ABSTRACT
Introduction
During past years, lipid nanoparticles (LNPs) have emerged as promising carriers for RNA delivery, with several clinical trials focusing on both infectious diseases and cancer. More recently, the success of messenger RNA (mRNA) vaccines for the treatment of severe diseases, such as acute respiratory syndrome coronavirus 2 (SARS-CoV-2), is partially justified by the development of LNPs encapsulating mRNA for efficient cytosolic delivery.
Areas covered
This review examines the production and formulation of LNPs by using microfluidic devices, the status of mRNA-loaded LNPs therapeutics and explores spray drying process, as a promising dehydration process to enhance LNP stability and provide alternative administration routes.
Expert opinion
Microfluidic techniques for preparation of LNPs based on organic solvent injection method promotes the generation of stable, uniform, and monodispersed nanoparticles enabling higher encapsulation efficiency. In particular, the application of microfluidics for the fabrication of mRNA-loaded LNPs is based on rapid mixing of small volumes of ethanol solution containing lipids and aqueous solution containing mRNA. Control of operating parameters and formulation has enabled the optimization of nanoparticle physicochemical characteristics and encapsulation efficiency.
1. Introduction
Nucleic acid (NA) therapy is founded on the use of nucleic-acid-based materials as drugs for disease intervention at the genetic level. Gene therapy includes gene inhibition, editing, repair, or gene replacement therapy (gene augmentation) [Citation1]. This large family of compounds have different molecular weight, geometry, and composition [Citation2]. NA-based therapies have several advantages over other traditional methods, including tissue specific targeting [Citation3]. However, their anionic structure and high molecular weight do not favor cell penetration. In addition, their degradation in physiological fluids by enzymes results in rapid renal clearance and short-time circulation in blood. Other challenges are related with poor cellular uptake and inefficient endosomal escape [Citation4]. To avoid this instability in biological environment, NAs can be encapsulated in a suitable carrier for efficient intracellular delivery. There are two categories of NA drugs according to their structures: DNA drugs and RNA drugs [Citation5]. Subcategories include catalytic oligos (DNAzyme, RNAzyme), antisense oligonucleotides (ASOs), aptamers (DNA aptamers, RNA aptamers), interference RNA (RNAi) (short interfering RNA (siRNA), micro RNA (miRNA), short-hairpin RNA (shRNA)), messenger RNA (mRNA), interference DNA (DNAi) and DNA (bacterial DNA, plasmid DNA (pDNA)). The first two subcategories of NA-based therapies can be delivered with either carrier or carrier-free, whereas for the others there is the need for a delivery carrier [Citation2]. The first therapeutic NA, a DNA ASO, was approved for clinical use in 1998 by the Food and Drug Administration (FDA), USA. This drug, fomivirsen (Vitravene), was developed for immunocompromised patients suffering with cytomegalovirus retinitis. Only 20 years later, the first RNA-based (siRNA) therapy, patisiran (Onpattro), was FDA approved, followed by givosiran in 2019, for the treatment of the polyneuropathy and acute hepatic porphyria, respectively [Citation6]. Currently, there are over 15 nucleic acid-based therapeutics approved by FDA and European Medicines Agency (EMA) [Citation7].
NA-based vaccines include viral vectors, plasmid DNA and mRNA [Citation8,Citation9]. These therapeutics promote vaccine development against a wide range of pathogens, as they support the delivery of any antigen of choice, regardless of whether it is derived from bacteria, parasite, or virus, and their immune responses are focused only on the antigens of interest [Citation9]. The mRNA vaccines have shown significant interest for the treatment against infectious diseases and several types of cancer. The use of mRNA-based vaccines has several benefits over conventional live attenuated and DNA-based vaccines. Because live attenuated vaccines show higher potency, there is an associated risk of reverting to a pathogenic form and cause infection. In comparison to DNA-based vaccines, mRNA therapeutics are easier to deliver as RNA only needs to be delivered into the cytoplasm of the host cell to be translated into protein. Also, mRNA therapeutics are safer since RNA cannot integrate his genome in the host cell. RNA exhibits shorter half-life, hence only a low level of expression can be achieved in vivo, resulting in a more controllable therapy in case of adverse effects. On top of all the above, mRNA vaccines allow rapid and easy development. Indeed, production of mRNA by a cell-free environment by in vitro transcription of a DNA template that contains the mRNA sequence avoids the use of microorganisms or cultured cells, allowing simple downstream purification and very rapid and cost-effective manufacturing [Citation10].
Particularly, in response to the recent SARS-CoV-2 pandemic, just only 10 weeks after the availability of genetic sequence was it possible to administer the vaccine to the first volunteer in a phase 1 clinical trial [Citation11].
However, until recently, no clinical approval of mRNA vaccines took place due to its instability and inefficient intracellular delivery. Naked mRNA is highly unstable under physiological conditions on account of the rapid degradation by extracellular ribonucleases. Its negative charge and high molecular weight hinder passive diffusion across the negatively charged cell membrane. To mitigate these setback, viral and non-viral vectors have been studied to enhance mRNA delivery to the target site, having present that risks of carcinogenesis [Citation12], immunogenicity [Citation13] and production difficulties are associated with viral vectors [Citation14].
Lipid nanoparticles (LNPs) started as non-viral vectors used to deliver small-molecule drugs and siRNAs [Citation15]. Currently, LNPs are the most used vectors for in vivo delivery of mRNA molecules, offering high encapsulation and high transfection efficiencies. To be efficacious, these vectors need to be able to (1) efficiently bind and condense mRNA, (2) protect it against enzyme degradation and (3) facilitate cellular uptake and endosomal escape into the cytosol. In the past decades, several efforts have been developed to synthetize and optimize LNPs formulations with focus on clinical utility for RNA delivery. Prior to SARS-CoV-2, mRNA prophylactic vaccines were already used in preclinical and clinical studies for other infectious diseases including zika virus, rabies, chikungunya and H10N8 and H7N9 influenza viruses. But SARS-CoV-2 boosted their interest. mRNA-loaded LNPs vaccines against SARS-CoV-2 are already in the market, developed by Moderna (mRNA-1273) and BioNTech/Pfizer (BNT162b2). Other candidates are in clinical evaluation stage. Examples are CureVac AG (NCT04449276; NCT04652102; NCT04674189; NCT04515147), Arcturus Therapeutics (NCT04480957; NCT04728347; NCT04668339), GlaxoSmithKline (NCT04758962), among others.
Traditional bulk methods for lipid nanoparticles production include lipid film hydration and ethanol injection. These methodologies are usually associated with a poor control over the nanoparticle self-assembly and hence their quality attributes. These limitations, in turn, lead to non-reliable results and processes that cannot be easily scaled-up, hindering the translation rate of these delivery systems from research stages, through clinical applications and, consequently, commercial production [Citation16].
Continuous-flow microfluidic devices revolutionized LNP formulation by allowing fast, scalable, reproducible, and robust production of nanoparticles. Methods like thin-film hydration generate large and heterogeneous nanoparticles with lower mRNA encapsulation efficiency, requiring further size reduction methods, such as extrusion or sonication. In fact, rapid mixing is crucial to achieve small-sized LNPs. As example, RNA-containing LNPs self-assemble during microfluidic mixing when an aqueous stream containing RNA is rapidly mixed with ethanol containing helper lipids, amino lipids (ionizable or cationic amino lipids) and polyethylene glycol (PEG) lipids [Citation17]. Targeting ligands can also be incorporated onto the LNP surface to facilitate the delivery of RNA molecules, and as needed these vectors can be co-delivered with adjuvants. But vaccines formulated as liquids accelerate their chemical and physical degradation, making it difficult to achieve an adequate shelf life.
Although many factors may contribute to vaccine instability, the most relevant is temperature degradation and for this reason cold-chain systems are normally required to keep liquid vaccines frozen or refrigerated, limiting their widespread use. The stability of vaccines can be improved through dehydration, preventing degradation pathways such as hydrolysis or oxidation, hence increasing thermostability and extended shelf life. Although lyophilization is the commonly used dehydration method for vaccines, spray drying can be a viable alternative, without vacuum or freezing involved in the process. Nevertheless, spray drying application for mRNA vaccines is relatively unexplored.
This paper outlines the use of lipid-based nanoparticles as non-viral carriers and their structure/composition. It further explores the effects of formulation and microfluidic operating parameters on the critical quality attributes of LNPs and the status of the use of microfluidics to support mRNA-loaded LNP applications (i.e. infectious diseases, cancer immunotherapy and protein replacement therapies). Finally, spray drying is surveyed for its potential as dehydration process to enhance the stability of mRNA-loaded LNPs.
2. Lipid-based nanocarriers
2.1. Lipid nanoparticles as a non-viral vector
The advantages of nanocarriers-based therapeutic mRNA delivery systems include mRNA protection from premature degradation and interaction with the biological environment, improvement of intracellular penetration and retention time. These nanocarriers can be viral or non-viral [Citation18]. Currently, viral carriers are the most efficient means to deliver NAs into cells. However, risks of immunogenicity, unwanted mutagenesis and cancer are still huge limitations of viral carriers [Citation19]. Because non-viral carriers show reduced pathogenicity, they are attractive candidates to be developed based on nanosized lipid or polymeric carriers [Citation20]. Lipid-based nanocarriers, often the preferred choice, naturally interact with the cell membrane, mainly composed by lipids [Citation21]. In addition, when comparing lipids with polymers, the risk of undesirable immunogenic responses to lipids is relatively lower, as polymers have normally higher molecular weights. Lipid-based nanocarriers include liposomes and lipid nanoparticles (). Currently, these systems represent more than half of FDA-approved nanocarriers for clinical use [Citation22–25].
Liposomes can be defined as closed assemblies of bilayers of lipid molecules with polar headgroups and hydrophobic tails (). When liposomes are dispersed in aqueous solutions, their polar headgroups interact with the aqueous environment due to the hydrogen bonds and polar interactions, whereas their hydrophobic tails interact with each other due to van der Waals forces [Citation26]. Normally, the lipid bilayer encapsulates hydrophobic drugs, while hydrophilic and ionic drug molecules can be entrapped into the aqueous core [Citation27]. These lipid carriers were discovered in the 1960s by A.D. Bangham and were introduced as drug delivery systems in the 1970s [Citation28]. However, physical stability of the dispersion, drug leakage, low activity, and difficulties in upscaling are limitations of these systems to be used in therapeutic applications [Citation29].
Lipid nanoparticles (LNPs) are used since the beginning of 2000s as drug delivery systems and currently are the most popular non-viral carriers used, especially because of their recent application in SARS-CoV-2 vaccines as mRNA carriers. LNPs contain an electron-dense core with different physicochemical properties from liposomes, despite containing excipients that are often seen in those carriers [Citation30]. These vectors can be divided into (1) lipid nanoemulsions (LNE), (2) solid lipid nanoparticles, and (3) nanostructured lipid carriers. Both lipid nanoemulsions and solid LNPs are colloidal nanocarriers with a lipophilic core responsible for the protection of the drug from both environmental and/or physiological degradation. Their primary advantage is the improvement of the bioavailability of drugs with poor water solubility. As LNE face several challenges such as fast diffusion from the liquid core and poor stability, these carriers are not being much used as drug delivery systems, except for parenteral nutrition [Citation31]. Solid LNPs can combine advantages of the traditional colloidal carriers, such as emulsions, liposomes, and polymeric nanoparticles, and at the same time mitigate their major weaknesses. Identical to emulsions and liposomes, they are made of physiological acceptable excipients and like polymeric nanoparticles, they exhibit control flexibility in drug release from the solid core. In addition, solid LNPs show a large surface area, superior cellular uptake, prolonged drug release and low toxicity [Citation32]. Nanostructured lipid carriers are modified solid LNPs, whose lipid core is an amorphous phase composed from solid and liquid lipids. These carriers can overcome some of the downsides of solid LNPs, such as low drug loading [Citation31]. In , the current pipeline of LNPs (program count vs phase) is shown.
2.2. Targeted drug delivery
Targeted drug delivery is one of the leading research trends in nowadays drug development. Drugs can be directed to the target cell by either passive or active methods. Passive targeting relies on changes in size, shape, or surface properties of the particle, which affect its circulation time and tumor accumulation [Citation33]. Active targeting is assisted using both responsive elements as light, magnetism or ultrasound and chemical moieties as peptides, antibodies, antibody fragments, small molecules, and carbohydrates [Citation34,Citation35]. Active targeting can significantly increase the amount of drug delivered compared to both passive targeting and free drug [Citation36]. The chemical moieties added onto the surface of particles enhance selectivity to the target cell, and internalization through receptor-mediated endocytosis or insertion and diffusion through lipid bilayers. Antibodies and their fragments are the most studied targeting ligands in preclinical and clinical trials [Citation37]. Antibodies, or immunoglobulins, are large Y-shaped glycoproteins found in all vertebrate life forms. The functional region of the antibody recognizes specific antigens that are expressed solely, or majorly, on the surface of the target cells. Nowadays, more than 30 different monoclonal antibodies are approved for clinical use [Citation34]. Small molecules such as folate and anisamide are another alternative for targeted drug delivery. Folate represents a popular targeting ligand in cancer therapy, due to the presence of strongly upregulated receptors in a wide range of tumors [Citation38–40]. Folate and its conjugates have seen to specifically target folate receptors of tumor cells through the endocytosis pathway [Citation41]. Anisamide, a benzamide derivative, binds to sigma receptors that are overexpressed in human tumors, such as prostate cancer and melanoma [Citation42]. Due to their small size, ease of production, high stability, and low immunogenicity, various peptides have been considered. Arginylglycylaspartic acid (RGD) is one of the most widely applied peptide ligands. Other ligands used onto the surface of lipid nanoparticles are octreotide, bombesin, and gonadotropin releasing hormone (GnRH) [Citation43–48]. Several carbohydrates such as hyaluronic acid, mannose, and galactose can be alternatives. As example, hyaluronic acid is a natural hydrophilic polysaccharide biocompatible, non-toxic, and non-immunogenic applied majorly to target cancer cells [Citation49–57], while transferrin is a targeting strategy reported with nanostructured lipid carriers [Citation58–60]. Besides targeting ligands mentioned above, cell-penetrating peptides (CPPs) which are positively charged short peptide sequences rich in lysine or arginine have also been used. They can facilitate cell internalization of diverse molecular cargos, from small molecules to nanoparticles. CPPs are typically divided into amphipathic (e.g. transportan, model amphipathic peptide (MAP), Pep-1), cationic (e.g. polyarginine, TAT, penetratin), and hydrophobic (e.g. K-FGF, C105Y) [Citation61]. CPP-coated nanoparticles are one of the promising vectors for RNAi delivery. Tomohiro and colleagues developed LNPs modified with a peptide derived from protamine as CPP for siRNA delivery [Citation62]. Another study is related to the use of oleoyl-octaarginine as CPP, showing potential in cancer therapy [Citation63]. Cell-penetrating homing peptides (CPHPs) are positively charged short peptide sequences that have both cell-penetrating and targeting capabilities [Citation34]. Adjuvants can also be added to LNPs to increase the immune response. However, LNP vectors can also have an adjuvant effect by itself [Citation64]. Currently, adjuvants as monosphosphoryl lipid A and imiquimod are being tested with success [Citation65].
3. Microfluidics for production of lipid-based nanoparticles
3.1. Microfluidic method
There are two main conventional manufacturing methods to produce LNPs: lipid film hydration followed by extrusion, sonication, or high-pressure homogenization and ethanol injection [Citation66]. In lipid film hydration, lipids are dissolved in an organic solvent and transferred to a round-bottom flask. The organic solvent is removed in vacuum, which results in a lipid film on the surface of the flask. Subsequently, the hydration of the film is done with an aqueous solution and large (>100 nm) heterogeneous particles are formed. These have low encapsulation efficiency which can be solved by size-reduction steps, such as extrusion, sonication, or high-pressure homogenization (HPH). Extrusion consists in passing a heterogeneous suspension of particles through multiple filters of a designed pore size, using syringe mini extruders [Citation67]. Reducing particle size by sonication can be achieved using a bath sonicator or a probe sonicator. HPH is often used for size reduction of larger batches by forcing the fluid to pass through a narrow nozzle at high velocity and high pressure (150–200 Mpa) [Citation68]. As for ethanol injection, it is a solvent-based method where lipids are dissolved in ethanol, and the solution is injected through a syringe needle in water under stirring to precipitate the lipid.
Microfluidics has brought the ability to manipulate mixing fluids in microfluidic paths with inner dimensions in the order of micrometers or even smaller [Citation69]. Among the benefits of microfluidics, are controlled manipulation, parallelization, consumption of small quantity of reagents, improved mass, and heat transfer (large surface area), quick system response, better reproducibility, improved portability, lower power consumption, safety, and reduction of cost (materials, reagents, and time) [Citation70]. Microfluidic systems are mainly fabricated in silicon, glass, or cyclic olefin copolymer [Citation71].
In 2008, Karnik et al. reported that using microfluidics instead of bulk methods for producing polymeric nanoparticles leaded to smaller sizes, improved polydispersity, slower release rates, and increased encapsulation efficiency and drug loading [Citation72]. The low throughput of one single microchannel is overcome by operating many microfluidic channels in parallel for commercial-scale production [Citation73]. And channel clogging or fouling, which can occur due to aggregation of components in the flow path, can be mitigated by the design, modification of surface properties with e.g. proteins or polymer, or tunning of imposed forces on the flow [Citation74,Citation75].
According to the source of manipulation forces, microfluidic systems are divided into active and passive. Active microfluidic systems for mixing rely on external forces, such as electric, magnetic, acoustic, thermal, pneumatic or pressure, to precisely manipulate fluids and particles, while passive systems depend on the channel geometry or intrinsic hydrodynamic forces for manipulation [Citation76].
For the microfluidic-based organic method, an ethanol solution containing lipids and an aqueous solution with nucleic acids are introduced in the microfluidic device. In a microfluidic device, LNPs are formed by the following four stages: (1) aggregation of lipid molecules; (2) formation of intermediate disk-like structures; (3) diffusion of intermediate disk-like structures and (4) enclose LNPs. The lipid molecules dissolved in ethanol solution, sometimes recurring to heat, are aggregated by self-assembly due to the increasing polarity of the solution (resulting from the decrease of ethanol concentration) ().
These intermediate disk-like structures are named bilayered phospholipid fragments and are thermodynamically semi-stable and grow to a limiting size.
From here, rapid mixing is required to form small-sized LNPs. The total flow rate (Qtotal) of both aqueous (Q1) and organic (Q2) phases, the flow rate ratio (Qratio) between the two streams and lipid concentration are also significant factors for controlling the final size and distribution of nanoparticles [Citation77]. The flow in microfluidics can be described by dimensionless numbers. The Reynolds number (Re), Péclet number (Pe) and the capillary number (Ca) are among the most important to evaluate mixing. The Reynolds number shows the ratio between inertial and viscous forces and is given by Re = ρUL/, where is the density of the fluid, U is the average velocity of the flow, L is the characteristic length of the flow problem (in microfluidics L is usually considered to be the hydraulic diameter, Dh, of the channels) and is the dynamic viscosity.
The Reynolds number is typically small in microsystems (Re < 2000) due to the decrease of both the velocity of the flow, U, of mm/s and the characteristic length Dh of the order of few micrometers. The flow regime, in this case laminar, is dominated by viscous forces, meaning that the fluid moves in parallel laminas along the flow path. If no heat transfer occurs, density (kg.m−3) and viscosity (kg/m.s) are assumed constant along the microchannel.
In this flow regime, the mixing of both mass and heat is predominantly achieved by diffusion, which can be quite inefficient for the length and timescale of the flow in microchannel systems.
The Péclet number, given by Pe = UL/D, where D is the mass diffusivity, indicates ratio between convective and diffusive transport. A small Pe indicates a diffusively dominated flow distribution (poor mixing), while if Pe >100, diffusive mixing plays a secondary role in the transport in comparison with convection (increasingly more efficient mixing).
The capillary number is calculated using Ca = μU/γ, where γ (kg/s2) is the surface tension at the interface between the two phases. The ratio between the capillary number of both phases differentiates the regime in which droplets are formed: squeezing, dripping, or jetting and consequently the dimensions of the droplets [Citation78].
3.2. Types of microchannels
Microfluidic systems contain microchannels, chambers, reservoirs, etc., which are fabricated on silicon, glass, ceramics, polymers, or metals using dedicated techniques such as lithography, micromilling, embossing or laser ablation, to name a few [Citation79]. The main microchannels system designs for mixing, meeting ethanol-injection needs, are T-junction (), hydrodynamic flow focusing (HFF) (), staggered-herringbone (SHM) (), baffle () and bifurcation () [Citation16].
Figure 4. Common designs of microchannels systems. a) T-junction, b) hydrodynamic flow focusing, c) staggered herringbone, d) baffle and e) bifurcation.
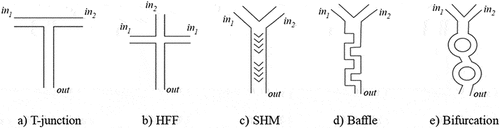
T-junction is one of the most basic embodiments. Two inlets collide at an angle, forming a junction. The interaction of two fluids entering the junction at high momentum promotes chaotic mixing. At low flow rates, the residence time of the fluid is high, fostering higher molecular diffusion. At higher Re, convection becomes more significant caused by the stretching and thinning of liquid laminas.
HFF mixing is the most common technique for continuous production of different types of vectors such polymeric nanoparticles, liposomes, and nanoemulsions. In this approach, the organic phase (containing the lipids) is injected into the central channel with lower flow rate, and the aqueous phase is injected into the two symmetric side channels providing the compression of the central flow. Mixing times are decreased by this compression due to the reduction of the diffusion length. Planar 2D geometries, most common of on-chip devices are less efficient than 3D geometries where the central flow is compressed both horizontally and vertically. Krzysztón et al. produced siRNA-loaded LNPs in a HFF device, showing 20% improvement on encapsulation efficiency for 38 nm LNPs, when compared to bulk methods [Citation80]. However, with HFF, small particles are only generated with Qratio > 30, leading to substantial dilution of the total solution [Citation16].
SHM devices revolutionized nanomedicine by providing higher control of nanoparticle attributes and high throughput. These devices appeared as a variation in basic microfluidic channel, employing a Y-junction followed by physical structures downstream known as staggered herringbone mixers. The repeated folding of the two input streams promotes chaotic advection (2 < Re < 500), significantly reducing the characteristic diffusion length. As such, these devices enable rapid mixing of the two phases with an associated fast decrease of ethanol concentration. Among SHM benefits are the simplicity of the manufacturing process, and the capability to achieve complete mixing of the streams at a low Re.
Baffles and bifurcations are common designs used for mixing in microchannels. Baffles cause the flow to separate, by promoting recirculation and backflow. The mixing efficiency is highly dependent on the baffle height and the Reynolds number in the recirculation zone. Higher baffles and Re promote larger recirculation zones and consequently enhanced convective mixing. Kimura et al. developed an innovative 2D baffle mixer device to overcome clogging in micromixers for LNPs, involving a series of perpendicular turns to rapidly mix the two streams [Citation81]. An example of a toroidal mixer device for LNPs production was successfully able to induce chaotic advection by sequential splitting and mixing of the two streams [Citation82].
3.3. Influence of operational parameters on the critical quality attributes of the LNPs
Various operating and formulation parameters influence the critical quality attributes of the final LNPs. The size of LNPs is one of the essential factors affecting drug delivery efficiency and therapeutic efficiency [Citation77]. Qtotal and Qratio are the most critical parameters mentioned that influence LNP size. Roces et al. reported the influence of different parameters on the final particle size. First, these authors showed that an increase in both Qtotal (5–20 ml/min) and Qratio (1:1–5:1) leads to a decrease in size. The study was made using LNPs with different cationic lipids in their composition (either DDAB, DOTAP or MC3). At a constant Qratio 3:1, the smallest particles were achieved using Qtotal = 20 ml/min and DOTAP in their composition (45–60 nm), followed by MC3-LNPs (50–68 nm) and DDAB-LNPs (75–88 nm). All LNPs showed homogenous size distributions (PdI 0.2). Qratio was evaluated at a constant Qtotal of 20 ml/min. LNPs produced at Qratio 5:1 resulted in smaller and homogeneous particle sizes (PdI 0.2). It is important to note that further examinations were performed at Qratio 3:1 and Qtotal = 20 ml/min. Other influencing formulation parameters have also been reported in literature such as lipid molar ratio or PEG-lipid content. For the evaluation of lipid molar ratio in DOTAP-LNPs, Roces and co-authors fixed the molar composition of PEG-lipid and DSPC at 2% and 10% respectively and varied the molar ratio between cholesterol and the cationic lipid from 10% to 60% and from 78% to 28 % respectively. A decrease in cationic lipid molar ratio resulted in a decrease in particle size and PdI. For MC3-LNPs, the effect of the molar ratio was only noted when 60% or 70% cholesterol was applied. For DDAB-LNPs, the major difference was detected in sizes, ranging from 56 nm to 600 nm, when ranging cholesterol molar ratio from 10% to 60%, respectively. Further, the substitution of structural and coating lipid was evaluated only for DOTAP-LNPs formulation, where HSPC was used as structural lipid and DSPE-PEG2000 as coating lipid. Replacement of both lipids leaded to nanoparticles with similar physicochemical characteristics. In addition, they showed that using TRIS 10 mM pH 7.4 buffer instead of citrate 100 mM pH 6 buffer, the particles formed were smaller, but less homogeneous (PdI ~ 0.4) [Citation66].
LNPs particle size, zeta potential, and PdI are usually characterized using dynamic light scattering (DLS). High encapsulation efficiencies were reported in all cases (>90%). Indeed, microfluidics allows high loadings, normally higher than 70%, as reported in other studies (see ). Belliveau et al. investigated the effect of Qtotal on the size and PdI of the particles using an SHM. Increasing the Qtotal from 0.02 to 4 ml/min leaded to a continuous decrease in the PdI. For the size, it did not change for Qtotal higher than 2 ml/min [Citation83]. Low lipid concentration is required to form small-sized nanoparticles. It was observed by Maeki and colleagues that using 10 mg/ml or 20 mg/ml lipid solution POPC/ethanol, instead of 5 mg/ml leaded to a larger size. These authors also reported the effect of the diluting rate of ethanol on the LNP size by using three types of microfluidic devices, differing from each other by the depths of the chaotic micromixer structures. The results indicated that a rapid dilution of ethanol is essential to formulate small LNPs and for low Qtotal and Qratio. As such, devices with deeper micromixers should be chosen [Citation77]. PEG-lipid content is also an important parameter to control LNPs size. Typically, as PEG-lipid content is increased, progressively smaller LNPs are achieved [Citation83,Citation84]. By varying the PEG-lipid content, SHM devices can be used to produce siRNA-loaded LNPs of different sizes. Chen and co-workers found that hepatic gene silencing was most efficient for particle sizes ranging from 38 to 78 nm, whereas the smallest (27 nm) were unstable and the largest ones (117 nm) were unable to penetrate the fenestrations in the liver vasculature [Citation85].
Table 1. In vivo studies using mRNA-loaded LNPs obtained by microfluidics.
4. In vivo performance of mRNA-loaded lipid nanoparticles formulated by microfluidics
LNPs are versatile complex structures, being able to encapsulate small and large RNA molecules and protect them against enzyme degradation and renal clearance. Since patisiran, many advances were made in the field and currently there are several active clinical trials using RNA-loaded LNPs at different stages with focus on different medical conditions. Besides siRNA therapeutics, multiple mRNA therapeutics are being developed, normally using intramuscular injection as delivery route. Moderna stands out in the development of mRNA-loaded LNP vaccines by having several candidates against the recent SARS-CoV-2 (NCT04470427; NCT04405076; NCT04283461), Zika virus (NCT03014089; NCT04064905), Chikungunya virus (NCT03325075; NCT03829384), influenza (NCT03076385; NCT03345043), cytomegalovirus infection (NCT03382405), and cancer (NCT03323398; NCT03897881; NCT03323398; NCT03739931).
The concept behind RNA-vaccines is to deliver an mRNA encoding the desired protein to the cytoplasm of the target cells, where translation occurs, to stimulate the adaptive immune response (). The main encoded proteins by mRNA vaccines are antigens [Citation98]], neutralizing antibodies [Citation99] and proteins with immunostimulatory activity [Citation100]. The most important targets for mRNA vaccines are antigen-presenting cells (APCs), concentrated at high density in lymph nodes, with dendritic cells being the most relevant cell type. These transfected cells express the mRNA-encoded protein for recognition by certain lymphocytes such as T cells. Currently, there are two major forms of RNA-based vaccines: (1) non-replicating mRNA and (2) self-amplifying mRNA vaccines [Citation8]. The advantage of the first form over the second is that non-replicating mRNA is smaller, simpler construct and lack any extra encoded proteins, which could trigger an unwanted immune response. In fact, self-amplifying mRNA is much larger than non-amplifying mRNA as it additionally encodes viral RNA polymerase for RNA replication leading to a dramatic decrease in the effective dose. mRNA vaccines can be used either for prophylactic vaccination for prevention of future infections or therapeutic vaccination for cancer immunotherapy. Only non-replicating mRNA vaccines are applied for cancer immunotherapies, while for infectious diseases, both forms can be used. Several preclinical trials of RNA-loaded LNPs obtained using microfluidics are reported in the literature ().
EE – Encapsulation efficiency; Chol – Cholesterol; iv–intravenous; sc – subcutaneous; id-intradermal; im-intramuscular; ith-intrathecal injection
Bahl et al. developed non-replicating mRNA vaccines encoding hemagglutinin proteins of H10N8 and H7N9 Influenza viruses [Citation101]. First, in vitro protein expression for both mRNA vaccines was confirmed by transfection in HeLa cells. Regarding animal studies, it evaluated the immunogenicity in mices, ferrets, and nonhuman primates. Mice were vaccinated i.d with 0.4, 2 or 10 ug/animal, ferrets with 50 or 100 ug/animal i.d and NHP with 200 or 400 ug/animal i.d or i.m. For these animal models, both vaccines generated an increased survival rate and a strong hemagglutination inhibition titer compared to control. Also, a phase I H10N8 mRNA vaccination study (NCT identifier: NCT03076385) was conducted in humans, being the first published example of NA-based vaccine against an infectious disease, without using electroporation method, showing promising results [Citation101]. In the same year, Moderna scientists also demonstrate a study against Influenza virus in NHP with non-replicating mRNA vaccines encoding again hemagglutinin proteins of H10N8. NHP were immunized either i.m or i.d, being that i.d. delivery showed more rapid response. In fact, the frequency of APCs in the skin is higher compared to the muscle. For three groups, three different doses were administrated: (1) 50 ug/animal i.m, (2) 50 ug/animal i.d and (3) 5ug/animal i.m, though the LNPs were co-formulated with glucopyranosyl lipid adjuvant (GLA) (with an additional immunization at week 15). Results showed that the use of GLA did not enhance immune responses, thus mRNA-loaded LNPs formulation without it were sufficient. Vaccine immunization induced robust germinal centers and B cell responses [Citation102]. More recently, Moderna developed a new class of ionizable lipids to replace MC3 lipid. The tolerability and immunogenicity of 30 LNPs (each comprising a different ionizable lipid) were screened after their i.m administration in mice (two 0.001 mg/kg mRNA doses with 3 weeks interval). It is reported that the pKa of the ionizable lipid and the LNPs size are determinant factors when it comes to LNP performance. First, the optimal pKa range is between 6.6 and 6.9 for i.m administration and between 6.2 and 6.5 for i.v administration. Second, LNPs showing sizes between 75 and 95 nm were the best performing formulations. The five lead formulations were further evaluated in NHPs [Citation103]. CureVac AG showed that single i.m injections of mRNA-loaded LNPs were sufficient to induce the production of rapid, strong, and long-lasting antibody titers against rabies infection [Citation104]. Pardi et al. demonstrated that a single, low-dose intradermal immunization elicited robust immunity against Zika virus in NHPs and mice. Additionally, the protective efficacy by a single immunization with DNA or inactivated virus vaccines was 50–100 times smaller than with LNP system [Citation105]. Although i.v. administration is less favorable, due its relationship with liver accumulation, some therapeutics have been reported in literature. In 2016, the University of Pennsylvania in collaboration with Acuitas Therapeutics reported that a single injection protected mice against intravenous HIV-1 challenge (30 ug of mRNA per animal) [Citation106]. Recently, Kose et al. developed mRNA-loaded LNPs for hepatic expression of a neutralizing human monoclonal antibody named CHKV-24 against the chikungunya infection. The experiments resulted in high CHKV-24 expression levels in both mice and NHP and, in addition, elicited protection against musculoskeletal tissue infection, arthritis, and reduction of viremia in mice [Citation107].
Apart from infectious diseases, microfluidics is also demanded for the formulation of mRNA vaccines against cancer. This mRNA therapeutics encode cancer-specific antigens to induce a specific immune response by host T cells against tumor cells. In this regard, Oberli et al. reported a treatment for melanoma using LNPs loading mRNA coding for different antigens, ovalbumin, or gp100 and TRP2 [Citation108]. First, the formulation was efficiently taken up by different immune cells in vivo, including dendritic cells, macrophages, neutrophils, and B cells. Further, efficacy was evaluated in two mouse tumor models, transgenic OVA-expressing and B16F10. Mice received sc injections with 10 ug/animal of mRNA. This treatment resulted in decreased tumor growth and prolonged survival of the treated mice. They also show that when TLR4 agonist lipopolysaccharide is added to the formulation as adjuvant, the immune response is increased. Other adjuvants have been reported to enhance mRNA-mediated cancer immunotherapy such as TLR1 and TLR2 agonist lipopeptide tri-palmitoyl-S-glyceryl cysteine (Pam3C) SK4 [Citation109]. The developed Pam3 incorporated LNPs led to an enhanced immune stimulation and a significant delay of tumor growth after two i.m injection of 20 ug mRNA per mouse with 1 week interval. The use of mRNA for the expression of therapeutic proteins has also been reported using microfluidics. The earliest study using mRNA-loaded LNPs for protein replacement therapy was published only in 2016 against Friedreich´s ataxia, a neurodegenerative disease. Mice injected intrathecally into the lower lumbar region (dose of 0.2 mg/kg) showed ~3-fold higher frataxin protein expression in the dorsal root ganglia in comparison to the control group [Citation110]. In 2018, Robinson et al. reported that mRNA-loaded LNPs can also be applied via nasal administration for the treatment of cystic fibrosis. Mice received two consecutive doses (0.1 mg/kg mRNA per day), resulting in the restoration of chloride transport to the nasal airway epithelium which lasted for 2 weeks post-exposure [Citation111]. Translate Bio was the first to own a phase I clinical trial with nebulized LNPs with focus in the treatment of cystic fibrosis (NCT03375047).
5. Drying of RNA and delivery platforms
Stability issues regarding liquid formulations of vaccines can be an obstacle for their industrialization and distribution. Elevated temperatures can be harmful for vaccine stability, and, for this reason, cold-chain systems are normally required to keep vaccines frozen or refrigerated [Citation112]. Chemical instability during mRNA storage includes hydrolysis of N-glycosidic bonds, hydrolysis of phosphodiester bonds, deamination of cytosine derivatives and oxidation of nucleobases or sugar moieties [Citation113]. However, when vaccines are converted to dry powder, greater thermostability and extended shelf life are achieved.
Lyophilization, or freeze-drying, is the most common method for drying vaccines. This batch process consists of three interdependent processes: (1) freezing wherein ice crystals are formed (2) primary drying wherein frozen water is removed through sublimation at low temperatures and (3) secondary drying wherein residual water is removed through desorption. Higher freezing temperatures, slower freezing rates, and longer secondary drying times are beneficial for stability [Citation114]. However, during this complex process stress sources are generated, such freezing and drying stresses [Citation115]. The mechanical stress induced by ice on particles and crystallization of PEG layer, leading to particle fusion, are situations that can occur during freezing [Citation114]. Excipients like cryoprotectants or lyoprotectants are stabilizers added to the particle suspensions immediately prior to lyophilization, being sugars (e.g. trehalose) or sugar alcohols (e.g. mannitol) the most used. Among several theories proposed to explain the stabilization of nanoparticles with cryo- or lyoprotectants, the amorphous glass theory stands out. This theory claims that during the freezing process, cryoprotectants solidify into an amorphous glass around the particles, protecting them from fusion [Citation116]. In 2007, Jones et al. reported that addition of trehalose in a self-amplifying RNA prior to lyophilization kept stability for at least 10 months under refrigerated conditions. After being transfected, high levels of expression were observed [Citation117]. A few years later, the efficacy of mRNA vaccines against infectious disease (Influenza) was first shown in animal models. The lyophilized mRNA influenza vaccine was stable at 37°C for 3 weeks before mice immunization [Citation118]. The same group in a later publication reported that critical exposure of a non-replication mRNA vaccine against rabies infection at 70°C did not compromise the protective capacity [Citation119]. Another mRNA vaccine also against rabies was reported by CureVac to be stable after lyophilization using trehalose and storage at 5–25°C for 3 years and at 40°C for 6 months [Citation120]. More recently, a study was published regarding mRNA-loaded LNPs. Zhao et al. group compared two different approaches for long-term mRNA nanoparticles storage. They observed that though using 20% (w/v) sucrose or trehalose stabilized the nanoparticles size and mRNA delivery efficiency in vitro, the same particles did not show efficiency in vivo delivery. A reason for it could be the change of nanoparticles structure during the lyophilization and reconstitution process. Freezing mRNA-loaded LNPs in liquid nitrogen with the addition of 5% (w/v) sucrose or trehalose can be an interesting alternative for long-term storage [Citation121].
Spray-drying provides an attractive alternative to lyophilization to produce dried vaccines that consume less energy, is less costly to operate, and avoids cell freezing and high vacuum. Spray drying is a continuous drying process which consists of four main phases: (1) atomization of the liquid feed, (2) contact between a hot drying gas and the atomized spray, (3) formation of dry particles, and (4) separation of particles. Another important point is that spray dried vaccines can be administered for non-conventional routes, such as oral, pulmonary, or intranasal routes [Citation122–124]. Despite these advantages, destabilization of the systems during spray drying may occur due to high temperatures and shear forces. Both thermal and shear stresses increase the kinetic energy, leading to particle collision. In addition, partial melting of lipids during the process also leads to particle aggregation, thus it is recommended the use of lipids with melting points >70°C [Citation125]. Indeed, differences in size distribution, PdI ~1 and high coefficients of variation are signals of particle aggregation. Thermal stresses can be minimized by adding a suitable stabilizer or using an alcoholic (instead of aqueous) dispersion medium due to the lower inlet temperatures [Citation126]. On the other hand, shear stresses can be minimized by using a low lipid content or by adding stabilizers. Saccharides are the most used stabilizers, though other excipients have been often added to formulation such as divalent ions, proteins, surfactants, and polymers [Citation127].
In 1998, the first spray drying study of lipid nanoparticles in the pharmaceutical field was conducted [Citation125]. The authors showed a successful conversion of solid LNP suspensions into a powdered form, using very low lipid concentrations (1%) and high trehalose levels (25%) as spray drying matrix. Adding biopolymers onto lipid nanoparticles, such as casein, pectin, or papain, prior to spray drying is another effective strategy to prevent aggregation. For example, Gaspar et al. covered solid LNPs with a papain layer followed by spray drying with trehalose or mannitol [Citation128]. Spray drying of curcumin-loaded solid LNPs covered with a layer of pectin followed by chemical crosslinking was also reported [Citation129]. Indeed, the crosslinking improved the physicochemical properties of the solid LNPs. The same authors applied different natural polysaccharides, such as pectin, carrageenan, carboxymethyl cellulose, gum arabic and alginate, as wall materials but aggregation of particles occurred. On the other hand, spray drying of nanostructured lipid nanoparticles containing 20–30% oleic acid with pectin or carrageenan led to stable powder particles [Citation130]. Polymer hybrid LNPs are also reported in literature. For example, acyclovir-loaded polymer hybrid LNPs were prepared from cross-linking hyaluronic acid with poly(acrylic acid). Compared with the conventional formulation, the solubility of acyclovir could be improved by 30%, enhancing its bioavailability as an oral drug delivery system [Citation131]. More recently, Dormenval et al. prepared spray dried siRNA-loaded polymer hybrid LNPs using mannitol as stabilizing excipient. The group also intends to use microfluidics for further upscaling of the process [Citation132].
To date, there are no commercialized spray dried vaccines. However, several studies have been developed, especially with focus on influenza and tuberculosis. Regarding spray dried mRNA therapeutics, few studies have been reported and to date, none related to spray dried mRNA-loaded LNPs. The first inhalable mRNA delivery was reported by Patel et al. In their study, mRNA was delivered by hyperbranched poly(beta amino esters) (hPBAEs) through nebulization to mice. High levels of gene expression were observed in the lung epithelium of mice [Citation133]. More recently, researchers from University of Hong Kong showed for the first time that inhalable mRNA dry powder can be prepared using spray drying and spray freeze drying. This PEGylated KL4/mRNA complexes resulted in promising gene expression in the lung of healthy mice and did not cause significant toxicity and inflammatory response [Citation134].
6. Conclusions
Based on preclinical and clinical studies, mRNA vaccines using LNPs as nanocarriers have shown promise for the treatment of a wide range of chemical conditions, including infectious diseases and cancer. LNPs offer several benefits over other vectors, including mRNA protection, delivery of higher payload, target ligands incorporation, and co-delivery with adjuvants. Microfluidics was showed to be a versatile approach for LNPs formulation as it allows a continuous nanosized production and offers scale-up options. The impact of several operating and formulation parameters including Qtotal and Qratio that influence physicochemical characteristics of the final particles needs to be considered. Optimization of these parameters on single microfluidic devices can be easily translated to identical multiplexed systems to increase throughput and production. Typically, mRNA vaccine formulations are developed in liquid state and stored frozen. However, efforts to develop thermostable mRNA formulations enhancing their distribution and storage have been gaining interest. Spray drying is a suitable competitor to conventional lyophilization as scientific interest grows in particle engineering and nonconventional routes for vaccine administration. Yet, its application for mRNA vaccines is still unexplored.
7. Expert opinion
Investigation of RNA therapeutics and mechanisms that enable their efficacy is one of the milestones in modern science. The significant developments observed in this field have been fueled by high RNA therapeutic promise in the development of prophylactic vaccines and therapeutic vaccination for cancer treatments. Currently, there are several preclinical and clinical studies dedicated to the evaluation of clinical effectiveness and safety of RNA therapies. Moreover, several innovations in drug delivery methods and RNA engineering have allowed the design of successful therapeutics for diseases that in the past were difficult to target.
To date, viral and non-viral carriers are leveraged for delivering RNA into the cell. Even though viral vectors have shown to be efficient, safety concerns are still challenging to address. Considering these safety issues and the FDA approval process, biodegradable and biocompatible nanoparticle carrier systems for RNA delivery possess great potential. Offering higher safety, non-viral lipid carriers display well-defined characteristics, which render them an attractive and efficient approach for RNA delivery, protecting the latter from enzymatic degradation and increasing transfection across cell membrane. Additionally, lipid carriers allow controlled drug release and can be modified to obtain desired characteristics regarding surface charge and/or hydrophobicity or targeting moieties. Moreover, LNPs may contribute to improve therapeutic efficacy with reduced drug dosage, dosing frequency, and side effects.
Microfluidic is a reliable alternative to produce lipid-based carriers. The rise of several companies dedicated to the commercialization of microfluidics platforms to develop drug formulations with well-defined physicochemical properties confirms the growing interest in this field. This trend is expected to continue, once microfluidics shows potential to become widely adopted for being a reproducible, versatile, economic, and safe approach not to mention with reduced environmental impact. Further, within a single device, a large variety of loaded carriers can be rapidly developed. These nanocarriers have been extensively reported in literature to be uniform in size and to have high encapsulation efficiency while maintaining their efficacy both in vivo and in vitro. However, liquid formulations may lack long-term stability as particles tend to agglomerate and suffer both physical and chemical stresses. Therefore, it is beneficial to explore dry powder formulations to improve shelf life and the supply chain.
In future, the formulation strategy of LNPs may be expanded to the encapsulation of more complex drugs and the functionalization of these carriers with targeting moieties to prevent the non-specific delivery and ensure effectiveness to the target site. Toxicity and immunogenicity risks may also drive further nanoparticle optimization initiatives to mitigate their off-target reactivity in biological systems. Moreover, efforts should be done to deeply understand the interaction between LNPs and their biological environment. The application of microfluidics for drug delivery production is expected to contribute to the creation of novel therapeutics capable of changing the landscape of biopharmaceutical industries. It is expected that the global market for microfluidic technologies reach nearly USD 34.5 billion by 2028 [Citation135]. However, microfluidic technologies still need to be improved to expand production to accelerate the translation of preclinical formulations to the commercial scale. Indeed, typically the daily production rate of nanoparticles by microfluidics is less than 10 mL/h and for clinical and industrial-scale production, a production rate of kilograms or more per day is desirable (>10 L/h). In this regard, it is highly desirable to consider the operation of several scaled-out devices in parallel, replicating the conditions of a single to multiple devices. Nevertheless, scalability remains challenging since it is difficult to ensure the same operating conditions for several similar devices [Citation136]. As an alternative to parallelization, new microdevices are being developed that allow for higher throughput and pressures (e.g. toroidal micromixer) [Citation137]. Some platforms are already available in the market, such as the NanoAssemblr® platforms. Impingement jets mixing technology is also an alternative to microfluidic method for the formulation and production of LNPs at throughputs suitable for in vivo studies, clinical trials, and industrial-scale production. For impingement jet mixing technology, the generation of supersaturation by turbulent micromixing is faster than the diffusion limited aggregation that controls LNPs assembly in microdevices [Citation138–140]. A successful siRNA-loaded nanoparticle production rate up to 3 kg/day was recently reported by developing a versatile coaxial turbulent jet mixer [Citation141]. Furthermore, the BNT162b2 vaccine also benefited from its continuous production with parallelization of 100 static mixers, which have been used to increase vaccine productivity to 100 million doses/month [Citation142]. Furthermore, the ability to administer these delivery systems through alternative routes can greatly advance their clinical relevance. Spray dryer is a suitable approach, not only for allowing the drug delivery to several routes of administration but also to improve API chemical stability during LNPs storage. Nevertheless, understanding the maintenance of relevant LNPs critical quality attributes and RNA integrity during spray drying process should be considered in future.
Article highlights
Lipid nanoparticles exhibit biocompatibility and biodegradability and demonstrate high efficiency in vitro and in vivo.
Microfluidic technologies allow the development of lipid systems with well-defined physicochemical characteristics through finely controlling the mixing of solvent and antisolvent streams.
Microfluidic technologies rely on nanoprecipitation processes in fluidic channels with small inner dimensions.
In comparison with batch processes, microfluidics methods are cost-effective, reproducible and allow the production of stable, uniform, and monodispersed nanoparticles with high encapsulation efficiency.
Spray drying is a suitable approach for drying lipid systems, increasing their chemical and physical stability.
Abbreviations and acronyms
APC | = | Antigen-presenting cell |
ASO | = | Antisense oligonucleotide |
Ca | = | Dimensionless capillary number |
CPHP | = | Cell-penetrating homing peptide |
CPP | = | Cell-penetrating peptide |
CTAB | = | Cetyltrimethylammonium bromide |
D | = | Diffusivity |
DC-Chol | = | 3β-[N-(N’,N’-dimethylaminoethane)carbamoyl] cholesterol |
DDAB | = | Didodecyldimethylammonium bromide |
Dh | = | Hydraulic diameter |
DLinDMA | = | 1,2-dilinoleyloxy-3-dimethylaminopropane |
DOPA | = | Dioleoylphosphatydic acid |
DOPC | = | Dioleoylphosphatidylcholine |
DOPE | = | 1,2-dioleoyl-sn-glycero-3-phosphoethanolamine |
DOTAP | = | 1,2-dioleoyl-3-trimethylammonium propane |
DOTMA | = | N-[1-(2,3-dioleoyloxy)propel]-N,N,N-trimethylammonium |
DSPC | = | 1,2-distearoyl-sn-glycero-3-phosphocholine |
FDA | = | Food and Drug Administration |
GLA | = | Glucopyranosyl lipid adjuvant |
GMO | = | Glycerol monooleate |
GnRH | = | Gonadotropin releasing hormone |
HFF | = | Hydrodynamic flow focusing |
HIV | = | Human immunodeficiency virus |
hPBAEs | = | Hyperbranched poly(beta amino esters) |
HSPC | = | Hydrogenated L-α-phosphatidylcholine |
iDNA | = | Interference DNA |
L | = | Characteristic length |
LNE | = | Lipid nanoemulsion |
LNP | = | Lipid nanoparticle |
MAP | = | Model amphipathic peptide |
MC3 | = | Dilinoleylmethyl-4-dimethylaminobutyrate |
miRNA | = | MicroRNA |
mRNA | = | Messenger RNA |
NA | = | Nucleic acid |
NHP | = | Nonhuman primate |
NLC | = | Nanostructured lipid carrier |
ODN | = | Oligodeoxynucleotide |
PBAE | = | Polyβ‐amino ester |
PdI | = | Polydispersity index |
PDNA | = | Plasmid DNA |
Pe | = | Dimensionless Péclet number |
PEG | = | Polyethylene glycol |
PEG-DMG | = | 1,2-dimyristoyl-sn-glycerol |
PEG-DSG | = | 1,2-distearoyl-sn-glycerol |
PEG-DSPE | = | 1,2-distearoyl-sn-glycero-3-phosphoethanolamine |
PEI | = | Polyethylenimine |
PLA | = | Polylactic acid |
PLGA | = | Poly(lactide-co-glycolide) |
POPC | = | 1-palmitoyl-2-oleoyl-sn-glycero-3-phosphocholine |
Qratio | = | Flow rate ratio |
Qtotal | = | Total flow rate |
Re | = | Dimensionless Reynolds number |
RES | = | Reticuloendothelial system |
RGD | = | Arginylglycylaspartic acid |
RNA | = | Ribonucleic acid |
RNAi | = | RNA interference |
SARS-CoV-2 | = | Severe acute respiratory syndrome coronavirus 2 |
SHM | = | Staggered-herringbone micromixer |
shRNA | = | Short-hairpin RNA |
siRNA | = | Short interfering RNA |
U | = | Characteristic velocity Density Surface tension Dynamic viscosity |
Declaration of interest
The authors have no relevant affiliations or financial involvement with any organization or entity with a financial interest in or financial conflict with the subject matter or materials discussed in the manuscript. This includes employment, consultancies, honoraria, stock ownership or options, expert testimony, grants or patents received or pending, or royalties.
Reviewer disclosures
Peer reviewers on this manuscript have no relevant financial or other relationships to disclose.
Additional information
Funding
References
- Charlie H, Uludaǧ H. Nucleic-acid based gene therapeutics: delivery challenges and modular design of nonviral gene carriers and expression cassettes to overcome intracellular barriers for sustained targeted expression. J Drug Target. 2012;20(4):301–328.
- Dobrovolskaia MA. Nucleic acid nanoparticles at a crossroads of vaccines and immunotherapies. Molecules. 2019;24:4620.
- Viola JR, Rafael DF, Wagner E, et al. Gene therapy for advanced melanoma: selective targeting and therapeutic nucleic acids. J Drug Deliv. 2013;2013:1–15.
- Weng Y, Huang Q, Li C, et al. Improved nucleic acid therapy with advanced nanoscale biotechnology. Mol Ther Nucleic Acids. 2020;19:581–601.
- Sridharan K, Gogtay NJ. Therapeutic nucleic acids: current clinical status. Br J Clin Pharmacol. 2016;82(3):659–672.
- Xiong H, Diermeier SD. Recent advances in oligonucleotide therapeutics in oncology. Int J Mol Sci. 2021;22:3295.
- Gupta A, Andresen JL, Manan RS, et al. Nucleic acid delivery for therapeutic applications. Adv Drug Deliv Rev. 2021;178:113834.
- Geall AJ, Mandl CW, Ulmer JB. RNA: the new revolution in nucleic acid vaccines. Semin Immunol. 2013;25:152–159.
- Maruggi G, Zhang C, Li J, et al. mRNA as a transformative technology for vaccine development to control infectious diseases. Mol Ther. 2019;27:757–772.
- Iavarone C, O’hagan DT, Yu D, et al. Mechanism of action of mRNA-based vaccines. Expert Rev Vaccines. 2017;16(9):871–881.
- Lurie N, Saville M, Hatchett R, et al. Developing covid-19 vaccines at pandemic speed. N Engl J Med. 2020;382(21):1969–1973.
- Baum C, Kustikova O, Modlich U, et al. Mutagenesis and oncogenesis by chromosomal insertion of gene transfer vectors. Hum Gene Ther. 2006;17(3):253–263.
- Bessis N, GarciaCozar FJ, Boissier MC. Immune responses to gene therapy vectors: influence on vector function and effector mechanisms. Gene Ther. 2004;11:10–17.
- Bouard D, Alazard-Dany N, Cosset FL. Viral vectors: from virology to transgene expression. Br J Pharmacol. 2009;157:153–165.
- Ickenstein LM, Garidel P. Lipid-based nanoparticle formulations for small molecules and RNA drugs. Expert Opin Drug Deliv. 2019;16:1205–1226.
- Evers MJW, Kulkarni JA, van der Meel R, et al. State-of-the-art design and rapid-mixing production techniques of lipid nanoparticles for nucleic acid delivery. Small. 2018;2:1700375.
- Maeki M, Uno S, Niwa A, et al. Microfluidic technologies and devices for lipid nanoparticle-based RNA delivery. J Control Release. 2022;344:80–96.
- Ramamoorth M, Narvekar A. Nonviral vectors in gene therapy - An overview. J Clin Diagn Res. 2015;9:1–6.
- Thomas CE, Ehrhardt A, Kay MA. Progress and problems with the use of viral vectors for gene therapy. Nat Rev Genet. 2003;4:346–358.
- Jafari M, Soltani M, Naahidi S, et al. Nonviral approach for targeted nucleic acid delivery. Curr Med Chem. 2012;19:197–208.
- Pathak K, Keshri L, Shah M. Lipid nanocarriers: influence of lipids on product development and pharmacokinetics. Crit Rev Ther Drug Carrier Syst. 2011;28:357–393.
- Bobo D, Robinson KJ, Islam J, et al. Nanoparticle-based medicines: a review of FDA-approved materials and clinical trials to date. Pharm Res. 2016;33:2373–2387.
- Anselmo AC, Mitragotri S. Nanoparticles in the clinic: an update. Bioeng Transl Med. 2019;4:e10143.
- Bisso S, Leroux JC. Nanopharmaceuticals: a focus on their clinical translatability. Int J Pharm. 2020;578:119098.
- Wicki A, Witzigmann D, Balasubramanian V, et al. Nanomedicine in cancer therapy: challenges, opportunities, and clinical applications. J Control Release. 2015;200:138–157.
- Bozzuto G, Molinari A. Liposomes as nanomedical devices. Int J Nanomedicine. 2015;10:975–999.
- Çağdaş M, Sezer AD, Bucak S. Liposomes as potential drug carrier systems for drug delivery. Appl nanotechnol drug del. 2014;1:1–50.
- Samad A, Sultana Y, Aqil M. Liposomal drug delivery systems: an update review. Curr Drug Deliv. 2007;4:297–305.
- Wissing SA, Kayser O, Müller RH. Solid lipid nanoparticles for parenteral drug delivery. Adv Drug Deliv Rev. 2004;56:1257–1272.
- Barros SA, Gollob JA. Safety profile of RNAi nanomedicines. Adv Drug Deliv Rev. 2012;64:1730–1737.
- Desfrançois C, Auzély R, Texier I. Lipid nanoparticles and their hydrogel composites for drug delivery: a review. Pharmaceuticals. 2018;11:118.
- Manjunath K, Ready JS, Venkateswarlu V. Solid lipid nanoparticles as drug delivery systems. Methods Find Exp Clin Pharmacol. 2005;27:127–144.
- Maeda H. SMANCS and polymer-conjugated macromolecular drugs: advantages in cancer chemotherapy. Adv Drug Deliv Rev. 2001;46:169–185.
- Kim CH, Lee SG, Kang MJ, et al. Surface modification of lipid-based nanocarriers for cancer cell-specific drug targeting. J Pharm Invest. 2017;47:203–227.
- Wang M, Thanou M. Targeting nanoparticles to cancer. Pharmacol Res. 2010;62:90–99.
- Attia MF, Anton N, Wallyn J, et al. An overview of active and passive targeting strategies to improve the nanocarriers efficiency to tumour sites. J Pharm Pharmacol. 2019;71:1185–1198.
- Richards DA, Maruani A, Chudasama V. Antibody fragments as nanoparticle targeting ligands: a step in the right direction. Chem Sci. 2017;8:63–77.
- Wu L, Tang C, Yin C. Folate-mediated solid-liquid lipid nanoparticles for paclitaxel-coated poly(ethylene glycol). Drug Dev Ind Pharm. 2010;36:439–448.
- Zhang S, Lu C, Zhang X, et al. Targeted delivery of etoposide to cancer cells by folate-modified nanostructured lipid drug delivery system. Drug Deliv. 2016;23:1838–1845.
- Ucar E, Teksoz S, Ichedef C, et al. Synthesis, characterization and radiolabeling of folic acid modified nanostructured lipid carriers as a contrast agent and drug delivery system. Appl Radiat Isot. 2017;119:72–79.
- Leamon CP, Reddy JA. Folate-targeted chemotherapy. Adv Drug Deliv Rev. 2004;56:1127–1141.
- Banerjee R, Tyagi P, Li S, et al. Anisamide-targeted stealth liposomes: a potent carrier for targeting doxorubicin to human prostate cancer cells. Int J Cancer. 2004;112:693–700.
- Shan D, Li J, Cai P, et al. RGD-conjugated solid lipid nanoparticles inhibit adhesion and invasion of αvβ3 integrin-overexpressing breast cancer cells. Drug Deliv Transl Res. 2015;5:15–26.
- Song S, Mao G, Du J, et al. Novel RGD containing, temozolomide-loading nanostructured lipid carriers for glioblastoma multiforme chemotherapy. Drug Deliv. 2016;23:1404–1408.
- Su Z, Niu J, Xiao Y, et al. Effect of octreotide-polyethylene glycol(100) monostearate modification on the pharmacokinetics and cellular uptake of nanostructured lipid carrier loaded with hydroxycamptothecine. Mol Pharm. 2011;8:1641–1651.
- Su Z, Shi Y, Xiao Y, et al. Effect of octreotide surface density on receptor-mediated endocytosis in vitro and anticancer efficacy of modified nanocarrier in vivo after optimization. Int J Pharm. 2013;447:281–292.
- Taratula O, Kuzmov A, Shah M, et al. Nanostructured lipid carriers as multifunctional nanomedicine platform for pulmonary co-delivery of anticancer drugs and siRNA. J Control Release. 2013;171:349–357.
- Du J, Li L. Which one performs better for targeted lung cancer combination therapy: pre- or post-bombesin-decorated nanostructured lipid carriers? Drug Deliv. 2016;23:1799–1809.
- Liu B, Han L, Liu J, et al. Co-delivery of paclitaxel and TOS-cisplatin via TAT-targeted solid lipid nanoparticles with synergistic antitumor activity against cervical cancer. Int J Nanomedicine. 2017;12:955–968.
- Landesman-Milo D, Goldsmith M, Leviatan Ben-Arye S, et al. Hyaluronan grafted lipid-based nanoparticles as RNAi carriers for cancer cells. Cancer Lett. 2013;334:221–227.
- Mohammadi Ghalaei P, Varshosaz J, Sadeghi Aliabadi H. Evaluating cytotoxicity of hyaluronate targeted solid lipid nanoparticles of etoposide on SK-OV-3 cells. J Drug Deliv. 2014;2014:1–7.
- Tran TH, Choi JY, Ramasamy T, et al. Hyaluronic acid-coated solid lipid nanoparticles for targeted delivery of vorinostat to CD44 overexpressing cancer cells. Carbohydr Polym. 2014;114:407–415.
- Qu CY, Zhou M, Chen YW, et al. Engineering of lipid prodrug-based, hyaluronic acid-decorated nanostructured lipid carriers platform for 5-fluorouracil and cisplatin combination gastric cancer therapy. Int J Nanomedicine. 2015;10:3911–3920.
- Shen H, Shi S, Zhang Z, et al. Coating solid lipid nanoparticles with hyaluronic acid enhances antitumor activity against melanoma stem-like cells. Theranostics. 2015;5:755–771.
- Jain A, Agarwal A, Majumder S, et al. Mannosylated solid lipid nanoparticles as vectors for site-specific delivery of an anti-cancer drug. J Control Release. 2010;148:359–367.
- Jain A, Kesharwani P, Garg NK, et al. Galactose engineered solid lipid nanoparticles for targeted delivery of doxorubicin. Colloids Surf B Biointerfaces. 2015;134:47–58.
- van der Jeught K, de Koker S, Bialkowski L, et al. Dendritic cell targeting mRNA lipopolyplexes combine strong antitumor T-cell immunity with improved inflammatory safety. ACS Nano. 2018;12:9815–9829.
- Khajavinia A, Varshosaz J, Dehkordi AJ. Targeting etoposide to acute myelogenous leukaemia cells using nanostructured lipid carriers coated with transferrin. Nanotechnology. 2012;23:405101.
- Han Y, Zhang Y, Li D, et al. Transferrin-modified nanostructured lipid carriers as multifunctional nanomedicine for codelivery of DNA and doxorubicin. Int J Nanomedicine. 2014;9:4107–4116.
- Shao Z, Shao J, Tan B, et al. Targeted lung cancer therapy: preparation and optimization of transferrin-decorated nanostructured lipid carriers as novel nanomedicine for co-delivery of anticancer drugs and DNA. Int J Nanomedicine. 2015;10:1223–1233.
- Layek B, Lipp L, Singh J. Cell penetrating peptide conjugated chitosan for enhanced delivery of nucleic acid. Int J Mol Sci. 2015;16:28912–28930.
- Asai T, Tsuzuku T, Takahashi S, et al. Cell-penetrating peptide-conjugated lipid nanoparticles for siRNA delivery. Biochem Biophys Res Commun. 2014;444:599–604.
- Li Y, Lee RJ, Yu K, et al. Delivery of siRNA using lipid nanoparticles modified with cell penetrating peptide. ACS Appl Mater Interfaces. 2016;8:26613–26621.
- Kedmi R, Ben-Arie N, Peer D. The systemic toxicity of positively charged lipid nanoparticles and the role of Toll-like receptor 4 in immune activation. Biomaterials. 2010;31:6867–6875.
- Rizwan SB, McBurney WT, Young K, et al. Cubosomes containing the adjuvants imiquimod and monophosphoryl lipid A stimulate robust cellular and humoral immune responses. J Control Release. 2013;165:16–21.
- Roces CB, Lou G, Jain N, et al. Manufacturing considerations for the development of lipid nanoparticles using microfluidics. Pharmaceutics. 2020;12:1095.
- Berger N, Sachse A, Bender J, et al. Filter extrusion of liposomes using different devices: comparison of liposome size, encapsulation efficiency, and process characteristics. Int J Pharm. 2001;223:55–68.
- Comuzzo P, Calligaris S. Potential applications of high pressure homogenization in winemaking: a review. Beverages. 2019;5:56.
- International Organization for Standardization. 2022. ISO 10991:2009 micro process engineering — Vocabulary. https://www.iso.org/standard/46546.html. [Accessed 2022 Jun 15]
- Khan IU, Serra CA, Anton N, et al. Production of nanoparticle drug delivery systems with microfluidics tools. Expert Opin Drug Deliv. 2015;12:547–562.
- Mukhopadhyay R. When PDMS isn’t the best. Anal Chem. 2007;79:3248–3253.
- Karnik R, Gu F, Basto P, et al. Microfluidic platform for controlled synthesis of polymeric nanoparticles. Nano Lett. 2008;8:2906–2912.
- Nisisako T, Torii T. Microfluidic large-scale integration on a chip for mass production of monodisperse droplets and particles. Lab Chip. 2008;8:287–293.
- Mukhopadhyay R. When microfluidic devices go bad. Anal Chem. 2005;77:429–432.
- Silverio V, S C. Lab-on-a-chip: systems integration at the microscale. Drug Delivery Devices Therapeutic Systems. 2021;4:63–87.
- Mansur EA, Ye M, Wang Y, et al. A state-of-the-art review of mixing in microfluidic mixers. Chin J Chem Eng. 2008;16:503–516.
- Maeki M, Fujishima Y, Sato Y, et al. Understanding the formation mechanism of lipid nanoparticles in microfluidic devices with chaotic micromixers. PLOS ONE. 2017;12:e187962.
- de Menech M, Garstecki P, Jousse F, et al. Transition from squeezing to dripping in a microfluidic T-shaped junction. J Fluid Mech. 2008;595:141–161.
- Prakash S, Kumar S 2015. Fabrication of microchannels: a review. Proceedings of the Institution of Mechanical Engineers, Part B: Journal of Engineering Manufacture San Diego (CA), USA. 229:1–16.
- Krzysztoń R, Salem B, Lee DJ, et al. Microfluidic self-assembly of folate-targeted monomolecular siRNA-lipid nanoparticles. Nanoscale. 2017;9:7442–7453.
- Kimura N, Maeki M, Sato Y, et al. Development of the iLiNP device: fine tuning the lipid nanoparticle size within 10 nm for drug delivery. ACS Omega. 2018;3:5044–5051.
- Webb C, Forbes N, Roces CB, et al. Using microfluidics for scalable manufacturing of nanomedicines from bench to GMP: a case study using protein-loaded liposomes. Int J Pharm. 2020;582:119266.
- Belliveau NM, Huft J, Lin PJ, et al. Microfluidic synthesis of highly potent limit-size lipid nanoparticles for in vivo delivery of siRNA. Mol Ther Nucleic Acids. 2012;1:e37.
- Arteta MY, Kjellman T, Bartesaghi S, et al. 2018. Successful reprogramming of cellular protein production through mRNA delivered by functionalized lipid nanoparticles. Proceedings of the National Academy of Sciences. 115:3351–3360.
- Chen S, Tam YYC, Lin PJC, et al. Influence of particle size on the in vivo potency of lipid nanoparticle formulations of siRNA. J Control Release. 2016;235:236–244.
- Liang F, Lindgren G, Lin A, et al. Efficient targeting and activation of antigen-presenting cells in vivo after modified mRNA vaccine administration in rhesus macaques. Mol Ther. 2017;25:2635–2647.
- VanBlargan LA, Himansu S, Foreman BM, et al. An mRNA vaccine protects mice against multiple tick-transmitted flavivirus infections. Cell Rep. 2018;25:3382–3392.
- Zeng C, Hou X, Yan J, et al. Leveraging mRNAs sequences to express SARS-CoV-2 antigens in vivo. bioRxiv. 2020;32:e2004452.
- Corbett KS, Flynn B, Foulds KE, et al. Evaluation of the mRNA-1273 vaccine against SARS-CoV-2 in nonhuman primates. N Engl J Med. 2020;383:1544–1555.
- Richner JM, Himansu S, Dowd KA, et al. Modified mRNA vaccines protect against zika virus infection. Cell. 2017;168:1114–1125.
- Awasthi S, Hook LM, Pardi N, et al. Nucleoside-modified mRNA encoding HSV-2 glycoproteins C, D, and E prevents clinical and subclinical genital herpes. Sci Immunol. 2019;4(39):eaaw7083.
- John S, Yuzhakov O, Woods A, et al. Multi-antigenic human cytomegalovirus mRNA vaccines that elicit potent humoral and cell-mediated immunity. Vaccine. 2018;36(12):1689–1699.
- Roth C, Cantaert T, Colas C, et al. A modified mRNA vaccine targeting immunodominant NS epitopes protects against dengue virus infection in HLA class I transgenic mice. Front Immunol. 2019;10:1424.
- Meyer M, Huang E, Yuzhakov O, et al. Modified mRNA-based vaccines elicit robust immune responses and protect guinea pigs from Ebola virus disease. J Infect Dis. 2018;217(3):451–455.
- Miao L, Li L, Huang Y, et al. Delivery of mRNA vaccines with heterocyclic lipids increases anti-tumor efficacy by STING-mediated immune cell activation. Nat Biotechnol. 2019;37(10):1174–1185.
- Ramaswamy S, Tonnu N, Tachikawa K, et al. Systemic delivery of factor IX messenger RNA for protein replacement therapy. Proc Nat Acad Sci. 2017;114(10):1941–1950.
- Jiang L, Berraondo P, Jericó D, et al. Systemic messenger RNA as an etiological treatment for acute intermittent porphyria. Nat Med. 2018;24(12):1899–1909.
- Zhang C, Maruggi G, Shan H, et al. Advances in mRNA vaccines for infectious diseases. Front Immunol. 2019;10:594.
- Tiwari PM, Vanover D, Lindsay KE, et al. Engineered mRNA-expressed antibodies prevent respiratory syncytial virus infection. Nat Commun. 2018;9:3999.
- Manara C, Brazzoli M, Piccioli D, et al. Co-administration of GM-CSF expressing RNA is a powerful tool to enhance potency of SAM-based vaccines. Vaccine. 2019;37:4204–4213.
- Bahl K, Senn JJ, Yuzhakov O, et al. Preclinical and clinical demonstration of immunogenicity by mRNA vaccines against H10N8 and H7N9 influenza viruses. Mol Ther. 2017;25:1316–1327.
- Lindgren G, Ols S, Liang F, et al. Induction of Robust B cell responses after influenza mRNA vaccination is accompanied by circulating hemagglutinin-specific ICOS+ PD-1+ CXCR3+ T follicular helper cells. Front Immunol. 2017;8:1539.
- Hassett KJ, Benenato KE, Jacquinet E, et al. Optimization of lipid nanoparticles for intramuscular administration of mRNA vaccines. Mol Ther Nucleic Acids. 2019;15:1–11.
- Thran M, Mukherjee J, Pönisch M, et al. mRNA mediates passive vaccination against infectious agents, toxins, and tumors. EMBO Mol Med. 2017;9(10):1434–1447
- Pardi N, Hogan MJ, Pelc RS, et al. Zika virus protection by a single low-dose nucleoside-modified mRNA vaccination. Nature. 2017;543:248–251.
- Pardi N, Secreto AJ, Shan X, et al. Administration of nucleoside-modified mRNA encoding broadly neutralizing antibody protects humanized mice from HIV-1 challenge. Nat Commun. 2017;8:14630.
- Kose N, Fox JM, Sapparapu G, et al. A lipid-encapsulated mRNA encoding a potently neutralizing human monoclonal antibody protects against chikungunya infection. Sci Immunol. 2019;4:eaaw6647.
- Oberli MA, Reichmuth AM, Dorkin JR, et al. Lipid Nanoparticle Assisted mRNA Delivery for Potent Cancer Immunotherapy. Nano Lett. 2017;17(3):1326–1335.
- Lee K, Kim SY, Seo Y, et al. Adjuvant incorporated lipid nanoparticles for enhanced mRNA-mediated cancer immunotherapy. Biomater Sci. 2020;8(4):1101–1105.
- Nabhan JF, Wood KM, Rao VP, et al. Intrathecal delivery of frataxin mRNA encapsulated in lipid nanoparticles to dorsal root ganglia as a potential therapeutic for Friedreich’s ataxia. Sci Rep. 2016;6(1):20019.
- Robinson E, MacDonald KD, Slaughter K, et al. Lipid nanoparticle-delivered chemically modified mRNA restores chloride secretion in cystic fibrosis. Mol Ther. 2018;26(8):2034–2046.
- Dumpa N, Goel K, Guo Y, et al. Stability of Vaccines. AAPS PharmSciTech. 2019;20(2):42.
- Chen C, Yang Z, Tang X. Chemical modifications of nucleic acid drugs and their delivery systems for gene-based therapy. Med Res Rev. 2018;38(3):829–869.
- Howard MD, Lu X, Jay M, et al. Optimization of the lyophilization process for long-term stability of solid–lipid nanoparticles. Drug Dev Ind Pharm. 2012;38(10):1270–1279.
- Fonte P, Andrade F, Azevedo C, et al. Effect of the freezing step in the stability and bioactivity of protein-loaded PLGA nanoparticles upon lyophilization. Pharm Res. 2016;33(11):2777–2793.
- Abdelwahed W, Degobert G, Fessi H. Investigation of nanocapsules stabilization by amorphous excipients during freeze-drying and storage. Eur J Pharm Biopharm. 2006;63(2):87–94.
- Jones KL, Drane D, Gowans EJ. Long-term storage of DNA-free RNA for use in vaccine studies. Biotechniques. 2007;43(5):675–681.
- Petsch B, Schnee M, Vogel AB, et al. Protective efficacy of in vitro synthesized, specific mRNA vaccines against influenza A virus infection. Nat Biotechnol. 2012;30(12):1210–1216.
- Stitz L, Vogel A, Schnee M, et al. A thermostable messenger RNA based vaccine against rabies. PLoS Negl Trop Dis. 2017;11(12):e0006108.
- Alberer M, Gnad-Vogt U, Hong HS, et al. Safety and immunogenicity of a mRNA rabies vaccine in healthy adults: an open-label, non-randomised, prospective, first-in-human phase 1 clinical trial. Lancet. 2017;390(10101):1511–1520.
- Zhao P, Hou X, Yan J, et al. Long-term storage of lipid-like nanoparticles for mRNA delivery. Bioact Mater. 2020;5(2):358–363.
- Yusuf H, Kett V. Current prospects and future challenges for nasal vaccine delivery. Hum Vaccines Immunother. 2017;13(1):34–45.
- Tonnis WF, Kersten GF, Frijlink HW, et al. Pulmonary vaccine delivery: a realistic approach? J Aerosol Med Pulm Drug Deliv. 2012;25(5):249–260.
- Chablani L, Tawde SA, D’Souza MJ. Spray-dried microparticles: a potential vehicle for oral delivery of vaccines. J Microencapsul. 2012;29(4):388–397.
- Freitas C, Müller RH. Spray-drying of solid lipid nanoparticles (SLNTM). Eur J Pharm Biopharm. 1998;46(2):145–151.
- Mehnert W. Solid lipid nanoparticles Production, characterization and applications. Adv Drug Deliv Rev. 2001;47(2–3):165–196.
- Kanojia G, Have R RT, Soema PC, et al. Developments in the formulation and delivery of spray dried vaccines. Hum Vaccines Immunother. 2017;13(10):2364–2378.
- Gaspar DP, Serra C, Lino PR, et al. Microencapsulated SLN: an innovative strategy for pulmonary protein delivery. Int J Pharm. 2017;516(1–2):231–246.
- Wang T, Ma X, Lei Y, et al. Solid lipid nanoparticles coated with cross-linked polymeric double layer for oral delivery of curcumin. Colloids Surf B Biointerfaces. 2016;148:1–11.
- Wang T, Hu Q, Zhou M, et al. Preparation of ultra-fine powders from polysaccharide-coated solid lipid nanoparticles and nanostructured lipid carriers by innovative nano spray drying technology. Int J Pharm. 2016;511:219–222.
- Sithole MN, Choonara YE, du Toit LC, et al. Development of a novel polymeric nanocomposite complex for drugs with low bioavailability. AAPS PharmSciTech. 2018;19:303–314.
- Lokras C, Cano-Garcia A, Wadhwa G, et al. Identification of factors of importance for spray drying of small interfering RNA-loaded lipidoid-polymer hybrid nanoparticles for inhalation. Pharm Res. 2019;36:142.
- Patel AK, Kaczmarek JC, Bose S, et al. Inhaled nanoformulated mRNA polyplexes for protein production in lung epithelium. Adv Mater. 2019;31:e1805116.
- Qiu Y, Man R, Liao Q, et al. Effective mRNA pulmonary delivery by dry powder formulation of PEGylated synthetic KL4 peptide. J Control Release. 2019;314:102–115.
- Globenewswire. 2022. https://www.globenewswire.com/en/news-release/2022/07/19/2481569/0/en/The-global-microfluidic-devices-market-is-predicted-to-reach-US-34-5-Billion-by-2028.html. [Accessed 2022 August 1]
- Shepherd SJ, Warzecha CC, Yadavali S, et al. Scalable mRNA and siRNA lipid nanoparticle production using a parallelized microfluidic device. Nano Lett. 2021;21:5671–5680.
- Carvalho BG, Ceccato BT, Michelon M, et al. Advanced microfluidic technologies for lipid nano-microsystems from synthesis to biological application. Pharmaceutics. 2022;14:141.
- Pagels RF, Edelstein J, Tang C, et al. Controlling and predicting nanoparticle formation by block copolymer directed rapid precipitations. Nano Lett. 2018;18:1139–1144.
- Johnson BK, Prud’homme RK. Chemical processing and micromixing in confined impinging jets. AIChE J. 2003;49:2264–2282.
- Johnson BK, Prud’homme RK. Mechanism for rapid self-assembly of block copolymer nanoparticles. Phys Rev Lett. 2003;91:1–4.
- Lim JM, Swami A, Gilson LM, et al. Ultra-high throughput synthesis of nanoparticles with homogeneous size distribution using a coaxial turbulent jet mixer. ACS Nano. 2014;8:6056–6065.
- Chemtrix. 2022. https://chemtrix.com/news/pfizer-showcase-industrial-scale-continuous-manufacturing [Accessed 2022 August 1]