Abstract
In the present study, different parameters, affecting the growth of Thermomyces lanuginosus BJMDU1 and extracellular synthesis of silver nanoparticles (AgNPs), were studied. Culture extract of thermophilic mould Thermomyces lanuginosus BJMDU1 showed potential in green synthesis of silver nanoparticles when the fungus was grown in a sucrose yeast extract medium (pH 8.0) at 45°C. Mycosynthesis of AgNPs was enhanced in the presence of light using 2 mM AgNO3 and 200 µl of culture extract at 50°C. The reaction parameters significantly improved the synthesis of AgNPs. Techniques like UV-Vis & FT-IR spectroscopy, X-ray diffraction, transmission electron microscopy and dynamic light scattering were used to characterise AgNPs. Biogenic nanoparticles showed an absorption peak at 430 nm having 80 nm size. Biogenic AgNPs showed potent antibacterial and antiplasmodial activity with no haemolysis up to 40 µg/ml. The p-nitrophenol was catalytically reduced to p-aminophenol by AgNPs. Therefore, green mycosynthesised AgNPs could be used in the therapeutics and remediation of environmental pollutants.
1. Introduction
Nanotechnology is an emerging field of modern research involving the synthesis and applications of particles ranging from 1-100 nm [Citation1]. Its importance is rapidly increasing in several sectors such as cosmetics, health care, food and feed, chemical industries, biomedical sciences, drug delivery, electronics, biolabeling, and other applications [Citation1, Citation2]. The biological synthesis of metal nanoparticles is preferred globally to overcome the limitations associated with chemical and physical processes, which are costly, toxic, and hazardous to human health [Citation3]. The biological methods are clean, green, eco-friendly, non-hazardous, and inexpensive, attracting researchers for green nanobiotechnological applications [Citation1, Citation2].
Bacteria [Citation3], fungi [Citation4], and plants [Citation5–8] are utilized for the biological synthesis of silver nanoparticles (AgNPs). Among all sources, filamentous moulds have been used for the high synthesis of NPs, as they produce many enzymes extracellularly including reductases [Citation5, Citation9]. There are many reports on the synthesis of AgNPs using mesophilic fungi like Penicillium spp [Citation10], Phanerochaete chrysosporium [Citation11], Aspergillus oryzae MTCC 1846 [Citation12], A. fumigatus BTCB10 [Citation13], Trichoderma harzianum [Citation14] and other related fungi [Citation15]. However, the reports on thermophilic fungi are very scanty compared to their mesophilic counterparts [Citation16–18]. Biosynthesis of AgNPs mainly occurs through extracellular and intracellular enzymes. In extracellular nanoparticle synthesis, the metal solution is mixed with the aqueous filtrate of the fungal culture, which reduces silver ions into NPs [Citation17]. In intracellular synthesis, the metal is added to the culture medium that intercalates into the biomass, and extraction of NPs is required after the synthesis using physical and chemical methods [Citation19]. Therefore, the extracellular method is widely used due to the lack of processes employed to release the nanoparticles from the cells [Citation17]. Thermophilic fungi secrete large amounts of enzymes, which reduce a wide variety of metals into nanoparticles such as gold, silver, iron oxide, and others [Citation15, Citation16, Citation18]. The metabolites and enzymes secreted by thermophilic moulds are highly thermostable and can be used for catalysis at higher temperatures as compared to other sources like plants and mesophilic microorganisms [Citation9]. Therefore, thermophilic fungi are a promising source for the green synthesis of AgNPs for various biotechnological applications. Few researchers have reported the synthesis of silver NPs by thermophilic moulds like Humicola sp. and these biogenic AgNPs are highly stable and non-toxic showing antimicrobial and anti-cancer activities [Citation5, Citation15, Citation20]. Thermophilic fungi including Thermomyces lanuginosus have also been studied for the synthesis of gold [Citation16] and iron nanoparticles [Citation18]. But, this fungus has not been used for extracellular synthesis of AgNPs. Therefore, we have explored the potential of T. lanuginosus BJMDU1 in green synthesis of silver nanoparticles.
Biogenic AgNPs have been reported in the reduction of a nitro group into an amino group with a shift of absorbance peak from 405 (p-nitrophenol) to 300 nm (p-aminophenol) [Citation18, Citation21]. The reduction of nitroaromatics to amino aromatics has great potential because an amino group of a compound has been used to synthesize dyes, herbicides, pharmaceuticals, and other compounds. Due to their resistant nature, these substances have been discharged into the environment, where they are causing environmental pollution and human health hazards [Citation18, Citation22]. The research on the anti-microbial activity of nanomaterials has increased significantly due to their potential action against various pathogens [Citation22, Citation23]. Comparable to commercial silver nanoparticles, biogenic nanoparticles have shown stronger antimicrobial and anti-cancerous effects [Citation23, Citation24]. Biogenic AgNPs have shown promising results in controlling the growth of pathogenic fungi and bacteria. Several studies have shown that biogenic AgNPs are highly efficient in showing inhibitory activities against Plasmodium falciparum [Citation25]. However, there is no report showing mycosynthesized AgNPs using T. lanuginosus showing antiplasmodial and antimicrobial activity. Therefore, the present study reports the green synthesis of AgNPs using culture extract of a thermophilic mould T. lanuginosus BJMDU1 followed by their characterization and applicability as an antimicrobial as well as anti-malarial agent. These biogenic AgNPs have catalytically reduced p-nitrophenol into p-aminophenol. This is the first report showing the green synthesis of silver nanoparticles exhibiting antimalarial activity synthesized from culture extract of T. lanuginosus BJMDU1.
2. Materials and methods
2.1. Cultivation of microorganisms
Thermophilic mould, Thermomyces lanuginosus BJMDU1 was grown on YpSs agar slants at 45 °C and preserved as described earlier [Citation26]. Spores were collected in normal saline with 0.1% Tween 80 after growing the mould on YpSs agar at 45°C for 4 days. The mould was grown in YpSs broth inoculating with 2.4 × 107 CFU/mL. Gram-positive [Micrococcus luteus] and Gram-negative [Escherichia coli DH5α] bacterial cultures were also revived forte-nightly in a nutrient agar medium.
2.2. Green mycosynthesis of silver nanoparticles
Initially, the mould was cultivated in YpSs broth (pH 5.5) in shake flasks at 45 °C for 3 days. Whatman No.1 filter paper discs were used to filter fungal biomass and culture extract was centrifuged at 10,000 rpm and 4 °C for 10 min. Culture filtrate was used in green synthesis of AgNPs by following the method described earlier by Ahmed and his group [Citation7]. The changed brown colour of the sample was scanned (300-600 nm) using a UV-Vis spectrophotometer (Shimadzu 1800, Japan).
2.3. Fungal growth and conditions for green synthesis of silver nanoparticles
The mould was cultured in different media (Table ) to select a suitable medium for the fungal growth and collection of culture filtrate. The effect of sucrose medium of different pH (5.0-8.0) was studied on the growth of the thermophilic mould and the culture filtrate was used in the synthesis of AgNPs.
Table 1. Compositions of different media used for the cultivation of thermophilic mould.
The culture extract of thermophilic mould was taken and different reaction conditions were also selected for enhancement of the synthesis of AgNPs. The effects of temperature (35-50 °C), light, reaction time (2-8 h), different amounts of fungal culture extract (25-200 µl), and varied levels of silver nitrate (0.3 mM-2 mM) were also studied on the formation of AgNPs. Control experiments containing only culture filtrate and AgNO3 solution were also tested during each experiment.
2.4. Characteristics of silver nanoparticles
Characterization of biologically synthesized AgNPs was carried out based on UV-Vis spectroscopy, FT-IR [Bruker, Germany], X-ray diffraction, Transmission electron microscopy, and dynamic light scattering (DLS) techniques. The biologically synthesized AgNPs were scanned in the wavelength range of 300-600 nm using a spectrophotometer (Shimadzu-UV 1800). Control samples containing culture filtrate and solution of AgNO3 were also tested.
In the further characterization process, AgNPs were thoroughly washed with sterile distilled water and scanned (375-4000 cm−1) in an FTIR spectrophotometer [Bruker, Germany] to get structural information. The size of mycosynthesized silver nanoparticles was analysed by dynamic light scattering (Spectro-scatter RiNA, FmbH class 3B).
Mycosynthesized NPs for HR-TEM analysis were prepared by drop casting of 10 μL AgNPs onto the grid [Citation23]. Morphological properties of the AgNPs were obtained by HR-TEM (JEOL Japan, JEM-2100 Plus).
2.5. Antimicrobial potential of silver nanoparticles
Mycosynthesized AgNPs (10 mg/mL) were tested for anti-bacterial activity against Gram-positive (Micrococcus luteus) and Gram-negative (Escherichia coli DH5α) bacteria. Anti-bacterial testing was studied by agar well diffusion assay using the Kirby–Bauer test [Citation25] by measuring the zone of inhibition after 24 h.
2.6. Antimalarial and hemolytic activity of mycosynthesized silver nanoparticles
Plasmodium falciparum [chloroquine sensitive 3D7 strain] was cultured and maintained as described earlier by Trager and Jensen [Citation27] and synchronized ring-stage parasite was acquired by 5% sorbitol treatment [Citation28]. Stock solutions of AgNPs and chloroquine (CQ) were prepared in milli-Q water and further diluted with a culture medium. The anti-plasmodial activity was carried out using the SYBR Green I-based fluorescence assay of Bhatia et al. [Citation29]. Sorbitol-synchronized ring-stage parasites were cultured in the absence or presence of AgNPs. CQ was used as a positive control. Fluorescence was measured with excitation and emission wavelength fixed at 485 and 530 nm, respectively in a multi-well plate reader [Fluorescence Reader, PerkinElmer]. Fluorescence counts after subtracting counts of control drug CQ [0.1 M] were plotted to determine IC50 values.
Hemolytic activity of mycosynthesized AgNPs was tested as described earlier by Ashokraja et al. [Citation30]. Briefly, fresh RBCs were suspended in PBS (2% hematocrit) and 100 μl aliquots were taken in wells in a 96–well plate with different concentrations of AgNPs or AgNO3 and incubated at 37°C for 4 h. PBS alone and 0.4% Triton X-100 in PBS [100% hemolysis] were taken as controls. Hemoglobin release as an index of hemolysis was monitored in supernatant by A415. Percent hemolysis is expressed as taking 100% hemolysis by positive control i.e. Triton X-100 treated RBCs.
2.7. Reduction potential of mycosynthesized AgNPs
The reduction of p-nitrophenol was carried out by mycosynthesized AgNPs. Reaction containing 150 μL of p-nitrophenol (2 mM), 1 mL NaBH4 (30 mM), and 50 μL AgNPs was carried out to monitor absorption peak at 301 and 405 nm using a spectrophotometer.
All experiments were performed in triplicate and their average values along with standard errors are presented.
3. Results and discussion
3.1. Green mycosynthesis of silver nanoparticles
Synthesis of AgNPs has been carried out using different physical and chemical methods, but biological synthesis has been favoured due to their eco-friendly and economical nature [Citation5, Citation16, Citation31]. The mechanism of nanoparticles synthesis has been studied in many mesophilic fungal species [Citation32]. The thermophilic mould T. lanuginosus has earlier been studied for the production of gold nanoparticles, hydrolytic enzymes, and biomolecules useful in various biotechnological applications [Citation16, Citation33, Citation34]. However, this fungus has not been studied in detail for green synthesis of AgNPs. Therefore, this study reports the potential of culture-extract of T. lanuginosus BJMDU1 in synthesis of AgNPs. The culture filtrate of the mould reduced silver nitrate into AgNPs as observed by a change in colour of the reaction mixture. The culture filtrate reduces silver nitrate and the colour of the solution turns dark brown (Figure (a)). Mycosynthesis of AgNPs was later confirmed through the UV-Vis spectrum (Figure (b)). However, there was no synthesis observed with fungal biomass in this study. The culture filtrate of mould contains many biomolecules including polysaccharides, amino acids, enzymes/proteins, and vitamins, which are useful in the conversion of Ag+ to Ag0 [Citation33–35]. The colour change from light brown to dark brown was the initial sign of the synthesis of silver nanoparticles (Figure (a)). In the UV-Vis spectrum, a peak at 430 nm confirmed the synthesis of nanomaterials (Figure (b)). The SPR confirms the electron excitation in the conductive band around the silver nanoparticles [Citation34, Citation36]. The absorption band of culture extract-mediated AgNPs appeared at 430 nm within 30 min of reaction as observed in earlier studies also [Citation33, Citation36]. The extracellular synthesis by filamentous fungi is highly preferred due to their economical cultivation at a large scale as well as lack of additional methods for release of cytosolic nanoparticles. The mechanism for synthesis of silver nanoparticles using fungi has not been fully understood, but the enzymes and other metabolites present in the culture extract of these fungi are involved in the reduction of silver ions into AgNPs [Citation12, Citation17, Citation37].
3.2. Medium composition affecting synthesis of silver nanoparticles
Medium components are known to affect the fungal growth and the synthesis of AgNPs, therefore, the selection of a suitable medium is important for microbial growth and metabolite production. In the current study, medium 4 showed the higher synthesis of AgNPs by fungal culture extract as compared to other media (Figure (a)). This is due to the presence of nutrients necessary for the growth and metabolism of thermophilic mould, T. lanuginosus leading to the production of enhanced levels of reducing agents involved in the synthesis of AgNPs [Citation33, Citation38, Citation39]. For negative control, growth medium with 1 mM silver nitrate did not show formation of silver nanoparticles. Medium components are important factors controlling the green synthesis, shape, and size of the silver nanoparticles [Citation37, Citation40]. In the culture medium having both negatively and positively charged ions in the medium interacted with charged groups of the stabilizing agents, which had a positive effect on the synthesis of silver NPs [Citation40]. Furthermore, many of the studies suggested that culture media are known to impact the physicochemical efficiency of AgNPs [Citation40, Citation41]. Variations in media components have significantly affected the properties and formation of silver nanoparticles. Birla et al. [Citation38] found MGYP medium to synthesize AgNPs by Fusarium oxysporum. Saxena et al. [Citation39] reported that potato dextrose medium showed better results than other media. Bhatnagar et al. [Citation42] reported higher AgNPs formation by Talaromyces purpurogenus in the medium containing sucrose, yeast extract, and peptone. This is due to secretion of high amount of protein in the medium by the mould [Citation42].
Figure 2. Optimization of silver NPs synthesis by using 'one variable at a time' approach: (a) UV-Vis spectra of SNPs synthesized by growing fungus on different culture media like sucrose, YPSS, PDB, glucose and control of AgNO3. (b) Effect of medium pH on the silver NPs synthesis by culture filtrate of T. lanuginosus. (c) Effect of reaction temperature on the synthesis of AgNPs. (d) Effect of light and dark conditions on mycosynthesis of AgNPs. (e) Effect of different concentrations of AgNO3 on AgNPs synthesis.
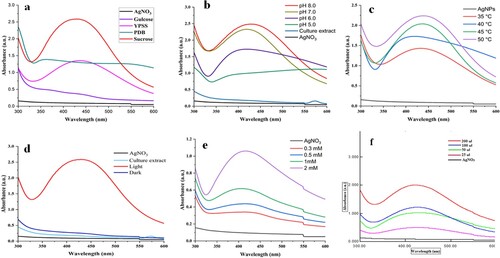
Medium pH has a significant role in the growth and production of fungal metabolites during submerged cultivation. In this study, the fungus grown in a medium of pH 8.0 supported high synthesis of AgNPs using culture extract (Figure (b)). Alkaline pH of the media has resulted in the high synthesis of AgNPs using fungi [Citation43, Citation44]. It has been reported that alkaline conditions increase competition between protons and metal ions for establishing interaction with negatively charged components for high rate of synthesis of AgNPs. However, if enzymes (like reductases) are involved in the synthesis of AgNPs, the effect of pH varies from one fungal culture to another [Citation14, Citation41, Citation43]. Culture extracts of Aspergillus terreus [Citation45] and Penicillium sp. [Citation10] resulted in enhanced synthesis of AgNPs when the fungi were cultivated in the medium of pH 8.0. Syed et al. [Citation20] also reported the enhanced synthesis of gadolinium oxide NPs using culture extract of Humicola sp. grown in the medium of pH 8.0.
3.3 Reaction conditions affecting synthesis of AgNPs
The temperature affects the rate of synthesis of AgNPs as well as their shape and stability. Reduction of Ag + increased with an increase in incubation temperature of the reaction mixture from 30 to 50°C (Figure (c)). High temperature increased the rate of reaction catalyzed by the enzymes/metabolites present in the culture extract of the fungi [Citation12, Citation38, Citation46]. The effect of temperature varies according to fungi used for the synthesis of silver nanoparticles. Similarly, Syed et al. [Citation20] and Khan and Jameel [Citation47] reported the maximum synthesis of nanoparticles at 50°C using culture extract of a thermophilic fungus, Humicola sp. In contrast, silver NPs synthesis by mesophilic fungi i.e. Penicillium sp., Colletotrichum sp. and Trichoderma harzianum has been reported in the range of 25–40°C, [Citation10, Citation14, Citation48]. It is clear from these findings that the effect of temperature varies for biogenic synthesis of AgNPs using fungal extracts, as thermophilic fungi showed maximum synthesis at higher temperatures as compared to mesophilic fungi [Citation10, Citation20, Citation47, Citation48]. This is due to the fact that the enzymes and metabolites produced by thermophilic moulds are more thermostable than mesophiles [Citation18, Citation20]. Some shifting in peaks during optimization of parameters is attributed to the capping of AgNPs by the metabolites present in the fungal culture extract [Citation47, Citation48].
The presence of light was a significant factor in the synthesis of AgNPs by culture extract of T. lanuginosus BJMDU1 (Figure (d)). In the reaction process, no synthesis of AgNPs was observed under dark conditions. This is due to the presence of photons in the light, which speed up the biological reduction of silver ions into AgNPs using fungal metabolites present in the culture filtrate [Citation49]. Rahman et al. [Citation49] have extensively studied the mechanism of light induced synthesis of AgNPs using algal biopolymers and concluded that light plays an important role in reduction of silver salt into AgNPs. Similar observations have been reported using fungi and bacteria for the synthesis of AgNPs in the presence of light [Citation36, Citation44, Citation50]. In contrast, Khan and Jameel [Citation47] reported the synthesis of gadolinium oxide NPs under dark conditions by Humicola sp. after a longer incubation time.
The optimum concentration of silver nitrate is also a critical factor for AgNPs synthesis [Citation12, Citation13, Citation43]. Therefore, an optimal concentration of silver nitrate is required for targeted synthesis of AgNPs. In the present study, silver NPs synthesis increases with an increase in silver nitrate concentration from 0.3 mM to 2 mM (Figure (e)). The concentrations of silver nitrate affect the crystallinity, size, and electrical conductivity of AgNPs. Biogenic AgNPs synthesized using low concentrations of silver nitrate exhibit more electrical conductivity than higher levels. Higher concentrations of silver nitrate are responsible for the synthesis of large-sized nanoparticles with irregular morphology and high crystallinity. Our results are in agreement with other studies on green mycosynthesis of AgNPs [Citation5, Citation13, Citation38, Citation43]. In contrast, Phanjom and Ahmed et al. [Citation12] reported higher concentrations of silver nitrate (8-10 mM) for green synthesis of AgNPs using A. oryzae.
The amount of culture filtrate affects the production of silver nanoparticles significantly due to the presence of metabolites involved in the reduction of silver ions into AgNPs. The present study indicated that with an increase in the volume of culture filtrate, silver NPs synthesis also increases (Figure (f)). This is because of an increase in the amount of reducing agents (enzymes/metabolites) present in the culture extract, which are involved in the synthesis of AgNPs [Citation48]. Similarly, other studies have found an increase in AgNPs synthesis with an increase in the volume of culture extract [Citation13, Citation14, Citation45, Citation50].
3.4. Characterization of mycosynthesized silver nanoparticles
In the UV-Vis spectrum, a band at 430 nm confirmed the synthesis of AgNPs (Figure (b)). The SPR confirms the electron excitation in the conductive band for silver nanoparticles [Citation20, Citation23, Citation39]. The absorption band of culture extract-mediated AgNPs appeared at 430 nm within 30 min of reaction as observed in earlier studies also. Green synthesized silver nanoparticles have been reported to display absorption bands in the range of 400–450 nm [Citation34, Citation36]. FTIR analysis of green synthesized AgNPs and fungal culture extract is presented in Figure (a). FTIR analysis provides information about the involvement of functional groups i.e. carboxyl groups (-C = O), and amine groups (−NH) in AgNPs formation at 3425 and 3281cm−1. In the FTIR spectrum, bands at 3281, 2130, 1992, 1975, 1636, 607, and 583 cm−1 confirmed the role of different amino acids in the green synthesis of AgNPs (Figure (a)). The bands at 2959, 2923, and 2851 cm−1 recognized symmetric and asymmetric stretching of carbohydrates and fatty acids, whereas peaks at 2360 and 2340 cm−1 corresponds to O = C = O stretching vibrations. The peaks at 1653, 1540, and 1077 cm−1 are attributed to the C = O, C = C-C, and C–O or C–O–C stretching vibrations, respectively [Citation50, Citation51]. The peak for the binding of the C–S–H groups was assigned at 668 cm−1. The banding pattern in fungal culture extract and AgNPs are significantly different from each other. The above-listed bands frequency confirmed the functional structure of proteins and amino acids as the capping agents of the AgNPs. Thus, the reducing, stabilizing, and capping agents of the proteins help in the synthesis of AgNPs [Citation49, Citation52]. Similar findings have been observed for the mycosynthesized AgNPs from different filamentous fungi [Citation35, Citation36, Citation51].
Figure 3. Structural characterization of AgNPs. (a) FT-IR spectrum of (i) culture extract of T. lanuginosus and (ii) mycosynthesized AgNPs. (b) X-ray diffraction pattern of mycosynthesized silver nanoparticles from culture filtrate of T. lanuginosus. (c) DLS data showing size analysis of silver nanoparticles. (d) transmission electron microscopic image of AgNPs.
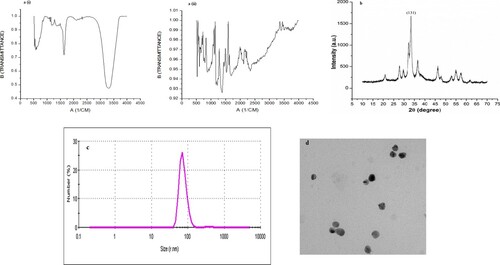
The X-ray diffraction method clearly describes the crystalline nature of AgNPs. More than six bands were observed with a main band at 131 representing silver NPs (Figure (b)). Similar and equivalent diffraction peaks of AgNPs have been reported in other studies on mycosynthesis of AgNPs [Citation53]. Further, the average mean size of silver nanoparticles was confirmed through DLS as 80 nm (Figure (c)). Analysis using Transmission electron microscopy revealed the size of AgNPs about 80 nm with spherical or oval shape (Figure (d)). It has been reported that polymorphic nanomaterials synthesized using biological processes are highly biocompatible in many applications with size ranging from 10 to 1000 nm [Citation54]. These nanoparticles have major advantages as drug delivery systems due to high biocompatibility, bioavailability, improved efficacy and enhanced solubility of therapeutics [Citation54].
3.5. Anti-bacterial potential of AgNPs
Mycosynthesized AgNPs inhibited the growth of both Gram-positive and Gram-negative bacterial cultures (Figure ). However, growth inhibition was observed higher in Gram -ve bacteria (Escherichia coli DH5α) than in Gram-positive bacteria (Micrococcus luteus). This could be due to the differences in their cell wall composition [Citation12, Citation51, Citation55, Citation56]. The cell walls of Gram-negative bacteria have a loosely attached lipopolysaccharide layer, whereas the cell wall of Gram-negative bacteria possesses a thick layer of peptidoglycan through which silver ions cannot penetrate easily. Thus, silver NPs are more toxic to E. coli than Micrococcus luteus. Silver NPs have a large surface area to interact with microbial cells and cause cell rupture that disturbs cell functionality [Citation12]. Silver nanoparticles have the ability for deeper penetration of microbial cell barriers and also damage cytosolic enzymes, proteins, and nucleic acids [Citation1]. These effects are responsible for low cell division and high cell death. Silver nanoparticles also interact with thiol groups of proteins and enzymes and form complexes with nucleotides. Mycosynthesized AgNPs have been reported to inhibit the growth of Gram-positive and negative bacteria including Pseudomonas aeruginosa, E. coli, Staphylococcus aureus, and Bacillus cereus [Citation39, Citation57, Citation58]. The silver nanoparticles synthesized by Aspergillus clavatus inhibited the growth of bacteria (E. coli, Pseudomonas fluorescens) and yeast (Candida albicans) [Citation53].
3.6. Anti-malarial potential of silver NPs
3.6.1 Anti-malarial activity of green nanoparticles
The anti-plasmodial potential of green synthesized AgNPs was determined based on the inhibition of growth of P. falciparum in culture. Biogenic AgNPs showed very good anti-plasmodial activity with an IC50 value of 300 ng/mL, which is further evident from Giemsa stained micrographs (Figure (a)–(b)). AgNPs at a concentration of 625 ng/mL inhibited the growth of plasmodium at the ring stage whereas 312 ng/mL allowed it to grow up to the trophozoite stage showing growth inhibition of plasmodium in a dose-dependent manner (Figure (a)). Whereas enriched fungal culture [1: 25-time dilution] showed no activity. AgNO3 was also found to have anti-plasmodial activity (IC50 600 ng/mL). Further, fungal culture-induced nanoparticles were also found equipotent to CQ resistant strain PfINDO with a selectivity index of 0.9. Panneerselvam and Arumugam, [Citation59] synthesized silver nanoparticles using Andrographis paniculata and reported an 83 ± 0.5% reduction in parasitemia at 100 µg/mL. Jamil et al. [Citation60] synthesized silver nanoparticles using leaves of Catharanthus roseus and showed good anti-plasmodial activity against P. falciparum. Mishra et al. [Citation61] synthesized silver nanoparticles using amylase, Ashoka, and neem extract and reported that anti-plasmodial activities of nanoparticles depend on the scaffold provided for nanotization. Karthik et al. [Citation62] reported that gold nanoparticles synthesized using Streptomyces sp., delayed the rise in parasitemia of Plasmodium berghei in mice. Rajakumar et al. [Citation63] synthesized palladium nanoparticles using Eclipta prostrata extract and reported the inhibition of Plasmodium bergheim in Swiss albino mice.
Figure 5. Silver NPs are potent non hemolytic inhibitors of plasmodial growth: (a) hemolytic profiles of green AgNPs and silver nitrate. (b) In-vitro plasmodial (Pf3D7) growth inhibition profiles of green silver nanoparticles and silver nitrates (c) micrographs of untreated (PRBC), chloroquine (200nM) and green silver nanoparticles treated culture (156 ng/ml, 312 ng/ml and 625 ng/ml).
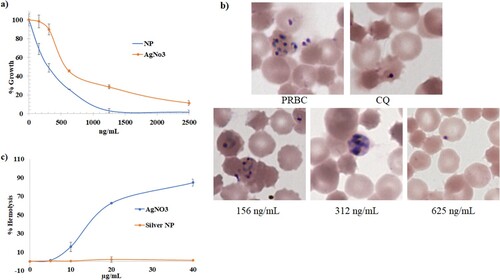
3.6.2 Hemocompatibility properties of green nanoparticles
With the intrinsic anti-plasmodial activity ionic silver (AgNO3) was also reported to show hemolytic activity [Citation61]. To find the advantage of AgNPs over ionic silver i.e. hemocompatibility, we studied the hemolytic behaviour of AgNPs. Fungal culture-induced silver nanoparticles showed no sign of hemolysis up to 40 μg/mL as compared to ionic silver which showed high hemolysis with hemolytic concentration in the range of 16–50 μg/mL (Figure (c)). This suggests that nanotization on green scaffolds reduces the hemolytic property of silver [Citation23].
3.7. Catalytic activity of AgNPs
To assess the reduction activity of green AgNPs, p-nitrophenol was treated with AgNPs and NaBH4. It was observed that p-nitrophenol was successfully converted into p-aminophenol (Figure ). A continuous decrease in absorbance at 430 nm and an increase in absorbance at 301 nm was observed in the reaction mixture. This showed the reduction in p-nitrophenol and its conversion to p-aminophenol in the reaction mixture in a time-dependent manner [Citation18, Citation23]. Catalytic reduction of p-nitrophenol has also been reported using biogenic gold, platinum, and iron nanoparticles [Citation18, Citation64]. This property of mycosynthesized AgNPs could be helpful in bioremediation of environmental pollutants released from various industries.
4. Conclusion
A biological process for green mycosynthesis of AgNPs has been developed first time using culture extract of a thermophilic mould T. lanuginosus BJMDU1. Mycosynthesized AgNPs showed anti-bacterial and anti-malarial activity. Furthermore, mycosynthesized AgNPs showed a catalytic reduction of p-nitrophenol to p-aminophenol. Therefore, mycosynthesized AgNPs using T. lanuginosus could be used as a therapeutic agent as well in the mitigation of environmental pollution.
Acknowledgments
The authors thank M. D. University, Rohtak for providing research facilities for completing this work. We are also grateful to Dr. Rajvinder Singh, Department of Genetics, M. D. University, Rohtak for providing the FT-IR facility and Dr. Anil Ohlan, Department of Physics, M. D. University, Rohtak for providing technical help in the XRD facility.
Disclosure statement
No potential conflict of interest was reported by the author(s).
Additional information
Funding
References
- Kumar V, Kaushik NK, Tiwari SK, et al. Green synthesis of iron nanoparticles: sources and multifarious biotechnological applications. Int J Biol Macromol. 2023;253(4):127017. doi:10.1016/j.ijbiomac.2023.127017
- Ranjha MMAN, Shafique B, Rehman A, et al. Biocompatible nanomaterials in food science, technology, and nutrient drug delivery: recent developments and applications. Front Nutr. 2022;8.
- Banti CN, Hadjikakou SK. Antimicrobial materials with medical applications. Int J Mol Sci. 2022;23:1890. doi:10.3390/ijms23031890
- Horsfall L. Biological synthesis of metallic nanoparticles by bacteria, fungi and plants. J Nanomed Nanotechnol. 2014;5. doi:10.4172/2157-7439.1000233
- Guilger-Casagrande M, Lima RD. Synthesis of silver nanoparticles mediated by fungi: a review. Front Bioeng Biotechnol. 2019;7:287. doi:10.3389/fbioe.2019.00287
- Manimaran M, Kannabiran K. Actinomycetes-mediated biogenic synthesis of metal and metal oxide nanoparticles: progress and challenges. Lett Appl Microbiol. 2017;64:401–408. doi:10.1111/lam.12730
- Ahmed S, Ahmad M, Swami BL, et al. A review on plants extract mediated synthesis of silver nanoparticles for antimicrobial applications: A green expertise. J Adv Res. 2016;7:17–28. doi:10.1016/j.jare.2015.02.007
- S. Shahriary, F. Tafvizi, P. Khodarahmi, etal, Phyto-mediated synthesis of CuO nanoparticles using aqueous leaf extract of Artemisia deserti and their anticancer effects on A2780-CP cisplatin-resistant ovarian cancer cells, Biomass Conv. Bioref. 2022;14:2263–2279. doi:10.1007/s13399-022-02436-x.
- Adebayo EA, Azeez MA, Alao MB, et al. Fungi as veritable tool in current advances in nanobiotechnology. Heliyon. 2021;7:e08480. doi:10.1016/j.heliyon.2021.e08480
- Yassin, M. A., Elgorban, A. M., El-Samawaty, A. E. R. M., & Almunqedhi, B. M. (2021). Biosynthesis of silver nanoparticles using Penicillium verrucosum and analysis of their antifungal activity. Saudi J Biol Sci. 28(4): 2123–2127. doi:10.1016/j.sjbs.2021.01.063
- Deniz F, Mazmanci MA. The biosynthesis of silver nanoparticles with fungal cytoplasmic fluid obtained from Phanerochaete chrysosporium ME446. Environmental Research and Technology. 2020;3:187–192. doi:10.35208/ert.788891
- Phanjom P, Ahmed G. Effect of different physicochemical conditions on the synthesis of silver nanoparticles using fungal cell filtrate of aspergillus oryzae (MTCC No. 1846) and their antibacterial effect. Adv. Nat. Sci: Nanosci. Nanotechnol. 2017;8 :045016. doi:10.1088/2043-6254/aa92bc
- Shahzad A, Saeed H, Iqtedar M, et al. Size-Controlled production of silver nanoparticles by aspergillus fumigatus BTCB10: likely antibacterial and cytotoxic effects. J Nanomater. 2019: e5168698. doi:10.1155/2019/5168698
- Guilger-Casagrande M, Germano-Costa T, Pasquoto-Stigliani T, et al. Biosynthesis of silver nanoparticles employing Trichoderma harzianum with enzymatic stimulation for the control of Sclerotinia sclerotiorum. Sci Rep. 2019;9:14351. doi:10.1038/s41598-019-50871-0
- Rangdist S, Pakdeesiriwong N, Chumkiew S, et al. Green synthesis and characterization of silver nanoparticles from filamentous fungi isolated from the environment and their anti-microbial activity against healthcare-associated bacterial pathogens; 2020.
- Molnár Z, Bódai V, Szakacs G, et al. Green synthesis of gold nanoparticles by thermophilic filamentous fungi. Sci Rep. 2018;8:3943. doi:10.1038/s41598-018-22112-3
- L.P.C. Silva, J.P. Oliveira, W.J. Keijok, A.R. da Silva, A.R. Aguiar, M.C.C. Guimarães, C.M. Ferraz, J.V. Araújo, F.L. Tobias, F.R. Braga, Extracellular biosynthesis of silver nanoparticles using the cell-free filtrate of nematophagous fungus Duddingtonia flagrans. 12: 2017; 6373–6381
- Kumar V, Singh D, Singh B. Mycogenic synthesis of iron nanoparticles using thermophilic mould Myceliophthora thermophila and their applicability in environmental remediation. Biocatalysis and Agricultural Biotechnology. 2024;56:103020. doi:10.1016/j.bcab.2024.103020
- Velhal SG, Kulkarni SD, Latpate RV. Fungal mediated silver nanoparticle synthesis using robust experimental design and its application in cotton fabric. Int Nano Lett. 2016;6(4):257–264. doi:10.1007/s40089-016-0192-9
- Syed A, Saraswati S, Kundu GC, et al. Biological synthesis of silver nanoparticles using the fungus Humicola sp. and evaluation of their cytoxicity using normal and cancer cell lines. Spectrochim Acta A Mol BiomolSpectrosc. 2013;114:144–147. doi:10.1016/j.saa.2013.05.030
- Rodriguez Mejía Y, Bogireddy NKR. Reduction of 4-nitrophenol using green-fabricated metal nanoparticles. RSC Adv. 2022;12:18661–18675. doi:10.1039/D2RA02663E
- Kutyła M, Trytek M, Buczek K, et al. Biomass of a psychrophilic fungus as a biocatalyst for efficient direct esterification of citronellol. Bioenergy Res. 2022;15(1):399–411. doi:10.1007/s12155-021-10289-x
- Bhatt CS, Rajavel A, Parimi DS, et al. Sustainable exscalar monodispersed gold nanoparticles with enhanced dispersion stability and biocompatibility for theragnostics. ACS Appl. Nano Mater. 2023;6:12548–12559. doi:10.1021/acsanm.3c02407
- Mousavi B, Tafvizi F, ZakerBostanabad S. Green synthesis of silver nanoparticles using Artemisia turcomanica leaf extract and the study of anti-cancer effect and apoptosis induction on gastric cancer cell line (AGS). Artif Cells Nanomed Biotechnol. 2018;46:499–510. doi:10.1080/21691401.2018.1430697
- Avitabile E, Senes N, D’avino C, et al. The potential antimalarial efficacy of hemocompatible silver nanoparticles from Artemisia species against P. falciparum parasite. PLoS One. 2020;15(9):e0238532), doi:10.1371/journal.pone.0238532
- Bala A, Singh B. Cellulolytic and xylanolytic enzymes of thermophiles for the production of renewable biofuels. Renewable Energy. 2019;136:1231–1244. doi:10.1016/j.renene.2018.09.100
- Trager W, Jensen JB. Human malaria parasites in continuous culture. J Parasitol. 2005;91:484–486. doi:10.1645/0022-3395(2005)091[0484:HMPICC]2.0.CO;2
- Aw B, Wm K, Jc S, et al. Antibiotic susceptibility testing by a standardized single disk method. Am J Clin Pathol. 1966;45(4):493–496. https://pubmed.ncbi.nlm.nih.gov/5325707/.
- Bhatia R, Gautam A, Gautam SK, et al. Assessment of SYBR green I dye-based fluorescence assay for screening antimalarial activity of cationic peptides and DNA intercalating agents. Antimicrob Agents Chemother. 2015;59:2886–2889. doi:10.1128/AAC.03266-14
- Ashokraja C, Sakar M, Balakumar S. A perspective on the hemolytic activity of chemical and green-synthesized silver and silver oxide nanoparticles. Mater Res Express. 2017;4:105406. doi:10.1088/2053-1591/aa90f2
- Khan SA, Gambhir S, Ahmad A. Extracellular biosynthesis of gadolinium oxide (Gd2O3) nanoparticles, their biodistribution and bioconjugation with the chemically modified anticancer drug taxol. Beilstein J. Nanotechnol. 2014;5:249–257. doi:10.3762/bjnano.5.27
- Loshchinina EA, Vetchinkina EP, Kupryashina MA. Diversity of biogenic nanoparticles obtained by the fungi-mediated synthesis: a review. Biomimetics. 2023;8:1), doi:10.3390/biomimetics8010001
- Elgorban AM, Al-Rahmah AN, Sayed SR, et al. Antimicrobial activity and green synthesis of silver nanoparticles using Trichoderma viride. Biotechnology & Biotechnological Equipment. 2016;30:299–304. doi:10.1080/13102818.2015.1133255
- Singh J, Dutta T, Kim KH, et al. ‘Green’synthesis of metals and their oxide nanoparticles: applications for environmental remediation. J Nanobiotechnol. 2018;16(1):1–24. doi:10.1186/s12951-018-0408-4
- Ottoni CA, Simões MF, Fernandes S, et al. Screening of filamentous fungi for antimicrobial silver nanoparticles synthesis. AMB Express. 2017;7:31. doi:10.1186/s13568-017-0332-2
- Neethu S, Midhun SJ, Sunil MA, et al. Efficient visible light induced synthesis of silver nanoparticles by Penicillium polonicum ARA 10 isolated from Chetomorpha antennina and its antibacterial efficacy against Salmonella enteric serovar Typhimurium. J Photochem Photobiol, B. 2018;180:175–185. doi:10.1016/j.jphotobiol.2018.02.005
- Balakumaran MD, Ramachandran R, Balashanmugam P, et al. Mycosynthesis of silver and gold nanoparticles: optimization, characterization and antimicrobial activity against human pathogens. Microbiol Res. 2016;182:8–20. doi:10.1016/j.micres.2015.09.009
- Birla SS, Gaikwad SC, Gade AK, et al. Rapid synthesis of silver nanoparticles from fusarium oxysporum by optimizing physicocultural conditions. Scientific World J. 2013: e796018. doi:10.1155/2013/796018
- Saxena J, Sharma PK, Sharma MM, et al. Process optimization for green synthesis of silver nanoparticles by sclerotinia sclerotiorum MTCC 8785 and evaluation of its antibacterial properties. SpringerPlus. 2016;5:861. doi:10.1186/s40064-016-2558-x
- Vazquez-Muñoz R, Bogdanchikova N, Huerta-Saquero A. Beyond the nanomaterials approach: influence of culture conditions on the stability and antimicrobial activity of silver nanoparticles. ACS Omega. 2020;5:28441–28451. doi:10.1021/acsomega.0c02007
- Barhoum A. (2022). Handbook of Nanocelluloses: Classification, Properties, Fabrication, and Emerging Applications.
- Bhatnagar S, Kobori T, Ganesh D, et al. Biosynthesis of silver nanoparticles mediated by extracellular pigment from Talaromyces purpurogenus and their biomedical applications. Nanomaterials. 2019;9:1042. doi:10.3390/nano9071042
- Singh D, Rathod V, Ninganagouda S, et al. Optimization and characterization of silver nanoparticle by endophytic fungi Penicillium sp. isolated from curcuma longa (turmeric) and application studies against MDR E. coli and S. aureus. Bioinorg Chem Appl. 2014: e408021. doi:10.1155/2014/408021
- Velu M, Lee J-H, Chang W-S, et al. Fabrication, optimization, and characterization of noble silver nanoparticles from sugarcane leaf (Saccharum officinarum) extract for antifungal application. 3 Biotech. 2017;7 :147. doi:10.1007/s13205-017-0749-y
- Omran BA, Nassar HN, Fatthallah NA, et al. Characterization and antimicrobial activity of silver nanoparticles mycosynthesized by aspergillus brasiliensis. J Appl Microbiol. 2018;125:370–382. doi:10.1111/jam.13776
- Sharma NK, Vishwakarma J, Rai S, et al. Green route synthesis and characterization techniques of silver nanoparticles and their biological adeptness. ACS Omega. 2022;7(31):27004–27020. doi:10.1021/acsomega.2c01400
- Khan NT, Jameel J. Optimization of reaction parameters for silver nanoparticles synthesis from fusarium oxysporum and determination of silver nanoparticles concentration. J Material Sci Eng. 2016;5; doi:10.4172/2169-0022.1000283
- Chandankere R, Chelliah J, Subban K, … Qi X. Pleiotropic functions and biological potentials of silver nanoparticles synthesized by an endophytic fungus. Front Bioeng Biotechnol. 2020;8:95. doi:10.3389/fbioe.2020.00095
- Rahman A, Kumar S, Bafana A, et al. A mechanistic view of the light-induced synthesis of silver nanoparticles using extracellular polymeric substances of Chlamydomonas reinhardtii. Molecules. 2019;24(19):3506. doi:10.3390/molecules24193506
- Zomorodian K, Pourshahid S, Sadatsharifi A, et al. Biosynthesis and characterization of silver nanoparticles by aspergillus species. BioMed Res Int. 2016: e5435397. doi:10.1155/2016/5435397
- Singh P, Mijakovic I. Strong antimicrobial activity of silver nanoparticles obtained by the green synthesis in viridibacillus sp. extracts. Front Microbiol. 2022;13:820048. doi:10.3389/fmicb.2022.820048
- Rahimivand M, Tafvizi F, Noorbazargan H. Synthesis and characterization of alginate nanocarrier encapsulating artemisia ciniformis extract and evaluation of the cytotoxicity and apoptosis induction in AGS cell line. Int J Biol Macromol. 2020;158:338–357. doi:10.1016/j.ijbiomac.2020.05.006
- Verma VC, Kharwar RN, Gange AC. Biosynthesis of antimicrobial silver nanoparticles by the endophytic fungus aspergillus clavatus. Nanomedicine. 2010;5:33–40. doi:10.2217/nnm.09.77
- Paulkumar K, Gnanajobitha G, Vanaja M, et al. Green synthesis of silver nanoparticle and silver-based chitosan bionanocomposite using stem extract of Saccharum officinarum and assessment of its antibacterial activity. Adv. Nat. Sci: Nanosci. Nanotechnol. 2017;8:035019. doi:10.1088/2043-6254/aa7232
- Sharma A, Sagar A, Rana J, et al. Green synthesis of silver nanoparticles and its antibacterial activity using fungus Talaromyces purpureogenus isolated from Taxus baccata Linn. Micro and Nano Systems Letters. 2022;10(1):2), doi:10.1186/s40486-022-00144-9
- Dastidar MG, Nallal V, Razia M. (2021). Synergistic antibacterial activity of mycosynthesized AgNPs with antibiotics against multidrug resistant Pseudomonas aeruginosa and investigation of protein profile.
- Arshad F, Naikoo GA, Hassan IU, et al. Bioinspired and green synthesis of silver nanoparticles for medical applications: A green perspective. Appl Biochem Biotechnol. 2023: 1–34.
- Gudikandula K, Vadapally P, Singara Charya MA. Biogenic synthesis of silver nanoparticles from white rot fungi: their characterization and antibacterial studies. OpenNano. 2017;2:64–78. doi:10.1016/j.onano.2017.07.002
- Panneerselvam S, Arumugam G. A biochemical study on the gastroprotective effect of hydroalcoholic extract of andrographis paniculata in rats. Indian J Pharmacol. 2011;43:402–408. doi:10.4103/0253-7613.83110
- Jamil K, Khattak SH, Farrukh A, … Ali GM. Biogenic synthesis of silver nanoparticles using Catharanthus Roseus and its cytotoxicity effect on vero cell lines. Molecules. 2022;27(19):6191. doi:10.3390/molecules27196191
- Mishra A, Kaushik NK, Sardar M, et al. Evaluation of anti-plasmodial activity of green synthesized silver nanoparticles. Colloids Surf B Biointerfaces. 2013;111:713–718. doi:10.1016/j.colsurfb.2013.06.036
- Karthik L, Kumar G, Rao KVB. Antioxidant activity of newly discovered lineage of marine actinobacteria. Asian Pac J Trop Med. 2013;6:325–332. doi:10.1016/S1995-7645(13)60065-6
- Rajakumar G, Rahuman AA, Chung I-M, et al. Antiplasmodial activity of eco-friendly synthesized palladium nanoparticles using Ecliptaprostrata extract against Plasmodium berghei in Swiss albino mice. Parasitol Res. 2015;114:1397–1406. doi:10.1007/s00436-015-4318-1
- Mejía YR, Bogireddy NKR. Reduction of 4-nitrophenol using green-fabricated metal nanoparticles. RSC Adv. 2022;12(29):18661–18675. doi:10.1039/D2RA02663E
- Maria BS, Devadiga A, Kodialbail VS, et al. Synthesis of silver nanoparticles using medicinal zizyphus xylopyrus bark extract. Appl Nanosci. 2015;5:755–762. doi:10.1007/s13204-014-0372-8