form stable complexes with Fe oxy/hydroxides. The exchangeable fraction represented only minor portions of the total concentration; less than 1% for station S1 and less than 4% for downstream soils, with an average of 0.30 and 0.1 mg/kg, respectively. This suggested relatively very low mobility and bioavailability of this element.
The percentage of Pb content into the various fractions followed the order B1>>B4 > B2 > B3. In tailing (S1), a very high relative amount of 76% was mainly present in the exchangeable fraction (B1). This fraction was labeled as the most mobile, and therefore was the most dangerous for the environment [Citation11]. It is also important to note, that an anthropogenic source was behind the predominance of this fraction [Citation42]. The fraction with the next highest relative amount of 17% was the residual fraction (B4). The relative amounts of Pb associated with the two remaining fractions were very small: 6% of reducible and 1% of oxidizable bounds. Far from tailing, B1 fraction was about 84% and the residual fraction was negligible (0%), while reducible and oxidizable bounds increased to 10% and 5%, respectively.
The Cd in the study area was mainly in the carbonate and exchangeable fraction with about 41% in the tailing (S1) and 58% in the downstream soils. This was followed by the reducible fraction with about 31% in the tailing (S1) and 39% in the downstream soils. Followed the oxidizable fraction with 26% in the tailing (S1) and 2% in the downstream soils. The results indicated that a considerable amount of Cd was bound to the non-residual fractions and thus could [Citation43] mobilized under appropriate environmental conditions and become bioavailable; easily taken up by plants growing in the soils and thus be toxic [Citation44]. Only a minor portion of the total Cd, not exceeding 1%, has an affinity for the residual fraction. The percentage of Cd content into the various fractions followed the order B1 > B2 > B3 > B4.
For Cu, the exchangeable fraction (B1) and oxidizable fraction (B3) were considerably present at the tailing station with an average percentage of 36% and 7% respectively, but at the soils stations (S2, S3 and S4), these fractions decreased to 1% and became negligible. The reducible fraction also decreased moderately from 55% in tailing to 27% in soils, indicating a significant presence of Cu in the oxide or hydroxide form. This suggested that non-residual fractions were decreasing from Station S1 to downstream soils, which revealed that Cu was unstable and potentially mobile and bioavailable in the tailings under certain environmental circumstances [Citation45]. While the residual fraction increased from 1% to 71% of the total Cu content, implying that Cu became more and more stable far from the tailing. The percentage of Cu content into the various fractions followed the order B2 > B1 > B3 > B4.
A large portion of Ni, 61% in tailing and 74% in soils was extracted in the exchangeable and carbonate fraction which could be easily released in case of changes in pH, redox potential, salinity, etc. [Citation46]. A significant amount was also found in the organic bound fraction with 20% in tailing and 10% in soils. Nearly 11% in tailing and 12% in soils of Ni associated with the residual fraction (B4). The extracted Ni content bounded to Fe-Mn oxide fraction was the weakest with an average of 8% in tailing and 4% in soils. The percentage of Ni content into the various fractions followed the order B1 > B3 > B4 > B2.
Far from the tailing, the fractionation indicated that major portion of Cr was associated with the exchangeable fraction (B1) with 82%, this fraction was labile and could enter the food chain [Citation47,Citation48]. Followed by residual fraction (B4) with 15%, while the remaining fractions (reducible B2 and oxidizable B3 fractions) were negligible. In tailing, the exchangeable fraction decreased, whilst the other fractions increased with a 35%, 31%, 29% and 5% for B4, B1, B3 and B2, respectively.
The sequential extraction procedure showed that Zn was strongly associated with the exchangeable fraction (B1) with an average of 46% in tailing and 77% in soils. This fraction was considered the most mobile and bioavailable, as the associated trace metals were weakly bound to the soil and could be released into the environment at any physicochemical change [Citation49]. The oxidizable fraction (B3) also held another 22% in tailing and 11% of the total Zn in the remaining stations. The residual fraction (B4) had an extraction rate of 17% in tailing and 6% in other stations. Almost similar proportions were recorded for the reducible fraction (B2). The percentage of Zn content into the various fractions followed the order B1 > B3 > B4 = B2. In the majority of soils and tailing samples studied, Pb and Zn were mainly concentrated in the non-residual fractions (mainly the exchangeable fraction), this was in line with other studies [Citation15,Citation34,Citation50,Citation51].
3.5. Pollution indexes and potential leaching risk
A reference station (SR) was considered as the soil geochemical background in the study area. The measured metals contents of the SR in this work were in fact consistent with those determined by El Azhari et al. [Citation33] using the normality curves method, as shown in Table S1 (Supplementary Material). According to Table , the contamination factor of Cr, Ni and Cd was <2 implying a null to average contamination for all samples (tailing and soils) at different depths, contrary to contamination factor of Pb and Zn which far exceeded the limit value. The contamination factor of Cu and As was >6 in the tailing (S1) while it was <1 in the soils; S2 through S4. In accordance with the results of the contamination factor, the geo-accumulation index revealed that the study area was heavily polluted by Pb and Zn; the tailing was classified as both extremely polluted and moderately polluted; moreover, the soils were classified as moderately to heavily polluted, and unpolluted to moderately polluted by Pb and Zn, respectively. On the other hand, the geo-accumulation index of Cr, Ni and Cd was below zero throughout the area, indicating that the area was unpolluted. The residue was moderately to heavily polluted by Cu and As, while the soils were not polluted by these two metals. The potential leaching risk of Pb, Cu, Cd and Zn was 99%, 95%, 85% and 42%, respectively, in the tailing. While the potential leaching risk of Cr and Ni was 12% and 21% which were lower values compared to other stations (Table ).
Table 2. Variations in ecological indices of metallic trace elements at the mine tailing and soils.
3.6. Intra-relationships between variables
A basic Pearson’s correlation study was performed in order to understand the relationships between the physico-chemical parameters and the total M.T.Es concentrations. As given in Figure b, a strong positive correlation was identified between Pb, As, Cu, Zn and Cd which might reflect a similarity in pollution level and sources. Additionally, there was a high to medium correlation between the M.T.Es above and the silty, clay fractions, pH and EC. These results were consistent with the results of studies conducted worldwide, which showed that the fine-grained fraction has a higher tendency to adsorb M.T.Es than the coarse-grained fraction, because it contained particles with large surface areas such as clay minerals and iron and manganese oxyhydroxides [Citation52,Citation53]. All of the above mentioned parameters were significantly negatively correlated with sand, OM and NT, showing that increasing M.T.Es (Pb, As, Cu, Zn and Cd), silt and clay fractions, pH and EC will reciprocally generate a decrease in sand, OM and NT. Both Ni and Cr were negatively and weakly correlated with all other T.M.E.s, respectively. This is justified by the lateral distribution behavior of M.T.Es; concentrations of Pb, Zn, Cu, Cd and As decreased from upstream (tailing) to downstream (Hassan II dam), while Cr and Ni levels increased. Both Ni and Cr were also moderately correlated with each other and with MO, CEC, CI, NT and CaCO3.
Figure 4. Multivariate statistical analyses of M.T.Es and physico-chemical properties, in studied mine tailing and soils: Pearson’s correlation matrix (a) and principal component analyses (PCA) (b).
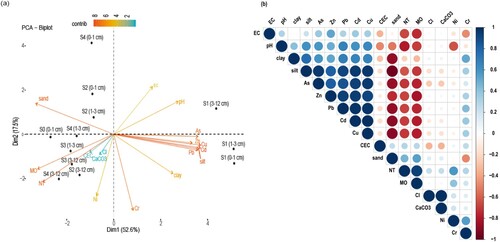
The PCA results (Figure a) revealed that the DM1 and DM2 dimensions explained 70% of the total variability in our scatterplot, which was sufficient to provide an acceptable view of the data structure. The Pb, As, Cu, Cd, Zn and silts were strongly correlated with the DM1, which explains 52.6% of the total variability, and formed an individualized group. This result was consistent with the results of Sigg et al. [Citation54]; El Ganaoui [Citation55]; Lions [Citation56]; Yao et al. [Citation53] and Maslennikova et al. [Citation57], indicating that the fine fraction played a major role in M.T.Es retention. This fine fraction could be transported easily under the climatic conditions of the study area and thus control metal pollution in the environment. The Clay, pH and EC were moderately correlated with this dimension, while sand, MO and NT were strongly negatively correlated with it. The M.T.Es (Pb, As, Cu, Cd, Zn) presented in DM1 tend to be clearly associated with anthropogenic activities related to mining, mainly because at Zeïda, the mined ore body (Arkose) was mainly composed of cerussite, galena and other accessory minerals. Therefore, Pb and Zn are considered to be the metallic signature of the Zeïda tailings. This is also supported by the fact that high concentrations of these M.T.Es are detected in the tailings and with distance, the concentrations decreased and returned to their local geochemical background equivalents. Which was also consistent with the relatively high contamination factor and potential leaching risk values of these metals. Both Ni and Cr were associated with DM2 which reflected the contributing role of natural geological sources. This was because their values were close to the local geochemical background for both soils and residues. The contribution of CEC, CaCO3 and CI was extremely low. This is due to their homogeneity throughout the area – from tailings (S1) to downstream (S4).
3.7. Hierarchical clustering
The heatmap was a correlation matrix combined with the hierarchical clustering method. Negative correlations are colored blue and positive correlations are colored red. This was a method of simultaneous visualization of station clusters and geochemical properties clusters. This could be useful for identifying properties that control the mobility, release and bioavailability of M.T.Es in soils from tailing and downstream soils. Figure showed that the stations could be divided into two clusters. Cluster A was characterized by samples from mine tailing that was very heavily polluted by the former mining activity. Cluster B was composed of soils located downstream of the Zeïda tailing, as indicated on the location map. This area was moderately to weakly affected by mining activity compared to Cluster A. In abscissa, the parameters were divided into two clusters C and D; Cluster D was divided into two sub-clusters: E including MO, CO, NT, sand and CEC. And F contained carbonates, CI, Cr and Ni. The two sub-clusters E and F became increasingly important as they moved away from the tailing. Cluster C included high total M.T.Es concentrations (Pb, Cu, Zn, Cd, and As), predominance of mobile and bioavailable metal fractions (B1, B2, and B3), high leaching risk potentials with silt, clay, pH, and EC. This cluster was more dominant in the tailing than in the soils, suggesting their relationship to mining activities. It can be said that clusters A and E combined tailing and the geochemical properties that control it. Clusters B and D included downstream soils and the geochemical properties that control them.
4. Conclusion
Abandoned mine tailings rich in trace metals can become a very important source of pollution of different environmental matrices (water, sediment, soil, plants … .). Therefore, the physico-chemical characterization of tailings and adjacent soils is important to assess the risk of potential environmental mobility of metallic trace elements. The results indicated that all samples presented a neutral to alkaline pH, this could be attributed to the geology of the study area and also to the mined ore which was rich in carbonates in the form of lead carbonate (PbCO3). The results indicated that the high concentration of Pb, Cu, Cd, As and Zn was found in the tailing (S1) and that concentration decreased with an increase in distance, as follows: S1>> S2 > S3 > S4 in most cases. The ecological risk and pollution indices used in this work indicated that tailing was intensely polluted by Pb and Zn. Sequential extraction results revealed the importance of the exchangeable fraction (i.e. the most mobile and bioavailable fraction present in tailing and soils): it exceeded 60% for Zn, Cr, Ni, and Cd, with a maximum of >70% for Pb and a minimum of <10% for As and Cu. This indicated potentially high leaching risk of Pb, Zn, Cr, Ni and Cd which meant that these metals could be easily released in case of changes in pH, redox potential, salinity, etc. In conclusion, this study -through its results- highlighted the urge to treat the tailing dumped in the old mine area. Most importantly, what amplifies this urge is the seriousness of the situation as it is in its current state, and as a lurking risk that is about to happen.
Supplemental Material
Download MS Word (92 KB)Disclosure statement
No potential conflict of interest was reported by the author(s).
References
- Coskun A, Horasan BY, Ozturk A. Heavy metal distribution in stream sediments and potential ecological risk assessment in Konya Northeast region. Environ Earth Sci. 2021;80(5):1–18.
- Gemici Ü, Tarcan G, Somay AM, et al. Factors controlling the element distribution in farming soils and water around the abandoned Halıköy mercury mine (Beydağ, Turkey). Appl Geochem. 2009;24(10):1908–1917.
- Horasan BY. The environmental impact of the abandoned mercury mines on the settlement and agricultural lands; Ladik (Konya, Turkey). Environ Earth Sci. 2020;79(10):1–13.
- Chen H, Chen Z, Chen Z, et al. Calculation of toxicity coefficient of potential ecological risk assessment of rare earth elements. Bull Environ Contam Toxicol. 2020;104(5):582–587.
- Higueras P, Esbrí JM, García-Ordiales E, et al. Potentially harmful elements in soils and holm-oak trees (Quercus ilex L.) growing in mining sites at the Valle de Alcudia Pb-Zn district (Spain)–Some clues on plant metal uptake. J Geochem Explor. 2017;182:166–179.
- Hanfi MY, Yarmoshenko IV. Health risk assessment quantification from heavy metals contamination in the urban soil and urban surface deposited sediment. J Taibah Univ Sci. 2020;14(1):285–293.
- Antoniadis V, Levizou E, Shaheen SM, et al. Trace elements in the soil-plant interface: phytoavailability, translocation, and phytoremediation–a review. Earth Sci Rev. 2017;171:621–645.
- Li J, Kosugi T, Riya S, et al. Pollution potential leaching index as a tool to assess water leaching risk of arsenic in excavated urban soils. Ecotoxicol Environ Saf. 2018;147:72–79. https://doi.org/10.1016/j.ecoenv.2017.08.002.
- Zhao B, Xing C, Zhou S, et al. Sources, fraction distribution and health risk assessment of selenium (Se) in Dashan village, a Se-Rich area in Anhui Province, China. Bull Environ Contam Toxicol. 2020;104(4):545–550.
- Rauret G, López-Sánchez JF, Sahuquillo À, et al. Improvement of the BCR three step sequential extraction procedure prior to the certification of new sediment and soil reference materials. J Environ Monit. 1999;1:57–61.
- Pasquet C, Monna F, van Oort F, et al. Mobility of Ni, Co, and Mn in ultramafic mining soils of New Caledonia, assessed by kinetic EDTA extractions. Environ Monit Assess. 2018;190(11):638. doi:10.1007/s10661-018-7029-0.
- Cappuyns V, Swennen R. Evolution of element release during oxidation and its relevance towards the management of contaminated sediments. J Comun Geol. 2013;100(1):41–47.
- Zhang C, Yu Z-G, Zeng G-M, et al. Effects of sediment geochemical properties on heavy metal bioavailability. Environ Int. 2014;73:270–281.
- Oukemeni D, Bourne JH. Etude géochimique des granitoïdes du pluton d’Aouli, Haute Moulouya, Maroc. J Afr Earth Sci. 1993;17(4):429–443. https://doi.org/10.1016/0899-5362(93)90002-8.
- Iavazzo P, Adamo P, Boni M, et al. Mineralogy and chemical forms of lead and zinc in abandoned mine wastes and soils: an example from Morocco. J Geochem Explor. 2012;113:56–67. https://doi.org/10.1016/j.gexplo.2011.06.001.
- NF ISO 11464. Qualité des sols, prétraitement pour analyses physico-chimiques. NF X31-412/NF ISO 11464 décembre 2006; Ed (2006 Dec 1).
- Chaplain V, Défossez P, Delarue G, et al. Impact of lime and mineral fertilizers on mechanical strength for various soil pHs. Geoderma. 2011;167:360–368.
- Tian H, Fang L, Duan C, et al. Dominant factor affecting Pb speciation and the leaching risk among land-use types around Pb-Zn mine. Geoderma. 2018;326:123–132.
- Rhoades J. Cation exchange capacity, Methods of soil analysis: part 2 chemical microbiological properties. Agronomy. 1983;9:149–157.
- Nasri H, Elhammouti K, Azdimousa A, et al. Calcimetric and sedimentometric characterization of clay deposits in the Neogene Boudinar basin (north eastern Rif, Morocco): implication on the eustatic and hydrodynamic evolution of the basin and economic interest. J Mater Environ Sci Technol. 2016;7:859–870.
- Harris D, Horwáth WR, Van Kessel C. Acid fumigation of soils to remove carbonates prior to total organic carbon or CARBON-13 isotopic analysis. Soil Sci Soc Am J. 2001;65(6):1853–1856.
- Blinda M. (2007). Pollution tellurique du littoral Nord-Ouest du Maroc entre Tanger et Tétouan: Caractérisation, impact sur l’environnement et proposition de solutions.
- Caria G, Proix N, Ciesielski H. Dosage du carbone organique par combustion sèche après décarbonatation automatisée des sols. Journal Institut National de la Recherche Agronomique; 2007.
- Liu M, Han Z. Distribution and bioavailability of heavy metals in soil aggregates from the Fenhe river Basin, China. Bull Environ Contam Toxicol. 2020;104(4):532–537.
- Bao J, Wang L, Xiao M. Changes in speciation and leaching behaviors of heavy metals in dredged sediment solidified/stabilized with various materials. Environ Sci Pollut Res. 2016;23(9):8294–8301.
- Kumar V, Parihar RD, Sharma A, et al. Global evaluation of heavy metal content in surface water bodies: a meta-analysis using heavy metal pollution indices and multivariate statistical analyses. Chemosphere. 2019;236:124364.
- Brady NC, Weil RR. The nature and properties of soils . Vol. 13Upper Saddle River, NJ : Prentice Hal; 2008. p. 662–710.
- Argane R. Valorisation des rejets miniers à faible teneur en sulfures comme granulats pour mortiers [doctoral dissertation]. Lyon: INSA; 2015.
- Ma X, Zuo H, Tian M, et al. Assessment of heavy metals contamination in sediments from three adjacent regions of the Yellow River using metal chemical fractions and multivariate analysis techniques. Chemosphere. 2016;144:264–272.
- Rafiei B, Khodaei AS, Khodabakhsh S, et al. Contamination assessment of lead, zinc, copper, cadmium, arsenic and antimony in Ahangaran mine soils, Malayer, West of Iran. Soil Sediment Contam. 2010;19(5):573–586.
- El Hachimi ML, El Hanbali M, Fekhaoui M, et al. Impact d’un site minier abandonné sur l’environnement: cas de la mine de Zeïda (Haute Moulouya, Maroc). Bulletin de l’institut Scientifique, Rabat, section Sciences de la Terre. 2005;27:93–100.
- Blanchard C. Caractérisation de la mobilisation potentielle des polluants inorganiques dans les sols pollués [doctoral dissertation]. Lyon: INSA; 2000.
- El Azhari A, Rhoujjati A, El Hachimi ML, et al. Pollution and ecological risk assessment of heavy metals in the soil-plant system and the sediment-water column around a former Pb/Zn-mining area in NE Morocco. Ecotoxicol Environ Safe. 2017;144:464–474.
- Rodríguez L, Ruiz E, Alonso-Azcárate J, et al. Heavy metal distribution and chemical speciation in tailings and soils around a Pb–Zn mine in Spain. J Environ Manage. 2009;90(2):1106–1116.
- Bowen H. Environmental chemistry of the elements. Journal Environmental chemistry of the elements. New York: Academic Press; 1979.
- Nouairi J, Rocha F, Medhioub M. Geobiological assessment of the pollution effect of abandoned mine ores (Fej Lahdoum, Northwest Tunisia). Arab J Geosci. 2019;12(24):1–12.
- Jung MC. Heavy metal contamination of soils and waters in and around the Imcheon Au–Ag mine, Korea. Appl Geochem. 2001;16(11-12):1369–1375.
- Lü J, Jiao W-B, Qiu H-Y, et al. Origin and spatial distribution of heavy metals and carcinogenic risk assessment in mining areas at You’xi County southeast China. Geoderma. 2018;310:99–106.
- Wenzel WW, Kirchbaumer N, Prohaska T, et al. Arsenic fractionation in soils using an improved sequential extraction procedure. Anal Chim Acta. 2001;436(2):309–323.
- Kim EJ, Yoo J-C, Baek K. Arsenic speciation and bioaccessibility in arsenic-contaminated soils: sequential extraction and mineralogical investigation. Environ Pollut. 2014;186:29–35.
- Amaibi PM, Entwistle JA, Kennedy N, et al. Mineralogy, solid-phase fractionation and chemical extraction to assess the mobility and availability of arsenic in an urban environment. Appl Geochem. 2019;100:244–257.
- Harrison RM, Laxen DP, Wilson SJ. Chemical associations of lead, cadmium, copper, and zinc in street dusts and roadside soils. Environ Sci Technol. 1981;15(11):1378–1383.
- Wang Y, Yang Z, Shen Z, et al. Assessment of heavy metals in sediments from a typical catchment of the Yangtze River, China. Environ Monit Assess. 2011;172(1):407–417.
- Mahanta MJ, Bhattacharyya KG. Total concentrations, fractionation and mobility of heavy metals in soils of urban area of Guwahati, India. Environ Monit Assess. 2011;173(1):221–240.
- Sun Z, Xie X, Wang P, et al. Heavy metal pollution caused by small-scale metal ore mining activities: a case study from a polymetallic mine in South China. Sci Total Environ. 2018;639:217–227.
- Huang J, Huang R, Jiao JJ, et al. Speciation and mobility of heavy metals in mud in coastal reclamation areas in Shenzhen, China. Environ Geol. 2007;53(1):221–228.
- Jain C, Malik D, Yadav R. Metal fractionation study on bed sediments of Lake Nainital, Uttaranchal, India. Environ Monit Assess. 2007;130(1):129–139.
- Turki A. Metal speciation (cd, cu, Pb and Zn) in sediments from Al Shabab lagoon, Jeddah, Saudi Arabia. Marine Scienes. 2007;18(1):191–210.
- Tack F, Verloo MG. Chemical speciation and fractionation in soil and sediment heavy metal analysis: a review. Int J Environ Anal Chem. 1995;59(2-4):225–238.
- Esshaimi M, El Gharmali A, Berkhis F, et al. Speciation of heavy metals in the soil and the mining residues, in the zinclead Sidi Bou Othmane abandoned mine in Marrakech area. Linnaeus Eco-Tech. 2010;10:975–985.
- Mufalo W, Tangviroon P, Igarashi T, et al. Solid-Phase Partitioning and Leaching Behavior of Pb and Zn from Playground Soils in Kabwe, Zambia. Toxics. 2021;9(10):248.
- Bradl H, Kim C, Kramar U, et al. Interactions of heavy metals. Interface Sci Technol. 2005;6:28–164.
- Yao Q, Wang X, Jian H, et al. Characterization of the particle size fraction associated with heavy metals in suspended sediments of the Yellow River. Int J Environ Res Public Health. 2015;12(6):6725–6744.
- Sigg L, Behra P, Stumm W. Chimie des milieux aquatiques. 5e édition. Paris: Dunod; 2001.
- El Ganaoui O. Modélisation de la dynamique sédimentaire pour les transferts des radionucléides dans les cours d’eau [doctoral dissertation]. Aix-Marseille 2; 2002.
- Lions J. Etude hydrogéochimique de la mobilité de polluants inorganiques dans des sédiments de curage mis en dépôt: expérimentations, suivi in situ et modélisations [doctoral dissertation]. Paris: ENMP; 2004.
- Maslennikova S, Larina N, Larin S. The effect of sediment grain size on heavy metal content. J Lakes Reserv Ponds. 2012;6(1):43–54.