ABSTRACT
Introduction
Perinatal asphyxia (PA) still causes significant morbidity and mortality. Therapeutic hypothermia (TH) is the only effective therapy for neonates with moderate to severe hypoxic-ischemic encephalopathy after PA. These neonates need additional pharmacotherapy, and both PA and TH may impact physiology and, consequently, pharmacokinetics (PK) and pharmacodynamics (PD).
Areas covered
This review provides an overview of the available knowledge in PubMed (until November 2022) on the pathophysiology of neonates with PA/TH. In vivo pig models for this setting enable distinguishing the effect of PA versus TH on PK and translating this effect to human neonates. Available asphyxia pig models and methodological considerations are described. A summary of human neonatal PK of supportive pharmacotherapy to improve neurodevelopmental outcomes is provided.
Expert opinion
To support drug development for this population, knowledge from clinical observations (PK data, real-world data on physiology), preclinical (in vitro and in vivo (minipig)) data, and molecular and cellular biology insights can be integrated into a predictive physiologically-based PK (PBPK) framework, as illustrated by the I-PREDICT project (Innovative physiology-based pharmacokinetic model to predict drug exposure in neonates undergoing cooling therapy). Current knowledge, challenges, and expert opinion on the future directions of this research topic are provided.
1. Pathophysiology of the asphyxiated neonate receiving therapeutic hypothermia
1.1. Definitions and general aspects
Perinatal asphyxia (PA) is a medical condition at delivery caused by oxygen deprivation, potentially resulting in hypoxic-ischemic encephalopathy (HIE) [Citation1]. Approximately 0.5% of all live-born neonates are affected by PA, and about 0.18% are diagnosed with HIE (mild, moderate, or severe). Perinatal asphyxia is still one of the most common causes of death in (near)term neonates [Citation2]. Oxygen deprivation is reflected in the diagnostic clues for HIE, consisting of clinical findings (Apgar score and Thomson score), and findings based on either laboratory values (arterial blood base excess, lactate, and pH for acidosis) or monitoring (electroencephalographic (EEG) activity) [Citation1,Citation3]. Therapeutic hypothermia (TH; target core temperature of 33.5°C for 72 hours with subsequent slow rewarming at 0.5°C/hour) is effective in reducing mortality or impaired neurodevelopmental outcome in moderate to severe HIE (number needed to treat 7, outcome variable decreases from about 55% to 45%) [Citation4,Citation5].
1.2. The pathophysiology of asphyxia, modulated by therapeutic hypothermia
Asphyxia is a syndrome affecting multiple organs, including the central nervous system (CNS). The initial cardiovascular response to oxygen depletion results in organ-specific vasoconstriction. This subsequently results in perfusion and oxygen supply redistribution from non-vital organs to the heart and brain. Other organs, such as the kidneys, liver, and intestines, are in a low perfusion setting [Citation6,Citation7]. Therefore, it is essential to be aware that TH further modulates the ’natural’ progression of the pathophysiology of asphyxia. As TH is only indicated in (near)term neonates with moderate or severe HIE, this mainly refers to (patho)physiological parameters on cardiac output (CO; stroke volume, myocardial strain, and heart rate), regional blood flow (pulmonary hypertension, cerebral, renal, and hepatic blood flow), renal function (glomerular filtration rate, GFR), plasma composition (albumin, hematocrit, and α-1 glycoprotein) and intestinal functions (gastric emptying, intestinal motility, and intestinal permeability). Using an exploratory search in PubMed on PA and TH in human neonates until November 2022, we have listed pathophysiological characteristics of system-specific functions in asphyxiated neonates undergoing TH or not (). This approach has the intention to provide the reader with a snapshot of system-specific observations, but is not complete, nor does it claim to be systematic.
Table 1. System-specific observations on the impact of perinatal asphyxia on neonatal physiology, with or without therapeutic hypothermia.
We will further discuss the cardiovascular system, renal impairment, and acute kidney injury (AKI), and hepatic impairment, including drug-induced liver injury (DILI) diagnosis. All these aspects can significantly affect pharmacokinetics (PK) and should therefore be considered in physiologically-based pharmacokinetic (PBPK) models. Furthermore, AKI and DILI are relevant to tailor pharmacovigilance in this population.
1.2.1. Cardiovascular system
The CO is affected by the disease (PA) and the treatment (TH), as there are significant reductions in cardiac biomarkers and less myocardial dysfunction on electrocardiography (ECG) and ultrasound in TH cases compared to non-TH asphyxia cases [Citation9]. The myocardial function (strain rate about 50% slower) is mainly determined by asphyxia, while hypothermia reduces the heart rate [Citation8]. Gebauer et al. documented in a paired study that CO during whole-body hypothermia (33°C) was significantly lower when compared to normothermia (37°C) (median and range: 169 (157–202) versus 254 (241–339) mL/kg/min) [Citation20]. Right and left ventricular output in asphyxiated whole-body TH cases compared to normative values were 108 (−51%) and 107 (−52%) compared to 224 and 222 mL/kg/min, respectively [Citation21]. There is also an even more pronounced decrease in intestinal and hepatic blood flow, reflected by an increased blood flow velocity via ultrasound, with a subsequent increase during and after rewarming [Citation22].
1.2.2. Renal impairment and acute kidney injury
For drugs that are mainly eliminated by the kidneys (glomerular filtration (GF) + renal tubular processes), and used during PA/TH, reports consistently observed a significant decrease in clearance (−30 to −60%) compared to non-asphyxia control cases, with an increase in clearance over postnatal age (PNA). Interestingly, the highest reduction in clearance (40–60%) seems to be present during the first day of life, with less pronounced differences afterward (<30%). Clearance becomes similar to healthy controls in the second part of the first week of life [Citation23]. Favié et al., classifying renally eliminated drugs as part of the high-clearance drugs, estimated a time-dependent maturation function of clearance (1.23%/hour), far exceeding the effects of maturation on clearance (estimated to be 0.05%/hour) [Citation24]. These authors also stressed the large (71.6%) interindividual variability in clearance, as these patterns are also reflected in datasets on serum creatinine (sCr) values in PA/TH cases compared to controls [Citation24].
1.2.3. Hepatic metabolism and drug-induced liver injury
Favié et al. also estimated a maturation function for intermediate clearance drugs (0.54%/hour; liver metabolism mediated), which still exceeded the estimated maturation of ~ 0.05%/hour [Citation24]. When quantified by temperature reduction (between 33.5°C and 37°C,) clearance changed by 6.83%/°C and was, therefore, 23.9% lower at 33.5°C compared to 37°C.
When screening for DILI, there are certain commonly used biomarkers. However, there are limitations when using these to screen for DILI in neonates, as (indirect) hyperbilirubinemia is commonly part of neonates’ normal developmental physiology. Neonatal hyperbilirubinemia has a natural trend over time, with a peak on days 3 to 4 and a subsequent decrease over the first 10 to 14 days of PNA. However, the peak and mean total serum bilirubin levels are lower in asphyxia cases, either undergoing TH or not [Citation25,Citation26]. Among others, Muniraman et al. and Michniewicz et al. reported on liver enzyme patterns in asphyxia cases () [Citation3,Citation14]. Liver-injury-related enzymes (alanine transaminase (ALT) and aspartate transaminase (AST)) are higher in asphyxia cases during hospital stays when compared to healthy neonates and are modulated by HIE severity.
Table 2. Overview of median values and the range of liver enzymes (ALT = alanine transaminase; AST = aspartate transaminase; highest value observed during hospital stay) for the different grades (1–3) of hypoxic-ischemic encephalopathy (HIE) in two published cohorts [Citation3,Citation14].
1.2.4. The pathophysiological implications of hypoxic-ischemic encephalopathy and therapeutic hypothermia on the brain
Three main consecutive phases associated with neuronal cell damage occur in neonates with HIE; the primary phase and the latent phase, which consists of the secondary and tertiary phases. A first peak of neuronal cell damage occurs during the primary phase and is caused by primary energy failure during the hypoxic event at birth. A second peak results from subsequent reoxygenation and reperfusion after birth (‘reperfusion injury;’ latent phase). In the primary phase, the acute hypoxia shortly before or during delivery results in primary oxygen and energy deficiency, resulting in depolarization, potassium efflux, sodium and calcium influx, adenosine triphosphate (ATP) degradation, and finally resulting in cell necrosis if the event is sufficiently severe or prolonged. Subsequently, this results in the release of excitatory neurotransmitters, with glutamate as the most relevant compound (with subsequent N-methyl-D-aspartate (NMDA) receptor activation). However, the neonatal brain releases proportionally more inhibitory neurotransmitters, which is likely to contribute to the remarkable tolerance of the neonatal brain to hypoxia and ischemia [Citation1,Citation27].
During the latent phase (from 6–8 hours onwards), the secondary phase further causes cell damage and induces an inflammatory response. The subsequent apoptotic activity and the downregulation of trophic factors further contribute to neuronal cell injury. This latent phase can last for days (such as mitochondrial dysfunction and inflammatory response; secondary phase) up to weeks/years (tertiary phase) [Citation1,Citation27]. The primary phase is, therefore, the time window relevant to initiate TH to blunt the secondary phase, while the secondary phase is the ‘main target time interval’ for most ongoing drug development programs, like allopurinol, 2-iminobiotin, or erythropoietin (EPO) [Citation28–30]. illustrates the phases of injury, fundamental mechanisms, and potential drug targets to improve outcomes [Citation1].
Figure 1. Flow chart on mechanisms involved in the consecutive phases of neonatal hypoxic-ischemic encephalopathy over postnatal age. Adapted from Davidson et al. and used with permission from Elsevier [Citation1]. ATP = adenosine triphosphate; RIP-1 kinase = receptor-interacting serine/threonine-protein kinase 1.
![Figure 1. Flow chart on mechanisms involved in the consecutive phases of neonatal hypoxic-ischemic encephalopathy over postnatal age. Adapted from Davidson et al. and used with permission from Elsevier [Citation1]. ATP = adenosine triphosphate; RIP-1 kinase = receptor-interacting serine/threonine-protein kinase 1.](/cms/asset/4dc2d490-2b4b-4812-9825-22df68f32939/iemt_a_2237412_f0001_b.gif)
The following sections will first discuss the impact of hypothermia on biological processes, including gene expression and protein activity (section 2). This will be followed by a discussion of the current pharmacological strategies (section 3). We hereby distinguish between research lines for add-on pharmacotherapy during TH to improve neurodevelopmental outcomes and clinical pharmacology knowledge on drug classes used in the supportive care of neonates with PA/TH. Furthermore, comparative in vivo neonatal HIE pig models for drug development (section 4) and the relevance of real-world human neonatal data to improve pharmacotherapy (section 5) are summarized. Finally, the concept for developing a PBPK hypothermia model is covered in section 6. Besides an overview of currently available parameters in PBPK tools, the essential changes to modify these tools to a PA/TH setting are also discussed.
2. Impact of hypothermia on biological processes
This section will address current knowledge regarding cellular and molecular mechanisms of essential biochemical pathways affected by hypothermia. Attention was given to pathways (potentially) interfering with the function or expression of absorption, distribution, metabolism, and excretion (ADME) of relevant and/or pharmacological targets. The subsections will describe (2.1.) the modulation of hypoxia-associated events by TH; (2.2.) the fundamental knowledge regarding the influence of hypothermia on biological systems, with attention to pharmacologically relevant genes and proteins.
2.1. Modulation of hypoxia-associated events by therapeutic hypothermia
Several reviews have addressed the mechanisms governing neuroprotection during hypothermia in acute neurological diseases characterized by hypoxia (such as HIE) [Citation31–33].
Across acute and subacute ischemic phases, TH has been described to specifically modulate cellular pathways leading to (1) excitotoxicity; (2) apoptosis; (3) inflammation; (4) free radical production. Moreover, TH will modulate blood flow, slow overall cellular metabolism, and support blood-brain barrier integrity. The latter will be vital in determining CNS access of administered drugs. In the acute phase, hypothermia will cause a decrease in brain oxygen consumption and glucose metabolism of about 5%/°C. Hypothermia thus preserves ATP levels and pH, eventually preventing (excessive) lactate formation and acidosis. Notably, at least theoretically, the maintenance of ATP levels is expected to play an important role in keeping ATP-binding cassette (ABC) transporters (many are drug efflux transporters) functional. However, intrinsic protein function (independent of ATP supply) will also determine drug transporter activity at reduced temperatures. Based on in vitro transport experiments in cells transfected with multidrug resistance 1 (MDR1, also known as P-glycoprotein or P-gp), Jin et al. demonstrated a decrease of transport of the P-gp substrate digoxin up to 50% at 32°C compared to 37°C [Citation34]. However, the effect was limited to a 20% reduction for the P-gp substrate quinidine. At the same time, the impact of mild hypothermia (32–35°C) on passive diffusion (via trans- or paracellular pathways) appeared negligible.
The effects of TH on the blood flow in damaged brain tissue during reperfusion are characterized by a blunted overshoot as TH prevents the release of excitatory amino acids (e.g. glutamate). This is typically explained in terms of better maintenance of ion homeostasis, thus preventing necrosis.
Three early molecular/cellular events associated with ischemia, and which are likely affected by hypothermia include (1) immediate early gene (IEG) expression; (2) cellular stress response; (3) micro-ribonucleic acid (miRNA)-mediated signaling. TH induces the expression of so-called IEGs, which are known to encode transcription factors. Kamme et al. described the induction of the IEGs in the primary (1–2 hours after reperfusion) and secondary phase (12–36 hours after reperfusion) [Citation35]. Hypothermia accelerated the expression of some IEGs, which was interpreted as a more rapid recovery of intracellular signaling of neurons after ischemic insult. The neuroprotective effect of hypothermia via IEG modulation has been confirmed in animals [Citation36]. Global expression profiling efforts consistently revealed remarkable temperature-induced changes in the expression of neuroprotection factors in rat brains [Citation37].
MiRNA is a type of non-coding RNA playing a role in cellular signaling pathways and has been shown to exhibit increased expression within 2 hours of ischemic insults. At the same time, hypothermia can modulate the expression of specific miRNAs [Citation38,Citation39]. In recent years, several independent studies have elucidated particular miRNA profiles associated with HIE and TH, thereby opening the door to using umbilical cord blood levels of specific miRNAs as prognostic biomarkers for the early diagnosis and the grading of HIE injury [Citation40].
Winkler et al. discovered that 48 hours after birth, miR-145-5p is significantly increased in neonates with a poor neurodevelopmental outcome after PA compared to those with a good neurodevelopmental outcome and moderate to severe HIE [Citation41]. However, TH was a potential confounder in this study, as all neonates had already been treated with whole-body TH [Citation41]. O’Sullivan et al. validated the decrease of 3 miRNAs (miR-374a, miR-376c, and miR-181b) as biomarkers in umbilical cord blood of neonates with PA and HIE. Three other miRNAs (miR199a, miR-376a, and miR-410) were not validated as significantly altered in both cohorts [Citation42].
2.2. Fundamental knowledge regarding the influence of hypothermia on biological systems
2.2.1. Mechanisms governing the impact of hypothermia on gene expression
Both hypo- and hyperthermia can induce a cellular stress response, a cold shock response (CSR) and a heat shock response (HSR), respectively. Cold reduces the rates of many enzymatic reactions, slows down diffusion, and since membrane fluidity is heavily dependent on temperature, membrane transport and signaling are also affected. Low temperatures cause alterations in the properties of the lipid bilayer and might even change the fatty acid composition [Citation43,Citation44]. Denaturation of proteins is not only caused by heat but can also be caused by cold. Depending on the intensity and the time of exposure, low temperatures can trigger a cellular stress response (e.g. CSR), activate the apoptotic program, or lead to necrosis [Citation44].
The effect of TH on the cellular stress response has been studied primarily by evaluating the expression of heat shock proteins (Hsp), particularly the Hsp70 family. The Hsp70 family is upregulated during HSR and consists of anti-apoptotic proteins that help with, e.g. the folding and refolding of misfolded/aggregated proteins. An example of such a cellular stress condition is reflected in a recent study by Seo et al., which confirmed the association between Hsp70 (and Hsp47) expression and HIE severity [Citation45]. However, inconsistent findings have been reported on whether the hypothermia-altered cellular stress response is neuroprotective [Citation46]. For CSR, Matz et al. determined that Hsps could be neuroprotective in mice, while Kumar et al. found no neuroprotective effect of Hsp72 during hypothermia in gerbils after ischemia [Citation47,Citation48].
Another class of temperature-induced proteins, namely cold shock proteins (Csps), mitigate the detrimental effects of cold shock. Unlike Hsps, Csps are multifunctional RNA/DNA binding proteins [Citation49]. Csps are highly conserved across almost all species and are characterized by at least one cold shock domain involved in RNA and DNA binding [Citation49,Citation50]. The varied biological activities of Csps include transcription regulation, translation, splicing, and orchestrating the exosomal RNA content [Citation49].
Further, hypothermia can impact gene expression. In a model of hemorrhagic rats, Alam et al. observed mechanisms for changes in gene expression due to severe hypothermia (15°C) [Citation51]. Of 23 000 genes tested, 571 were affected, with 382 being up-regulated and 187 being down-regulated (Supplementary Table A). Hypothermia generally induces the up-regulation of pro-survival pathways and causes the down-regulation of several metabolic pathways, with the most significant impact on the liver [Citation51]. Research into other cellular stressors in rodents suggests inhibition of protein synthesis [Citation52,Citation53]. As hypothermia is a cellular stressor, it might also inhibit protein synthesis.
Published hypothermia studies describe a limited number of human cells (e.g. skeletal muscles, sperm, embryos, fibroblastic cells, chondrocytes, THP-1 cells, and stem cells); as a consequence, only limited data is available about the effect of temperature on, e.g. gene expression and epigenetic regulation in humans [Citation54–60]. Sonna et al. suggest a significant difference in response between CSR and HSR [Citation54]. Hypothermia can alter gene expression through (1) responses of cells to systemic signals; (2) a direct effect on cells; (3) modulating the effect of other stimuli [Citation54]. More generally, hypothermia suppresses the immune system by inducing changes in immune modulators; it increases phosphorylation and ubiquitination [Citation52,Citation54,Citation61–64]. Finally, the literature does not agree on whether hypothermia reduces or increases inflammation [Citation65–67].
2.2.2. Mechanisms governing the impact of hypothermia on protein function/activity
As mentioned above, temperature affects protein activity (e.g. enzymatic rates), and the effect on proteins depends on the temperature preference of the organism.
The first possible effect of temperature is on protein folding during synthesis. Indeed, even small temperature changes can impact the protein conformation, while more extreme temperature changes (species- and protein-dependent) can affect protein stability. Heat shock proteins can stabilize proteins at high and low temperatures by stabilizing unfolding or refolding misfolded/aggregated proteins (e.g. by cold denaturation) [Citation68]. However, current data do not suggest Hsp upregulation during CSR (section 2.2.1.), and as such, only existing Hsps can help counteract problems with folding. Folded proteins are generally highly sensitive to environmental conditions such as temperature, pH, and osmolyte concentration. Even relatively small changes in these environmental conditions can cause protein denaturation, leading to loss of protein function [Citation69]. The temperature sensitivity of proteins is due to their low net free energies of stabilization, which allows for structural flexibility [Citation70]. This structural flexibility is required to facilitate catalytic reaction by allowing necessary conformational changes [Citation69]. Different proteins undergo cold denaturation at different temperatures, and these temperatures are also dependent on other environmental conditions. Therefore, it is crucial to study the behavior of biomolecules as a function of the solution temperature to assess their biological activity and function [Citation71]. Additionally, Almeida et al. suggest that the optimal temperature range of a protein is not an intrinsic property as is reported but instead a direct consequence of the enzymatic degradation at specific experimental conditions [Citation72]. The denaturation rate is also influenced when the experimental conditions change, including enzyme concentration and assay time. Therefore, a protein’s optimal temperature range should not be considered an intrinsic property [Citation72,Citation73]. Almeida et al. highlighted that measuring the heat denaturation rates is especially important at temperatures close to or above an enzyme’s melting transition (Tm) [Citation72]. However, the actual temperature at which these effects do or do not occur varies between enzymes.
Secondly, as enzymes are energy-dependent processes, they are temperature-sensitive due to the association between heat capacity and enzyme catalysis changes in relation to different temperatures. The kinetic energy of protein substrates increases when the temperature is raised; when the kinetic energy is higher, the substrates have a higher chance of colliding and overcoming the activation energy. Generally, a reaction rate doubles with each temperature increase of 10°C, with a linear rate between a protein’s degradation temperatures [Citation74]. At certain temperatures, there is little to no temperature-driven protein degradation. However, at other temperatures, the protein denaturation (which causes a decrease in enzyme activity) can be overcome by the temperature-driven increased reaction rate [Citation72]. The temperature range in which a protein does not denature from heat or cold is organism and protein-dependent [Citation74].
Cytochrome P450s (CYPs), for example, are important enzymes for the metabolism of xenobiotics and medicines, and their activity is reduced during TH [Citation75]. CYPs can be divided into three classes, depending on their preferred ligand path [Citation76]. Preclinical studies also suggest that CYPs are affected differently by a change in temperature as their active sites have different volumes [Citation77]. CYPs with large active sites/binding pockets are CYP2C5, CYP2C8, CYP2C9, and CYP3A4. CYPs with medium active sites are CYP2B4 and CYP2D6, and CYP2A6 has a small active site. Therapeutic hypothermia affects CYPs with a narrow active site more than CYPs with a large(r) active site [Citation76,Citation78].
Thirdly, ligand binding is highly temperature sensitive as well due to its reliance on three events which can easily be disrupted (1) the geometry of the binding site in the protein; (2) the noncovalent bond establishment between ligand and binding site; (3) protein conformation change during ligand binding (applicable to many enzymes) [Citation79]. The geometry of the protein binding site depends on the stability of the protein at a certain temperature. Proteins are only slightly stable at physiological temperatures. When the temperature increases, a subtle structural change occurs in the protein, which nevertheless has a physiologically important impact on the enzyme conformation [Citation70]. Proteins need to be able to retain their 3D structure to maintain functionality. Yet high flexibility is required due to a need for rapid, precise, and reversible changes in protein assembly and conformation [Citation70,Citation79]. Regarding hypothermia, a conformational change in the binding site of an enzyme can reduce the enzyme activity. For CYPs, the temperature dependence might not be equal among the protein family. There are several differences in structures, both in the preferred paths of the ligands and the volume of the active site of these proteins [Citation76]. Otyepka et al. and Cojocaru et al. have published models of CYPs and their structural features, such as channels and active sites [Citation76,Citation80]. However, current data suggest that drug-protein binding is not significantly altered during mild to moderate hypothermia (30°C–35°C), unlike drug elimination [Citation77].
presents half-lives (t1/2) of important human enzymes and a clear variability in the estimated t1/2 of specific CYPs is observed, depending on which method was used to assess the t1/2 [Citation97]. This difference in t1/2 might mean that some proteins are more affected by a change in temperature than others. For example, paused protein synthesis at lower temperatures might have a more significant impact on proteins with a shorter t1/2.
Table 3. Half-lives (t1/2) of several human cytochrome P450 (CYP) enzymes and the method used to calculate their half-life. Table adapted from Yang et al. [Citation97].
Neonatal ontogeny (not further discussed in this review) is also relevant, as neonates have lower expression levels of CYPs and have several different isoforms than adults, such as CYP3A7 instead of CYP3A4. As discussed previously, neonates undergo rapid physiological changes in early life. This makes studying liver metabolism and the impact of PA/TH on liver metabolism challenging [Citation98].
Values in brackets show the range, and ± shows the standard deviation. * t1/2 = 0.693/kdeg. In vitro methods: (1) radio-labeling of the enzyme (‘Pulse-chase’ method); (2) degradation of enzyme in cultured hepatocytes or liver slices. In vivo methods: (3) recovery of enzyme activity after enzyme induction; (4) recovery of enzyme activity after mechanism-based inhibition (MBI); (5) PK modeling of auto-induction.
Lastly, close to the physiological temperature range, biological membranes also have a Tm [Citation99]. Transmembrane proteins such as CYPs might therefore be affected due to differences in the membrane at different temperatures. For example, CYP3A4 interacts with the membrane through specific lipid-protein interactions. This binding to the membrane significantly impacts the dynamic and structure of its globular domain [Citation100]. Therefore, a lower temperature might also influence protein activity due to the possible closing of access sites or increased rigidity and slower conformation changes. However, studies into the homeoviscous adaptation of the membrane at different temperatures suggest a total or partially self-correcting system that changes the membrane lipid composition. The composition of a membrane changes to become more rigid when exposed to higher temperatures and more flexible at lower temperatures to maintain membrane functions such as action potential [Citation101–103]. However, the homeoviscous adaptation does not seem possible at lower and higher temperatures for all cells [Citation104].
3. Pharmacological strategies for asphyxiated neonates receiving therapeutic hypothermia
3.1. Disease mechanisms and pharmacological targets
Despite the positive effect of TH, PA still accounts for a relevant portion of neonatal mortality and morbidity in (near)term neonates. Therefore, interventions to further improve outcomes are urgently needed. From a drug development aspect, PA qualifies as an orphan disease. The Committee for Orphan Medicinal Products estimates that about 15 000 neonates (0.3/10 000 EU inhabitants) are affected annually.
The specific setting to conduct clinical trials in this population makes PA/TH studies difficult. Active research is currently mainly driven by repurposing approaches like EPO, allopurinol, 2-iminobiotin, cannabidiol, melatonin, magnesium sulfate, sildenafil, vitamin C, E4, RLS-0071, or stem cells. The Helping to End Addiction Long-term Initiative (HEAL) consortium recently reported that EPO administration to neonates undergoing TH for HIE did not result in a lower risk of death or neurodevelopmental impairment than placebo and was associated with a higher rate of serious adverse events [Citation30]. Based on a search within the European Medicines Agency databank on orphan designations (April 6, 2023), we provide an overview of the current programs in the European Union ().
Table 4. Overview of retrieved orphan designation decision on Perinatal asphyxia (PA)/hypoxic-ischemic encephalopathy (HIE) from the Community Register of orphan medicinal products.
In the reviewed study protocols, phase 3 studies showed a consistency in the ‘intact’ survival without neurodevelopmental impairment as a primary outcome variable for neonates with PA/TH between 18–24 months. In contrast, short-term outcome variables showed more heterogeneity. Short-term outcome variables are biomarkers expected to be predictive for this primary outcome and useful in preceding phase 2 studies. Biomarkers include advanced EEG analyses over time throughout or after TH, Magnetic Resonance Imaging (MRI) in early neonatal life (first week) with scoring systems, or biochemical markers in blood or cerebrospinal fluid with neuron-specific enolase or S100B as the most commonly used brain-specific proteins [Citation105,Citation106]. Besides EPO, allopurinol also progressed toward a clinical phase 3 randomized controlled trial (RCT; Albino trial [Citation28]). Victor et al. summarized the status of the add-on compounds for neuroprotection in neonatal HIE, which are currently under investigation [Citation107]. Overall, therapies that further improve outcomes are highly needed and might have neuroprotective opportunities in low- and middle-income settings without access to TH.
3.2. Impact of therapeutic hypothermia on supportive pharmacotherapy
The modulation of the pathophysiology by TH in asphyxiated neonates is relevant for dosing of the commonly used drugs (antibiotics, analgosedatives, anti-epileptics, inotropes). A review by Lutz et al. analyzed 36 studies using 15 compounds. The impact of PA/TH on drug clearance was dependent on the route of elimination and was most pronounced for renal elimination during TH (no difference for phenobarbital, −25% for 1-hydroxymidazolam, −24% for lidocaine; −21% to −47% for morphine) [Citation108]. Mannitol and amikacin clearance (−40% to −60%) were estimated in the first 24–48 hours of life [Citation109,Citation110]. Based on gentamicin disposition (sampling days 2–5) in PA/TH cases, clearance was initially decreased (25–35%), with a subsequent increase observed after normothermia (96–120 hours) [Citation111]. Consequently, prolonging the dosing interval resulted in better target attainment for amikacin and gentamicin, characterized by renal elimination [Citation110,Citation112]. Population PK model-based dosing for neonates with PA receiving TH has been published for some commonly used drugs, like amikacin [Citation110]. Nevertheless, therapeutic drug monitoring of compounds with a small therapeutic window (e.g. aminoglycosides) is still recommended in these critically ill neonates to obtain safe and effective therapy.
For drugs undergoing metabolic elimination, the decreased clearance may reflect overall lower enzymatic activity with decreasing temperature or reduced hepatic blood flow. However, the underlying pathophysiological mechanisms still need further exploration and research [Citation113]. Besides opioids, alpha-2-adrenergic receptor agonists like dexmedetomidine are increasingly used for sedation and their opioid-sparing effect in neonates. The drug undergoes hydroxylation (mainly by CYP2A6) and direct N-glucuronidation in adults [Citation114]. The efficacy and short-term safety of dexmedetomidine were described in 19 neonates receiving TH [Citation115]. Despite an association with lower heart rate, Elliott et al. concluded that the compound can be used successfully in neonates with HIE receiving TH [Citation116]. In 2020, a phase 1 study was published in 7 neonates with PA/TH receiving dexmedetomidine [Citation117]. Clearance was comparable to slightly lower, and the volume of distribution was larger compared to a non-HIE, non-TH neonatal cohort but not statistically significant [Citation117]. Compared to fentanyl, dexmedetomidine appears to provide comparable control of agitation, reducing the need for additional sedatives, shorter time to extubation, and discontinuation of sedatives [Citation118]. Although the compounds seem promising, further research on optimal analgosedative pharmacotherapy in the setting of TH is requested and ongoing [Citation119,Citation120]. Besides clinical studies, preclinical research is paramount to gaining insights into this field. The following section discusses available in vivo pig models and methodological challenges.
4. Comparative in vivo neonatal hypoxic-ischemic encephalopathy pig models for drug development
4.1. Currently available in vivo pig asphyxia models
Much of the current understanding of PA in humans has been derived from animal studies, and several species have been used for this purpose [Citation121]. Among those animal models, the piglet asphyxia model is a well-established and relevant model for studying cerebral blood flow and cerebral metabolism [Citation122]. Term neonatal piglets also show a development similar to (near)term human neonates (36 to 38 weeks old) with comparable body systems and size at birth (1.5–2 kg). This represents an advantage over other species, such as rodents and even dogs and non-human primates, since this larger body size at birth allows for sampling at early stages without hampering the animal’s physiology and facilitates the adaptation of neonatal intensive care unit equipment for pigs. Furthermore, the hypoxic insult and the resulting hemodynamic derangements can be managed in the pig model, in contrast to smaller animal models where the adaptation of monitoring equipment is not feasible [Citation121]. As the piglet asphyxia model is well-characterized, this opens opportunities for pharmacological interventions and procedures applied in a clinical setting, such as TH. The following section will discuss the procedures for establishing the PA pig model.
Fetal and early postnatal (<24 hours after birth) procedures to induce PA in piglets are reported [Citation123]. Fetal procedures offer the advantage that the piglets have not been exposed yet to the external environment and may better mimic PA. However, the induced asphyxia by umbilical cord clamping appears to be not as severe as expected in human neonates, and the outcome is also very variable in piglets [Citation123]. Therefore, early postnatal procedures (<24 hours after birth) are more commonly used, and several protocols are reported. Cheung et al. described an experimental protocol of severe hypoxemia induced via normocapnic alveolar hypoxia [Citation124]. The neonatal piglets are surgically instrumented in a non-survival experiment, which allows the establishment of mechanical ventilation, arterial and central venous access, and the placement of catheters and flow probes to continuously monitor intra-vascular pressure and blood flow across different arteries. This protocol achieves severe hypoxemia by decreasing the inspired oxygen concentration to 10–15% and increasing the inhaled nitrogen gas concentration for two hours, aiming for 30–40% arterial oxygen saturation. This degree of hypoxemia produces clinical asphyxia, with severe metabolic acidosis, systemic hypotension, and cardiogenic shock with hypoperfusion of the vital organs [Citation124]. Others have described a similar piglet model for PA via whole-body hypoxia-asphyxia [Citation125]. This was induced by decreasing the inhaled oxygen to 10% for 45 minutes and the occlusion of the endotracheal tube for seven minutes [Citation125]. Global hypoxia-ischemia was established by Kyng et al., combining ischemia through hypotension with low inspiratory oxygen fraction (FiO2) via nitrogen gas and a flat trace amplitude-integrated electroencephalogram (aEEG), indicative of cerebral hypoxia [Citation126]. Survival was improved by adjusting oxygenation according to the aEEG response and arterial blood pressure. A different approach for inducing transient hypoxia-ischemia was established by remote occlusion of both common carotid arteries, using inflatable vascular occluders at the level of the fourth cervical vertebra, with a low FiO2 of 9% [Citation127]. Recently, a neonatal Göttingen minipig model for dose precision in PA has been developed, as this is the commonly used pig strain in drug development by pharmaceutical companies [Citation128]. This provides opportunities for the development of new drugs in PA.
4.2. Further methodological considerations in pig asphyxia models
When developing a piglet asphyxia model, the choice of medication, the method of controlling the insult duration and severity, post-insult resuscitation and care, and outcome evaluation should be carefully considered and monitored [Citation126]. In expected clinical asphyxia, systemic hypotension and hypoperfusion may also occur in the piglet. In hypotension, dobutamine, norepinephrine, and crystalloids can be used to maintain mean arterial blood pressure (MAP) above 45 mmHg, the lower limit of autoregulation reported in neonatal swine [Citation125]. A MAP above 40 mmHg can be sustained by administering bolus infusions of 0.9% saline and continuous infusions of dopamine, dobutamine, adrenaline, or noradrenaline [Citation127]. Neuromuscular blocking agents, such as vecuronium (0.2 mg/kg/h), are often used to prevent shivering and to provide consistent anesthesia [Citation125]. Regarding the duration of the hypoxic insult, literature data is variable, ranging from 12.5 minutes to 2 hours, as this duration depends on the method used (see section 4.1) [Citation124,Citation126]. The invasiveness of the procedures before the insult (which may result in pain, hypothermia, and potential blood loss) and the piglets’ age and weight may also impact this duration. Still, the variable duration of the insult in the different protocols is acceptable, as magnetic resonance spectroscopy analysis, EEG, brain histology, blood pressure, and/or blood gas analysis are used to assess the insult [Citation124,Citation126,Citation127,Citation129].
Nevertheless, there are also some limitations of the swine model for PA. The side effects of anesthesia and surgical trauma stress limit this model regarding survival length and the number of invasive procedures performed. The survival length may be increased with an adequate stabilizing period, appropriate use of anesthetic medications, and refined surgical techniques [Citation124]. Regarding the latter, skilled personnel with experience in surgery and neonatal piglet anesthesia are essential for these experiments. Other limitations include the logistical aspects of getting neonatal piglets in the research facility when no (pregnant) sows are housed on-site. As transporting neonatal piglets is prohibited, transporting pregnant sows to the facility will be needed. This results in many piglets that must be instrumented quickly (<24 hours) when the sow delivers. Using older piglets (two or more days of age) risks weakening the clinical relevance of the piglet model, as the model should mimic human PA [Citation130].
5. Benefits and challenges of real-world neonatal data: general reflections
5.1. Closing the gaps of special neonatal populations
Real-world data (RWD) obtained from clinical care can be used in research to generate real-world evidence [Citation131]. Sources of RWD are electronic health records, patient registries, databases, or patient-generated data from mobile or wearable devices. These data can be used to close gaps in PK and pharmacodynamics (PD) in special populations, including the safety, short-term, and long-term effects of pharmacotherapy in neonates and children [Citation132]. In addition, RWD can be applied to improve current neonatal dosing recommendations or study designs and to support the approval of drugs for neonatal use by regulatory authorities. Recent examples include a proof-of-concept study to provide real-world (PK/PD) evidence for azlocillin in neonates and optimized neonatal dosing for teicoplanin based on PK modeling and simulation using RWD [Citation133,Citation134].
Physiologically-based PK model development can benefit from real-world datasets covering the physiology of the population of interest. Neonatal physiology drastically changes during the first weeks after birth. The predictive performance of PBPK models will increase if the neonatal population’s time-dependent (PNA-dependent) physiology is well captured in the model [Citation132]. As Zhou et al. highlighted the predictive performance of PBPK models for drugs undergoing metabolization by CYPs and renal clearance is reasonable in children one month and older [Citation135,Citation136]. For neonates, overall PBPK predictive performance needs improvement. Our research group currently works on a PBPK platform to predict drug disposition in asphyxiated neonates treated with TH (I-PREDICT project, Innovative physiology-based pharmacokinetic model to predict drug exposure in neonates undergoing cooling therapy, ) [Citation113,Citation128]. Real-world data of sCr, as a biomarker for the renal function of neonates with PA/TH, were pooled to generate sCr PNA profiles over time. Overall, sCr patterns over PNA were significantly higher in neonates with PA/TH than in controls [Citation11]. These data can reflect renal physiology and be used to estimate GFR in a PA PBPK platform to improve the prediction of renally cleared drug exposure.
Figure 2. Visual presentation of the I-PREDICT project, aiming to improve dosage recommendation for drugs in neonates with perinatal asphyxia treated with therapeutic hypothermia by developing a physiologically-based pharmacokinetic model, combining preclinical (in vitro and animal data) and clinical data. Pharmacokinetic predictions of four model drugs (midazolam, fentanyl, phenobarbital, and topiramate) are investigated. Created with BioRender.com.
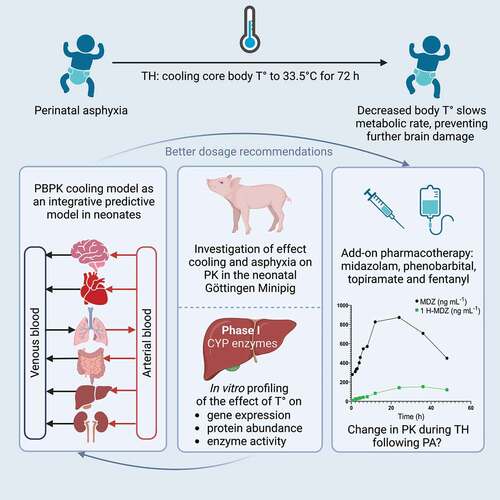
5.2. Challenges of real-world data research initiatives
Overall, the awareness and acceptance of real-world evidence are increasing among stakeholders (e.g. industry, regulators, clinicians, and patients). Real-world data has a promising role in improving pharmacotherapy. However, some challenges must be considered; compared to data collected during RCTs, RWD is usually unstructured and variable, requiring more advanced analytical methods/modeling and statistics for data analysis. Furthermore, concerns about the quality of the obtained data, privacy, and confidentiality may arise [Citation137]. Patients’ and parents’ perspectives are hereby of relevance. Recently, Sawtell et al. reported on the view of parents, adults born preterm, and professionals on the linkage of routinely collected RWD of preterm babies [Citation138]. It was considered a feasible and cost-efficient approach to gain insights into long-term outcomes and evaluate interventions in the neonatal period. The stakeholders all support data linkage, especially with opt-out consent. A minority, which is initially not supportive, becomes supportive if additional information is provided [Citation138]. Overall, we consider RWD an essential data source for developing PBPK models. Section 6 describes some consecutive steps in constructing a PBPK model for the neonatal population with PA/TH.
6. Physiologically-based pharmacokinetics (PBPK) as an integrative predictive model
6.1. Concept for development of a PBPK hypothermia model (I-PREDICT project)
As mentioned above, PBPK models are used for bottom-up (or middle-out) prediction of the PK of drugs and their metabolites in a predefined population [Citation139,Citation140]. Therefore, PBPK models are established with drug-dependent and system-dependent parameters on the relevant (patho)physiology. Drug-specific data include (1) physicochemical characteristics of the drug and its metabolites (e.g. lipophilicity, ionization behavior, and aqueous solubility); (2) parameters describing drug disposition pathways at the cellular and molecular level (e.g. membrane permeability, hepatic enzyme-mediated clearance, and plasma protein binding). Input data on the physiology includes organ weights, composition (water/lipids/proteins), subcompartmentalization (intracellular/interstitial/vascular), blood perfusion rates, and fluid exchange rates between vascular and interstitial/intracellular volumes. In addition, information on the specific pathology (e.g. diabetes, asphyxia, renal impairment) and/or treatment conditions can also be specified at the population level. Importantly, interindividual differences in these (patho)physiological data can also be included, allowing us to account for real-world inter-subject differences. Rapid and significant changes in ontogeny make PBPK models for the neonatal population challenging. Data on the impact of PA/TH (see previous sections) can then be integrated into such a PBPK model. Specifically for the neonatal population, adequate ‘scaling’ of physiological variables is crucial and essentially requires knowledge about the ontogeny of physiology and drug-metabolizing enzymes in the human neonatal population. This type of ‘rational’ extrapolation of adult to pediatric PBPK models has previously been illustrated for fentanyl and lorazepam [Citation141,Citation142]. The PBPK modeling study with fentanyl also covers the approach to be taken when multiple compounds are co-administered, i.e. when drug-drug interactions apply.
Recently, we reported on ongoing efforts to exploit PBPK models for predicting the impact of PA (‘disease’) and/or TH (‘treatment’) on the PK of model drugs in this vulnerable population [Citation113]. As clinical research in this setting is hampered by ethical, statistical, and technical challenges, the I-PREDICT project proposes a multidisciplinary approach to generate the input data required for establishing a PBPK framework for this setting (). In vivo studies in Göttingen minipigs with relevant model drugs (phenobarbital, midazolam, fentanyl, and topiramate) have been conducted to disentangle the impact of PA/TH on PK in a controlled in vivo setting () [Citation128]. In addition, in vitro experiments are ongoing to study the impact of reduced temperatures on enzyme kinetics, protein abundance and gene expression in the liver. The combined insights obtained with these non-clinical approaches will be incorporated into a conceptual PBPK framework for bottom-up prediction of in vivo PK under PA/TH conditions.
The following section will summarize the physiological parameters currently used to build human neonatal virtual subjects for PBPK modeling. Next, parameters likely influenced by temperature will be proposed, i.e. to inform a strategy for generating PBPK models for neonates with PA/TH.
6.2. Physiological parameters in available PBPK tools
The open-source PBPK platform PK-Sim and its available database were used to generate a structured overview of the anatomical and physiological parameters informing PBPK model building (version 10 – build 257). As described in the documentation of the Open systems Pharmacology platform PK-Sim, the ‘Individual’ building block specifies a virtual subject’s anatomical and physiological properties. This can be human or animal species [Citation143]. In the case of animal species, age-dependent scaling is performed proportionally for all organs in a linear manner based on total body weight. Human virtual subjects are composed of a literature database describing the impact of age, body weight, body mass index, and sex on anatomical and physiological features such as organ weights and blood perfusion rates. The database is used to build virtual subjects for starting values of age, weight, and height. This is achieved by modifying organ volumes, volume fractions (database yields global scale factor), and other parameters (e.g. hematocrit) for which (population) distribution data are available. For the human species, PK-Sim offers the choice between 8 different subpopulations (Asian, Japanese, Black, White and Mexican American, European, preterm, and pregnant populations). Male and female human growth models for the preterm population specify body weight in the function of PNA for gestational ages between 24 and 37 weeks. These preterm growth models merge with the growth curves of term infants at two years of PNA.
Of note, capillary surface areas (SA) are estimated based on two relatively simple approaches (1) based on the fraction of vascular space, the organ volume, and a proportionality constant, assuming similar vascular tree morphologies across organs; (2) based on organ blood flow. Supplementary Table B provides a structured summary of the most important parameters used to build PBPK models for male neonates six hours after birth, i.e. the ‘starting’ population in the context of PA/TH but excluding PA/TH itself. These data were obtained from the PK-Sim platform (version 10) and MoBi, specifying a male human subject ‘European (ICRP, 2002)’ and setting the age to 0.25 days (yielding a neonate of 3.50 kg, 51 cm). Reference data for this population have been reported previously [Citation144].
6.3. Pathophysiological changes to be explored during the development of PBPK models for perinatal asphyxia and therapeutic hypothermia
The underlined parameters in Supplementary Table B can be considered for modification when building a PBPK model for the neonatal PA/TH population. Such parameters include flow rates, GFR, and organ composition. Sensitivity analyses may inform prioritization for collecting pathophysiological data currently lacking for this specific condition/treatment. In addition, as described in more detail by Smits et al., the Göttingen minipig-based in vivo animal model for PA/TH is expected to further inform on non-maturational covariates [Citation113,Citation128]. Translational integration of these animal data into the human PBPK model will benefit from establishing a minipig PBPK model. Such a strategy has for instance already been applied to predict the PK of oseltamivir in a pediatric population [Citation145]. A particular challenge associated with this is the much more limited physiological database for minipigs compared to humans. On the other hand, the availability of in vivo PK data at higher resolution, is expected to at least support the development and evaluation of mechanistic hypotheses regarding the (separate) impact of PA and TH on PK. When building the human PBPK model, information obtained from the PA/TH animal model will be evaluated in combination with real world human data (e.g. renal function, albumin).
Importantly, the PA/TH PBPK modeling framework should also account for changes in intrinsic ADME properties related to disease and reduced temperature. In vitro studies exploring the impact of temperature on enzyme activity and gene expression of enzymes are currently ongoing [Citation146,Citation147]. Translational integration of these in vitro data in the PBPK models will need to consider adequate scaling factors for consistent in vitro to in vivo extrapolation. Quantitative proteomics measurements on the in vitro systems as well as on in vivo (liver) samples are ongoing and expected to support this translation endeavor. As also proposed in Smits et al., exploration (and possible determination) of biomarkers (e.g. 4β-hydroxycholesterol/6β-hydroxycortisol as surrogate for CYP3A activity) represents another opportunity to establish a representative PA/TH population in terms of hepatic protein abundance [Citation113]. In summary, carefully integrating the impact of temperature and/or PA on the physiological parameters and temperature-dependent intrinsic clearance is expected to enable a successful PBPK-based prediction strategy. A detailed workflow proposal has been described by Smits et al. [Citation113].
7. Discussion
Since the introduction of TH as a standard therapy, knowledge of PK in this patient population is exponentially increasing. However, knowledge gaps remain, e.g. underlying mechanisms of the impact of PA/TH on neonatal drug metabolism. To make progress in this research field, animal models are relevant since they allow uncoupling of the ‘phenotype = asphyxia +TH.’ In this preclinical space, the rodent (Rici-Vanucci carotid ligation model) and the neonatal pig model are most commonly used [Citation121,Citation148]. However, we should be aware of the limitations of approaches in simulating clinical hypoxia. For example, the rodent model does not have multi-organ failure compared to the pig model, so interspecies extrapolations need additional attention (section 4). As mentioned in Section 6.3, building a PBPK model for the Göttingen minipig with PA and/or TH is expected to be instrumental in this respect.
The 2-iminobiotin PK study by Favié et al. illustrates that extrapolation from the rat is insufficient in integrating the pathophysiology of the asphyxiated neonate undergoing TH (0.08 instead of the predicted 0.16 mg/kg every six hours results in targeted exposure). Therefore, this extrapolation may result in the overexposure of 2-iminobiotin in the subsequent phase 2 studies [Citation29]. Physiologically-based PK models are likely an effective tool to better integrate these different pieces of knowledge [Citation113]. It also allows learning from preclinical data and integrating preclinical and clinical observations to improve the predictive performance of PBPK models. Victor et al. concluded that in the search for new neuroprotective therapies in neonatal HIE and to progress these possible new therapies toward the clinical trial level, optimal preclinical (animal/juvenile) models, as well as industry support and dose-ranging studies with PK/PD modeling, are needed [Citation107]. Finally, optimal clinical trials for this orphan condition require well-designed protocols and multicenter collaboration to reach a sufficient sample size, avoid harmful exposures, and abandon therapies [Citation107].
8. Conclusion
This review summarizes the current knowledge of pathophysiology and the cellular and molecular mechanisms of the biochemical pathways affected by PA and TH. It identifies several knowledge gaps in this field. First, the available asphyxia pig models and their methodological considerations were covered since animal models are valuable tools for neonatal and pediatric drug development. To enable precision dosing in neonates with PA/TH, we proposed a multidisciplinary and translational PBPK approach using clinical and preclinical observations for model development and evaluation. Finally, the applicability and challenges in developing such a PBPK model and recommendations for future directions were provided.
9. Expert opinion
Although this review provides a detailed overview of currently available data in both the preclinical and clinical fields of relevance for PBPK development in the setting of PA/TH, we want to share some reflections for further research. First, from a molecular point of view, we found no information regarding the effect of Hsp expression on ADME properties. However, there is evidence that miRNA expression affects ADME-relevant protein expression (e.g. Rieger et al. [Citation149]). There is limited or no information on IEG expression and ADME-relevant protein expression and function. Additionally, fewer data and published studies are available regarding epigenetics in humans and other animals [Citation150]. This area of expertise is still largely unexplored and might be interesting to investigate further. Second, although this review mainly focuses on PK, data on PD in this population remains limited. This is not only the case in the setting of PA/TH, in general the collection of high-quality neonatal PD data is challenging. Advances in bedside neuromonitoring, neuroimaging tools, and laboratory biomarkers are promising to close this gap and link PK and PD observations [Citation105,Citation106]. Third, as neonatal clinical care evolved during the last decade, the clinical setting of TH at present differs from the setting during the first years after TH introduction. The ‘do’s’ and ‘don’ts’ for managing HIE during TH, as proposed in a recent care practice bundle by Wintermark et al., illustrate how guidance can be provided to healthcare providers in this setting [Citation151]. However, the effect of using such a care bundle on outcomes needs to be demonstrated in the future. At present, the application of less invasive respiratory support, tailored analgosedation resulting in more reactive patients, involvement of parents/family in daily care, dedicated nurses experienced in using the bedside equipment needed in this setting (e.g. cooling device, neuromonitoring expertise), palliative care, and specialized multidisciplinary follow-up after discharge are currently implemented in many centers. Researchers need to be aware of this evolution in clinical care and, consequently, the change of target population over time to reliably compare study results, including PK/PD findings.
A disease-specific PBPK model for asphyxiated newborns undergoing TH is currently unavailable but would be instrumental in streamlining drug dose and exposure. However, this is just one of the many challenges. As part of the PBPK framework under construction in the I-PREDICT project, the utility of promising biomarkers for the prediction of (hepatic) drug disposition is explored [Citation113]. Biomarker quantification (including 4β-hydroxycholesterol and 6β-hydroxycortisol as surrogate markers for CYP3A4 activity) in Göttingen minipigs and humans will be used to build additional confidence in bridging findings in Göttingen minipigs to humans. Moreover, the collection of such ADME-relevant biomarkers carries the promise to contribute to novel data regarding interindividual variability, for instance in metabolic activity in a very specific pediatric population. Subsequent refinement of the neonatal framework should also consider the impact of drug-drug interactions as well as pharmacogenetics as additional covariates [Citation152,Citation153]. However, the influence of a rapidly evolving ontogeny and associated developmental changes may overwhelm some of these other non-maturational covariates with a high impact in the adult population.
The specific setting to conduct clinical trials in this population (an orphan disease diagnosed very shortly after birth, unanticipated and no clustered care at diagnosis, the very short timelines to complete the consent process, uncertainties on relevant biomarkers for severity and outcome, uncertainties on how to model target exposure, the challenges, and limitations of the ‘commonly used’ RCTs), make these studies extremely challenging.
Consequently, to facilitate ongoing research, stimulate new drug development programs, and improve the impact of these activities, multistakeholder collaboration is warranted to streamline study flow and improve study design. This will bring together academia, clinical research networks, patients/parents, industry, and authorities, as has been recently reported for other settings like pediatric inflammatory bowel disease or pediatric oncology [Citation154,Citation155].
Article highlights
Therapeutic hypothermia (TH) represents the standard of care for neonates with moderate to severe perinatal asphyxia (PA) and hypoxic-ischemic encephalopathy (HIE). To support drug development for this population, knowledge from clinical observations (pharmacokinetic (PK) data and real-world data on physiology), preclinical (in vitro and in vivo animal model) data, and molecular and cellular biology insights can be incorporated into a physiologically-based PK (PBPK) model to serve as an integrative predictive modeling approach.
Using an exploratory search in PubMed (until November 2022), pathophysiological characteristics of system-specific functions in asphyxiated neonates were summarized, mainly referring to cardiac output, regional blood flow, renal function, plasma composition, or intestinal functions.
The current knowledge regarding cellular and molecular mechanisms of essential biochemical pathways affected by PA and/or TH is presented, with attention to pharmacologically relevant genes and proteins.
Animal models can provide useful information on mechanisms in human diseases, but also on exploring (patho)physiological factors affecting PK, safety, and efficacy of drugs. Currently available in vivo asphyxia pig models and the methodological considerations are also covered in this review.
By combining in vitro disposition research with in vivo animal and human data, the PBPK model development can be initiated using a multidisciplinary and translational approach. Physiologically-based PK modeling represents an established tool for drug development with a good track record, including regulatory acceptance. It represents an instrument for data integration, hypothesis testing, and knowledge generation. However, its use in neonatal pharmacotherapy is still limited.
Declaration of interest
The authors have no other relevant affiliations or financial involvement with any organization or entity with a financial interest in or financial conflict with the subject matter or materials discussed in the manuscript apart from those disclosed.
Reviewer disclosures
Peer reviewers on this manuscript have no relevant financial or other relationships to disclose.
Additional information
Funding
References
- Davidson JO, Gonzalez F, Gressens P, et al. Update on mechanisms of the pathophysiology of neonatal encephalopathy. Semin Fetal Neonatal Med. 2021 Oct;26(5):101267.
- WHO. Levels and trends in child mortality report, News Room, Fact-sheets2021 [cited 2023 May 5]. Fact-sheets2021 https://www.who.int/news-room/fact-sheets/detail/levels-and-trends-in-child-mortality-report-2021
- Michniewicz B, Szpecht D, Sowińska A, et al. Biomarkers in newborns with hypoxic-ischemic encephalopathy treated with therapeutic hypothermia. Childs Nerv Syst. 2020 Dec;36(12):2981–2988.
- Abate BB, Bimerew M, Gebremichael B, et al. Effects of therapeutic hypothermia on death among asphyxiated neonates with hypoxic-ischemic encephalopathy: A systematic review and meta-analysis of randomized control trials. Plos One. 2021;16(2):e0247229. doi: 10.1371/journal.pone.0247229
- Sun YJ, Zhang ZY, Fan B, et al. Neuroprotection by Therapeutic Hypothermia. Front Neurosci. 2019;13:586. doi: 10.3389/fnins.2019.00586
- O’Dea M, Sweetman D, Bonifacio SL, et al. Management of multi organ dysfunction in neonatal encephalopathy. Front Pediatr. 2020;8:239. doi: 10.3389/fped.2020.00239
- Nestaas E, Walsh BH. Hypothermia and Cardiovascular Instability. Clin Perinatol. 2020 Sep;47(3):575–592. doi: 10.1016/j.clp.2020.05.012
- Nestaas E, Skranes JH, Støylen A, et al. The myocardial function during and after whole-body therapeutic hypothermia for hypoxic-ischemic encephalopathy, a cohort study. Early Hum Dev. 2014 May;90(5):247–252.
- van Wincoop M, de Bijl-Marcus K, Lilien M, et al. Effect of therapeutic hypothermia on renal and myocardial function in asphyxiated (near) term neonates: A systematic review and meta-analysis. Plos One. 2021;16(2):e0247403. doi: 10.1371/journal.pone.0247403
- Allegaert K, Salaets T, Ward RM, et al. Qtc intervals are prolonged in late preterm and term neonates during therapeutic hypothermia but normalize afterwards. Children (Basel). 2021 Dec 8;8(12):1153.
- Keles E, Wintermark P, Groenendaal F, et al. Serum creatinine patterns in neonates treated with therapeutic hypothermia for neonatal encephalopathy. Neonatology. 2022;119(6):686–694. doi: 10.1159/000525574
- Krzyzanski W, Smits A, Van Den Anker J, et al. Population model of serum creatinine as time-dependent covariate in neonates. Aaps J. 2021 Jun 17;23(4):86.
- Brucknerová I, Ujházy E, Dubovický M, et al. Early assessment of the severity of asphyxia in term newborns using parameters of blood count. Interdiscip Toxicol. 2008 Dec;1(3–4):211–213.
- Muniraman H, Gardner D, Skinner J, et al. Biomarkers of hepatic injury and function in neonatal hypoxic ischemic encephalopathy and with therapeutic hypothermia. Eur J Pediatr. 2017 Oct;176(10):1295–1303.
- Groenendaal F, De Vooght KM, van Bel F. Blood gas values during hypothermia in asphyxiated term neonates. Pediatrics. 2009 Jan;123(1):170–172. doi: 10.1542/peds.2008-1955
- Van Anh TN, Hao TK, Chi NTD, et al. Predictions of hypoxic-ischemic encephalopathy by umbilical cord blood lactate in newborns with birth asphyxia. Open Access Maced J Med Sci. 2019;7(21):3564–3567. doi: 10.3889/oamjms.2019.581
- Beach RC, Menzies IS, Clayden GS, et al. Gastrointestinal permeability changes in the preterm neonate. Arch Dis Child. 1982 Feb;57(2):141–145.
- Hermans T, Carkeek K, Dereymaeker A, et al. Assessing neurovascular coupling using wavelet coherence in neonates with asphyxia. Adv Exp Med Biol. 2022;1395:183–187.
- Zhou KQ, McDouall A, Drury PP, et al. Treating Seizures after hypoxic-ischemic encephalopathy—Current controversies and future directions. IJMS. 2021 Jul 1;22(13):7121.
- Gebauer CM, Knuepfer M, Robel-Tillig E, et al. Hemodynamics among neonates with hypoxic-ischemic encephalopathy during whole-body hypothermia and passive rewarming. Pediatrics. 2006 Mar;117(3):843–850.
- Sehgal A, Wong F, Mehta S. Reduced cardiac output and its correlation with coronary blood flow and troponin in asphyxiated infants treated with therapeutic hypothermia. Eur J Pediatr. 2012 Oct;171(10):1511–1517. doi: 10.1007/s00431-012-1764-y
- Sakhuja P, More K, Ting JY, et al. Gastrointestinal hemodynamic changes during therapeutic hypothermia and after rewarming in neonatal hypoxic-Ischemic encephalopathy. Pediatr Neonatol. 2019 Dec;60(6):669–675.
- Favié LMA, Groenendaal F, van den Broek MPH, et al. Phenobarbital, midazolam pharmacokinetics, effectiveness, and drug-drug interaction in asphyxiated neonates undergoing therapeutic hypothermia. Neonatology. 2019;116(2):154–162. doi: 10.1159/000499330
- Favié LMA, de Haan TR, Bijleveld YA, et al. Prediction of drug exposure in critically Ill encephalopathic neonates treated with therapeutic hypothermia based on a pooled population pharmacokinetic analysis of seven drugs and five metabolites. Clin Pharmacol Ther. 2020 Nov;108(5):1098–1106. doi: 10.1002/cpt.1917
- Allegaert K, van den Anker J. Dose-related adverse drug events in neonates: Recognition and assessment. J Clin Pharmacol. 2021 Jun;61(Suppl 1):S152–s160. doi: 10.1002/jcph.1827
- Dani C, Poggi C, Fancelli C, et al. Changes in bilirubin in infants with hypoxic-ischemic encephalopathy. Eur J Pediatr. 2018 Dec;177(12):1795–1801.
- Annink KV, Franz AR, Derks JB, et al. Allopurinol: Old drug, new indication in neonates? Curr Pharm Des. 2017;23(38):5935–5942. doi: 10.2174/1381612823666170918123307
- Maiwald CA, Annink KV, Rüdiger M, et al. Effect of allopurinol in addition to hypothermia treatment in neonates for hypoxic-ischemic brain injury on neurocognitive outcome (ALBINO): study protocol of a blinded randomized placebo-controlled parallel group multicenter trial for superiority (phase III). BMC Pediatr. 2019 Jun 27;19(1):210.
- Favié LMA, Peeters-Scholte C, Bakker A, et al. Pharmacokinetics and short-term safety of the selective NOS inhibitor 2-iminobiotin in asphyxiated neonates treated with therapeutic hypothermia. Pediatr Res. 2020 Mar;87(4):689–696.
- Wu YW, Comstock BA, Gonzalez FF, et al. Trial of erythropoietin for hypoxic-ischemic encephalopathy in newborns. N Engl J Med. 2022 Jul 14;387(2):148–159.
- Allen KA, Brandon DH. Hypoxic ischemic encephalopathy: Pathophysiology and experimental treatments. Newborn Infant Nurs Rev. 2011 Sep 1;11(3):125–133.
- Yenari MA, Han HS. Neuroprotective mechanisms of hypothermia in brain ischaemia. Nat Rev Neurosci. 2012 Feb 22;13(4):267–278.
- Kurisu K, Kim JY, You J, et al. Therapeutic hypothermia and neuroprotection in acute neurological disease. Curr Med Chem. 2019;26(29):5430–5455. doi: 10.2174/0929867326666190506124836
- Jin JS, Sakaeda T, Kakumoto M, et al. Effect of therapeutic moderate hypothermia on multi-drug resistance protein 1-mediated transepithelial transport of drugs. Neurol Med Chir (Tokyo). 2006 Jul;46(7):321–327. discussion 327. doi: 10.2176/nmc.46.321
- Kamme F, Campbell K, Wieloch T. Biphasic expression of the fos and jun families of transcription factors following transient forebrain ischaemia in the rat. Effect of hypothermia. Eur J Neurosci. 1995 Oct 1;7(10):2007–2016.
- Akaji K, Suga S, Fujino T, et al. Effect of intra-ischemic hypothermia on the expression of c-Fos and c-Jun, and DNA binding activity of AP-1 after focal cerebral ischemia in rat brain. Brain Res. 2003 Jun 13;975(1–2):149–157.
- Kobayashi MS, Asai S, Ishikawa K, et al. Global profiling of influence of intra-ischemic brain temperature on gene expression in rat brain. Brain Res Rev. 2008 Jun;58(1):171–191.
- Vemuganti R. The MicroRNAs and Stroke: No need to be coded to be counted. Transl Stroke Res. 2010 Sep 1;1(3):158–160.
- Truettner JS, Alonso OF, Bramlett HM, et al. Therapeutic hypothermia alters microRNA responses to traumatic brain injury in rats. J Cereb Blood Flow Metab. 2011 Sep;31(9):1897–1907.
- Looney AM, Walsh BH, Moloney G, et al. Downregulation of umbilical cord blood levels of miR-374a in neonatal hypoxic ischemic encephalopathy. J Pediatr. 2015 Aug;167(2):269–73.e2.
- Winkler I, Heisinger T, Hammerl M, et al. MicroRNA expression profiles as diagnostic and prognostic biomarkers of perinatal asphyxia and hypoxic-ischaemic encephalopathy. Neonatology. 2022;119(2):204–213. doi: 10.1159/000521356
- O’Sullivan MP, Looney AM, Moloney GM, et al. Validation of altered umbilical cord blood MicroRNA expression in neonatal hypoxic-ischemic encephalopathy. JAMA Neurol. 2019 Mar 1;76(3):333–341.
- Fujita J. Cold shock response in mammalian cells. J Mol Microbiol Biotechnol. 1999 Nov;1(2):243–255.
- Sonna LA, Fujita J, Gaffin SL, et al. Invited review: Effects of heat and cold stress on mammalian gene expression. J Appl Physiol. 1985 Apr;92(4):1725–1742.
- Seo YM, Hwang-Bo S, Im SA, et al. Predictive value of heat-shock protein gene expression on severe neonatal hypoxic-ischemic encephalopathy. Diagnostics. 2022 Apr 13;12(4):981.
- Oh JS, Park J, Kim K, et al. HSP70-mediated neuroprotection by combined treatment of valproic acid with hypothermia in a rat asphyxial cardiac arrest model. Plos One. 2021;16(6):e0253328. doi: 10.1371/journal.pone.0253328
- Matz JM, Blake MJ, Tatelman HM, et al. Characterization and regulation of cold-induced heat shock protein expression in mouse brown adipose tissue. Am J Physiol. 1995 Jul;269(1 Pt 2):R38–47.
- Kumar K, Wu X, Evans AT, et al. The effect of hypothermia on induction of heat shock protein (HSP)-72 in ischemic brain. Metab Brain Dis. 1995 Dec;10(4):283–291.
- Lindquist JA, Mertens PR. Cold shock proteins: from cellular mechanisms to pathophysiology and disease. Cell Commun Signal. 2018 Sep 26;16(1):63.
- Heinemann U, Roske Y. Cold-shock domains—Abundance, structure, properties, and nucleic-acid binding. Cancers (Basel). 2021 Jan 7;13(2):190.
- Alam HB, Hashmi S, Frankelstein RA, et al. Alterations in gene expression after induction of profound hypothermia for the treatment of lethal hemorrhage. J Trauma Acute Care Surg. 2010;68(5):1084–1098. doi: 10.1097/TA.0b013e3181d76bd1
- Patel J, McLeod LE, Vries RG, et al. Cellular stresses profoundly inhibit protein synthesis and modulate the states of phosphorylation of multiple translation factors. Eur J Biochem. 2002 Jun;269(12):3076–3085.
- Hu BR, Wieloch T. Stress-induced inhibition of protein synthesis initiation: modulation of initiation factor 2 and guanine nucleotide exchange factor activities following transient cerebral ischemia in the rat. J Neurosci. 1993 May;13(5):1830–1838. doi: 10.1523/JNEUROSCI.13-05-01830.1993
- Sonna LA, Kuhlmeier MM, Carter HC, et al. Effect of moderate hypothermia on gene expression by THP-1 cells: a DNA microarray study. Physiol Genomics. 2006 Jun 16;26(1):91–98.
- Zak RB, Shute RJ, Heesch MW, et al. Impact of hot and cold exposure on human skeletal muscle gene expression. Appl Physiol Nutr Metab. 2017 Mar;42(3):319–325.
- Faraji S, Rashki Ghaleno L, Sharafi M, et al. Gene expression alteration of sperm-Associated antigens in human cryopreserved sperm. Biopreserv Biobank. 2021 Dec;19(6):503–510.
- Shaw L, Sneddon SF, Brison DR, et al. Comparison of gene expression in fresh and frozen-thawed human preimplantation embryos. Reproduction. 2012 Nov;144(5):569–582.
- Tabuchi Y, Furusawa Y, Kariya A, et al. Common gene expression patterns responsive to mild temperature hyperthermia in normal human fibroblastic cells. Int J Hyperthermia. 2013;29(1):38–50. doi: 10.3109/02656736.2012.753163
- Ito A, Aoyama T, Iijima H, et al. Culture temperature affects redifferentiation and cartilaginous extracellular matrix formation in dedifferentiated human chondrocytes. J Orthop Res. 2015 May;33(5):633–639.
- Farashi S, Sharifi E. Stem cell behavior at hypothermia: A review article. Curr Stem Cell Res Ther. 2021;16(6):718–729. doi: 10.2174/1574888X16666201229124842
- Fairchild KD, Singh IS, Carter HC, et al. Hypothermia enhances phosphorylation of I{kappa}B kinase and prolongs nuclear localization of NF-{kappa}B in lipopolysaccharide-activated macrophages. Am J Physiol Cell Physiol. 2005 Nov;289(5):C1114–21.
- Maruyama T, Kusakari S, Sato-Hashimoto M, et al. Hypothermia-induced tyrosine phosphorylation of SIRPα in the brain. J Neurochem. 2012;121(6):891–902. doi: 10.1111/j.1471-4159.2012.07748.x
- Bretteville A, Marcouiller F, Julien C, et al. Hypothermia-induced hyperphosphorylation: a new model to study tau kinase inhibitors. Sci Rep. 2012;2(1):480. doi: 10.1038/srep00480
- Zhao S, Xiao P, Cui H, et al. Hypothermia-induced ubiquitination of voltage-dependent anion channel 3 protects BV2 microglia cells from cytotoxicity following oxygen-glucose deprivation/recovery. Front Mol Neurosci. 2020;13:100. doi: 10.3389/fnmol.2020.00100
- Bogert NV, Werner I, Kornberger A, et al. Influence of hypothermia and subsequent rewarming upon leukocyte-endothelial interactions and expression of Junctional-Adhesion-Molecules a and B. Sci Rep. 2016 Feb 25;6(1):21996.
- Yenari MA, Han HS. Influence of hypothermia on post-ischemic inflammation: role of nuclear factor kappa B (NFkappaB). Neurochem Int. 2006 Jul;49(2):164–169. doi: 10.1016/j.neuint.2006.03.016
- Fairchild KD, Singh IS, Patel S, et al. Hypothermia prolongs activation of NF-kappaB and augments generation of inflammatory cytokines. Am J Physiol Cell Physiol. 2004 Aug;287(2):C422–31.
- Shan Q, Ma F, Wei J, et al. Physiological functions of heat shock proteins. Curr Protein Pept Sci. 2020;21(8):751–760. doi: 10.2174/1389203720666191111113726
- Maffucci I, Laage D, Sterpone F, et al. Thermal adaptation of enzymes: Impacts of conformational shifts on catalytic activation energy and optimum temperature. Chemistry. 2020 Aug 6;26(44):10045–10056.
- Somero G. Temperature and proteins: Little things can mean a lot. Physiology. 1996;11(2):72–77. doi: 10.1152/physiologyonline.1996.11.2.72
- Davidovic M, Mattea C, Qvist J, et al. Protein cold denaturation as seen from the solvent. J Am Chem Soc. 2009 Jan 28;131(3):1025–1036.
- Almeida VM, Marana SR, Lau ATY. Optimum temperature may be a misleading parameter in enzyme characterization and application. Plos One. 2019;14(2):e0212977. doi: 10.1371/journal.pone.0212977
- Arcus VL, Prentice EJ, Hobbs JK, et al. On the temperature dependence of enzyme-catalyzed rates. Biochemistry. 2016 Mar 29;55(12):1681–1688.
- Wojcik M, Miłek J. A new method to determine optimum temperature and activation energies for enzymatic reactions. Bioprocess Biosyst Eng. 2016 Aug;39(8):1319–1323. doi: 10.1007/s00449-016-1596-7
- Manikandan P, Nagini S. Cytochrome P450 structure, function and clinical significance: A review. Curr Drug Targets. 2018;19(1):38–54. doi: 10.2174/1389450118666170125144557
- Otyepka M, Skopalík J, Anzenbacherová E, et al. What common structural features and variations of mammalian P450s are known to date? Biochim Biophys Acta. 2007 Mar;1770(3):376–389.
- Tilton RF Jr., Dewan JC, Petsko GA. Effects of temperature on protein structure and dynamics: X-ray crystallographic studies of the protein ribonuclease-A at nine different temperatures from 98 to 320 K. Biochemistry. 1992 Mar 10;31(9):2469–2481.
- Zhou J, Poloyac SM. The effect of therapeutic hypothermia on drug metabolism and response: cellular mechanisms to organ function. Expert Opin Drug Metab Toxicol. 2011 Jul;7(7):803–816. doi: 10.1517/17425255.2011.574127
- Somero GN. Proteins and temperature. Annu Rev Physiol. 1995;57(1):43–68. doi: 10.1146/annurev.ph.57.030195.000355
- Cojocaru V, Winn PJ, Wade RC. The ins and outs of cytochrome P450s. Biochim Biophys Acta. 2007 Mar;1770(3):390–401. doi: 10.1016/j.bbagen.2006.07.005
- Diaz D, Fabre I, Daujat M, et al. Omeprazole is an aryl hydrocarbon-like inducer of human hepatic cytochrome P450. Gastroenterology. 1990 Sep;99(3):737–747.
- Renwick AB, Watts PS, Edwards RJ, et al. Differential maintenance of cytochrome P450 enzymes in cultured precision-cut human liver slices. Drug Metab Dispos. 2000 Oct;28(10):1202–1209.
- Faber MS, Fuhr U. Time response of cytochrome P450 1A2 activity on cessation of heavy smoking. Clin Pharmacol Ther. 2004 Aug;76(2):178–184. doi: 10.1016/j.clpt.2004.04.003
- Magnusson MO, Dahl ML, Cederberg J, et al. Pharmacodynamics of carbamazepine-mediated induction of CYP3A4, CYP1A2, and Pgp as assessed by probe substrates midazolam, caffeine, and digoxin. Clin Pharmacol Ther. 2008 Jul;84(1):52–62.
- Liston HL, DeVane CL, Boulton DW, et al. Differential time course of cytochrome P450 2D6 enzyme inhibition by fluoxetine, sertraline, and paroxetine in healthy volunteers. J Clin Psychopharmacol. 2002 Apr;22(2):169–173.
- Venkatakrishnan K, Obach RS. In vitro-in vivo extrapolation of CYP2D6 inactivation by paroxetine: prediction of nonstationary pharmacokinetics and drug interaction magnitude. Drug Metab Dispos. 2005 Jun;33(6):845–852. doi: 10.1124/dmd.105.004077
- Lucas D, Ménez C, Girre C, et al. Decrease in cytochrome P4502E1 as assessed by the rate of chlorzoxazone hydroxylation in alcoholics during the withdrawal phase. Alcohol Clin Exp Res. 1995 Apr;19(2):362–366.
- Emery MG, Jubert C, Thummel KE, et al. Duration of cytochrome P-450 2E1 (CYP2E1) inhibition and estimation of functional CYP2E1 enzyme half-life after single-dose disulfiram administration in humans. J Pharmacol Exp Ther. 1999 Oct;291(1):213–219.
- Pichard L, Fabre I, Daujat M, et al. Effect of corticosteroids on the expression of cytochromes P450 and on cyclosporin a oxidase activity in primary cultures of human hepatocytes. Mol Pharmacol. 1992 Jun;41(6):1047–1055.
- Levy RH, Pitlick WH, Troupin AS, and Green JR. The Effects of Disease States on Drug Pharmacokinetics. In: Benet, L. ed. Academy of Pharmaceutical Sciences. Washington DC; 1976. pp. 87-95.
- Lai AA, Levy RH, Cutler RE. Time-course of interaction between carbamazepine and clonazepam in normal man. Clin Pharmacol Ther. 1978 Sep;24(3):316–323. doi: 10.1002/cpt1978243316
- Warren JW Jr., Benmaman JD, Wannamaker BB, et al. Kinetics of a carbamazepine-ethosuximide interaction. Clin Pharmacol Ther. 1980 Nov;28(5):646–651.
- Fromm MF, Busse D, Kroemer HK, et al. Differential induction of prehepatic and hepatic metabolism of verapamil by rifampin. Hepatology. 1996 Oct;24(4):796–801.
- Rostami-Hodjegan A, Wolff K, Hay AW, et al. Population pharmacokinetics of methadone in opiate users: characterization of time-dependent changes. Br J Clin Pharmacol. 1999 Jul;48(1):43–52.
- Hsu A, Granneman GR, Witt G, et al. Multiple-dose pharmacokinetics of ritonavir in human immunodeficiency virus-infected subjects. Antimicrob Agents Chemother. 1997 May;41(5):898–905.
- von Bahr C, Steiner E, Koike Y, et al. Time course of enzyme induction in humans: effect of pentobarbital on nortriptyline metabolism. Clin Pharmacol Ther. 1998 Jul;64(1):18–26.
- Yang J, Liao M, Shou M, et al. Cytochrome p450 turnover: regulation of synthesis and degradation, methods for determining rates, and implications for the prediction of drug interactions. Curr Drug Metab. 2008 Jun;9(5):384–394. doi: 10.2174/138920008784746382
- van Groen BD, Nicolaï J, Kuik AC, et al. Ontogeny of hepatic transporters and drug-metabolizing enzymes in humans and in nonclinical species. Pharmacol Rev. 2021 Apr;73(2):597–678.
- Mužić T, Tounsi F, Madsen SB, et al. Melting transitions in biomembranes. Biochim Biophys Acta Biomembr. 2019 Nov 1;1861(11):183026. doi: 10.1016/j.bbamem.2019.07.014
- Baylon JL, Lenov IL, Sligar SG, et al. Characterizing the membrane-bound state of cytochrome P450 3A4: structure, depth of insertion, and orientation. J Am Chem Soc. 2013 Jun 12;135(23):8542–8551. doi: 10.1021/ja4003525
- Sinensky M. Homeoviscous adaptation–a homeostatic process that regulates the viscosity of membrane lipids in Escherichia coli. Proc Natl Acad Sci U S A. 1974 Feb;71(2):522–525. doi: 10.1073/pnas.71.2.522
- Anderson RL, Minton KW, Li GC, et al. Temperature-induced homeoviscous adaptation of Chinese hamster ovary cells. Biochim Biophys Acta. 1981 Mar 6;641(2):334–348. doi: 10.1016/0005-2736(81)90490-9
- Macdonald AG. The homeoviscous theory of adaptation applied to excitable membranes: a critical evaluation. Biochim Biophys Acta. 1990 Oct 8;1031(3):291–310. doi: 10.1016/0304-4157(90)90014-4
- Sojcic Z, Toplak H, Zuehlke R, et al. Cultured human skin fibroblasts modify their plasma membrane lipid composition and fluidity according to growth temperature suggesting homeoviscous adaptation at hypothermic (30 degrees C) but not at hyperthermic (40 degrees C) temperatures. Biochim Biophys Acta. 1992 Feb 17;1104(1):31–37. doi: 10.1016/0005-2736(92)90128-9
- Chalak L, Hellstrom-Westas L, Bonifacio S, et al. Bedside and laboratory neuromonitoring in neonatal encephalopathy. Semin Fetal Neonatal Med. 2021 Oct;26(5):101273.
- Wisnowski JL, Wintermark P, Bonifacio SL, et al. Neuroimaging in the term newborn with neonatal encephalopathy. Semin Fetal Neonatal Med. 2021 Oct;26(5):101304.
- Victor S, Rocha-Ferreira E, Rahim A, et al. New possibilities for neuroprotection in neonatal hypoxic-ischemic encephalopathy. Eur J Pediatr. 2022 Mar;181(3):875–887.
- Lutz IC, Allegaert K, de Hoon JN, et al. Pharmacokinetics during therapeutic hypothermia for neonatal hypoxic ischaemic encephalopathy: a literature review. BMJ Paediatr Open. 2020;4(1):e000685. doi: 10.1136/bmjpo-2020-000685
- Deferm N, Annink KV, Faelens R, et al. Glomerular filtration rate in asphyxiated neonates under therapeutic whole-body hypothermia, quantified by mannitol clearance. Clin Pharmacokinet. 2021 Jul;60(7):897–906.
- Cristea S, Smits A, Kulo A, et al. Amikacin pharmacokinetics to optimize dosing in neonates with perinatal asphyxia treated with hypothermia. Antimicrob Agents Chemother. 2017 Dec;61(12). doi: 10.1128/AAC.01282-17
- Bijleveld YA, de Haan TR, van der Lee HJ, et al. Altered gentamicin pharmacokinetics in term neonates undergoing controlled hypothermia. Br J Clin Pharmacol. 2016 Jun;81(6):1067–1077.
- Frymoyer A, Lee S, Bonifacio SL, et al. Every 36-h gentamicin dosing in neonates with hypoxic-ischemic encephalopathy receiving hypothermia. J Perinatol. 2013 Oct;33(10):778–782.
- Smits A, Annaert P, Van Cruchten S, et al. A physiology-based pharmacokinetic framework to support drug development and dose precision during therapeutic hypothermia in neonates [Review]. Front Pharmacol. 2020 2020 May 13; 11:11. doi: 10.3389/fphar.2020.00587
- Weerink MAS, Struys M, Hannivoort LN, et al. Clinical pharmacokinetics and pharmacodynamics of dexmedetomidine. Clin Pharmacokinet. 2017 Aug;56(8):893–913.
- O’Mara K, Weiss MD. Dexmedetomidine for sedation of neonates with HIE undergoing therapeutic hypothermia: A single-center experience. AJP Rep. 2018 Jul;8(3):e168–e173. doi: 10.1055/s-0038-1669938
- Elliott M, Burnsed J, Heinan K, et al. Effect of dexmedetomidine on heart rate in neonates with hypoxic ischemic encephalopathy undergoing therapeutic hypothermia. J Neonatal Perinatal Med. 2022;15(1):47–54. doi: 10.3233/NPM-210737
- McAdams RM, Pak D, Lalovic B, et al. Dexmedetomidine pharmacokinetics in neonates with hypoxic-ischemic encephalopathy receiving hypothermia. Anesthesiol Res Pract. 2020;2020:1–15. doi: 10.1155/2020/2582965
- Naveed M, Bondi DS, Shah PA. Dexmedetomidine versus fentanyl for neonates with hypoxic ischemic encephalopathy undergoing therapeutic hypothermia. J Pediatr Pharmacol Ther. 2022;27(4):352–357. doi: 10.5863/1551-6776-27.4.352
- Bäcke P, Bruschettini M, Sibrecht G, et al. Pharmacological interventions for pain and sedation management in newborn infants undergoing therapeutic hypothermia. Cochrane Database Syst Rev. 2022 Nov 10;11(11):Cd015023.
- Baserga M, DuPont TL, Ostrander B, et al. Dexmedetomidine use in infants undergoing cooling due to neonatal encephalopathy (DICE Trial): A randomized controlled trial: Background, aims and study protocol. Front Pain Res (Lausanne). 2021;2:770511. doi: 10.3389/fpain.2021.770511
- Ayuso M, Buyssens L, Stroe M, et al. The neonatal and juvenile pig in pediatric drug discovery and development. Pharmaceutics. 2021;13(1):44. doi: 10.3390/pharmaceutics13010044
- Mallard C, Vexler ZS. Modeling ischemia in the immature brain. Stroke. 2015;46(10):3006–3011. doi: 10.1161/STROKEAHA.115.007776
- van Dijk AJ, van Loon JPAM, Taverne MAM, et al. Umbilical cord clamping in term piglets: A useful model to study perinatal asphyxia? Theriogenology. 2008 2008 Sep 1;70(4):662–674. doi: 10.1016/j.theriogenology.2008.04.044
- Cheung P-Y, Gill RS, Bigam DL. A swine model of neonatal asphyxia. J Vis Exp. 2011;56(56):3166. doi: 10.3791/3166-v
- O’Brien CE, Santos PT, Kulikowicz E, et al. Hypoxia-ischemia and hypothermia independently and interactively affect neuronal pathology in neonatal piglets with short-term recovery. Dev Neurosci. 2019;41(1–2):17–33. doi: 10.1159/000496602
- Kyng KJ, Skajaa T, Kerrn-Jespersen S, et al. A piglet model of neonatal hypoxic-ischemic encephalopathy. J Vis Exp. 2015;99(99):e52454–e52454. doi: 10.3791/52454
- Ezzati M, Broad K, Kawano G, et al. Pharmacokinetics of dexmedetomidine combined with therapeutic hypothermia in a piglet asphyxia model. Acta Anaesthesiol Scand. 2014 Jul;58(6):733–742.
- Stroe M-S, Van Bockstal L, Valenzuela AP, et al. Development of a neonatal Göttingen minipig model for dose precision in perinatal asphyxia: technical opportunities, challenges, and potential further steps [Methods]. Front Pediatr. 2023;11:662.
- Robertson NJ, Faulkner S, Fleiss B, et al. Melatonin augments hypothermic neuroprotection in a perinatal asphyxia model. Brain. 2012;136(1):90–105. doi: 10.1093/brain/aws285
- Whitaker EE, Zheng CZ, Bissonnette B, et al. Use of a piglet model for the study of anesthetic-induced developmental neurotoxicity (AIDN): A translational neuroscience approach. J Vis Exp. 2017 Jun;11(124). doi: 10.3791/55193-v
- McMahon AW, Dal Pan G. Assessing drug safety in children - the role of real-world data. N Engl J Med. 2018 Jun 7;378(23):2155–2157.
- Smits A, De Cock P, Vermeulen A, et al. Physiologically based pharmacokinetic (PBPK) modeling and simulation in neonatal drug development: how clinicians can contribute. Expert Opin Drug Metab Toxicol. 2019 Jan;15(1):25–34.
- Wu YE, Wang T, Yang HL, et al. Population pharmacokinetics and dosing optimization of azlocillin in neonates with early-onset sepsis: a real-world study. J Antimicrob Chemother. 2021 Feb 11;76(3):699–709.
- Yamada T, Emoto C, Fukuda T, et al. Optimal teicoplanin dosing regimen in neonates and children developed by leveraging real-world clinical information. Ther Drug Monit. 2022 Jun 1;44(3):404–413.
- Zhou W, Johnson TN, Xu H, et al. Predictive performance of physiologically based pharmacokinetic and population pharmacokinetic modeling of renally cleared drugs in children. CPT Pharmacometrics Syst Pharmacol. 2016 Sep;5(9):475–483. doi: 10.1002/psp4.12101
- Zhou W, Johnson TN, Bui KH, et al. Predictive performance of physiologically based pharmacokinetic (PBPK) modeling of drugs extensively metabolized by major cytochrome P450s in children. Clin Pharmacol Ther. 2018 Jul;104(1):188–200. doi: 10.1002/cpt.905
- Dang A. Real-world evidence: A primer. Pharmaceut Med. 2023 Jan;37(1):25–36. doi: 10.1007/s40290-022-00456-6
- Sawtell M, van Blankenstein E, Bilal T, et al. Views of parents, adults born preterm and professionals on linkage of real-world data of preterm babies. Arch Dis Child Fetal Neonatal Ed. 2022 Oct 19;108(2):194–199. doi: 10.1136/archdischild-2022-324272
- Lin W, Chen Y, Unadkat JD, et al. Applications, challenges, and outlook for PBPK modeling and simulation: A regulatory, industrial and academic perspective. Pharm Res. 2022 Aug;39(8):1701–1731.
- Loisios-Konstantinidis I, Dressman J. Physiologically based pharmacokinetic/pharmacodynamic modeling to support waivers of in vivo clinical studies: Current status, challenges, and opportunities. Mol Pharm. 2021 Jan 4;18(1):1–17.
- Kovar L, Weber A, Zemlin M, et al. Physiologically-based pharmacokinetic (PBPK) modeling providing insights into fentanyl pharmacokinetics in adults and pediatric patients. Pharmaceutics. 2020 Sep 23;12(10):908.
- Maharaj AR, Barrett JS, Edginton AN. A workflow example of PBPK modeling to support pediatric research and development: Case study with lorazepam.Aaps J. 2013 2013 Apr 1;15(2):455–464. doi: 10.1208/s12248-013-9451-0
- Hindmarsh DRR AC, Serban R, Woodward CS, et al. The open systems pharmacology suite: Lawrence Livermore national laboratory; 2002-2021 [cited Jan 2023]. 10.02.23 11 [ Available from: https://docs.open-systems-pharmacology.org
- Basic anatomical and physiological data for use in radiological protection: reference values. A report of age- and gender-related differences in the anatomical and physiological characteristics of reference individuals. ICRP Publication 89. Ann ICRP. 2002;32(3–4):5–265. doi: 10.1016/S0146-6453(03)00002-2
- Parrott N, Davies B, Hoffmann G, et al. Development of a physiologically based model for oseltamivir and simulation of pharmacokinetics in neonates and infants. Clin Pharmacokinet. 2011 Sep;50(9):613–623.
- Leys K, Smits A, Allegaert K, et al. In vitro investigation of pharmacokinetics in neonates undergoing therapeutic hypothermia. In 50th Annual Conference of the European Teratology Society; 2022 25-28 Sep 2022; Antwerp, Belgium.
- Stroe M-S, Van Bockstal L, Valenzuela AP, et al., The neonatal Göttingen Minipig as translational model for drug disposition in perinatal asphyxia. In 50th Annual Conference of the European Teratology Society; 2022 25-28 Sep 2022; Antwerp, Belgium.
- Campion S, Inselman A, Hayes B, et al. The benefits, limitations and opportunities of preclinical models for neonatal drug development. Dis Model Mech. 2022 Apr 1;15(4). doi: 10.1242/dmm.049065
- Rieger JK, Klein K, Winter S, et al. Expression variability of absorption, distribution, metabolism, excretion-related microRnas in human liver: influence of nongenetic factors and association with gene expression. Drug Metab Dispos. 2013 Oct;41(10):1752–1762.
- McCaw BA, Stevenson TJ, Lancaster LT. Epigenetic responses to temperature and climate. Integr Comp Biol. 2020 Dec 16;60(6):1469–1480.
- Wintermark P, Mohammad K, Bonifacio SL. Proposing a care practice bundle for neonatal encephalopathy during therapeutic hypothermia. Semin Fetal Neonatal Med. 2021 Oct;26(5):101303. doi: 10.1016/j.siny.2021.101303
- Wojtyniak JG, Selzer D, Schwab M, et al. Physiologically based precision dosing approach for drug-drug-gene interactions: A simvastatin network analysis. Clin Pharmacol Ther. 2021 Jan;109(1):201–211.
- Min JS, Bae SK. Prediction of drug-drug interaction potential using physiologically based pharmacokinetic modeling. Arch Pharm Res. 2017 Dec;40(12):1356–1379. doi: 10.1007/s12272-017-0976-0
- Croft NM, de Ridder L, Griffiths AM, et al. Paediatric inflammatory bowel disease: a multi-stakeholder perspective to improve development of drugs for children and adolescents. Journal Of Crohn’s And Colitis. 2022 Sep 21;17(2):249–258. doi: 10.1093/ecco-jcc/jjac135
- Pearson ADJ, Weiner SL, Adamson PC, et al. ACCELERATE - Five years accelerating cancer drug development for children and adolescents. Eur J Cancer. 2022 May;166:145–164.