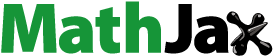
Abstract
Graphene is a novel material which has recently been gaining great interest in the biomedical fields. Our previous study observed that graphene-derived particles help induce bone formation in a murine calvarial model. Here, we further developed a blended graphene-contained polycaprolactone (PCL/G) filament for application in a 3D-printed bone scaffold. Since implants are expected to be for long-term usage, in vitro cell culture and in vivo scaffold implants were evaluated in a critical-size bone defect calvarial model for over 60 weeks. Graphene greatly improved the mechanical strength by 30.2% compared to pure PCL. The fabricated PCL/G scaffolds also showed fine cell viability. In animal model, an abnormal electroencephalogram power spectrum and early signs of aging, such as hair graying and hair loss, were found in the group with a PCL/G scaffold compared to pure PCL scaffold. Neither of the abnormal symptoms caused death of all animals in both groups. The long-term use of graphene-derived biomaterials for in-vivo implants seems to be safe. But the comprehensive biosafety still needs further evaluation.
Background
Graphene is a novel material which has recently been gaining great interest in the biomedical field (Alagarsamy et al. Citation2021; Zhao et al. Citation2021). Graphene is a two-dimensional (2D) single-atom-thick sheet of sp2-hybridized hexagonally arranged carbon atoms within a carbon material structure (Mittal et al. Citation2020). Graphene shows promising characteristics, including excellent mechanical properties, atomic structure stability, and electrical conductivity (Qu et al. Citation2018; Du et al. Citation2020). Graphene-based nanomaterials have also been used in dermatology research, due to their excellent biological properties such as cell proliferation stimulation, antibacterial properties, and biocompatibility (Zhao et al. Citation2021). In cardiovascular diseases, carbon nanomaterials like graphene showed a promising potential in the clinical translation of biomaterials-based therapies (Alagarsamy et al. Citation2021). 3D graphene foams were developed and showed the ability to maintain human mesenchymal stem cell (hMSC) viability and induce spontaneous osteogenic differentiation (Crowder et al. Citation2013). Incorporated graphene derivate into Zn or positively charged Fe3O4 (pFe3O4) scaffold not only increased its mechanical properties but also improved cell biocompatibility and alkaline phosphatase activity (Yang et al. Citation2020, Citation2021).
In our previous study (Chang et al. Citation2020), we tested the in vitro and in vivo biological response of graphene particles using a murine calvarial model and observed bone formation around the calvarial area. Other researchers have also studied the osteogenic potential of graphene (Eivazzadeh-Keihan et al. Citation2019; Du et al. Citation2020; Peng et al. Citation2020). Graphene has been introduced into different kinds of substrates. As showed in Shahin et al. (Citation2020) graphene nanoplatelets had the potential to improve the mechanical properties of magnesium-based metal matrix nanocomposites. A fabricated scaffold with graphene mixed with the synthetic polymer PCL showed a stimulated cell proliferation and lower immune response in vitro. This scaffold induced new tissue formation and osteogenic marker expression in vivo (Wang et al. Citation2019). Based on the literatures, a fabricated scaffold with graphene-derived polymer could provide a promising alternative strategy in bone regeneration applications.
Bone defects caused by trauma, resection of bone tumor, osteoporosis, or other pathological problems, are a substantial challenge in orthopedic surgery (Campana et al. Citation2014; Yan et al. Citation2019). Autogenous bone graft is still considered as the gold standard for bone substitution. However, the drawbacks of autografts are poor availability, donor-site morbidity, and its resulting complications (Zhang et al. Citation2019; Shang et al. Citation2021). Artificial bone substitution developed by tissue engineering is an alternative for bone grafting. The application of biocompatible cell scaffolds has been evaluated for bone tissue engineering in recent years (Shahin-Shamsabadi et al. Citation2018; Siddiqui et al. Citation2018). Additive manufacturing or three-dimensional (3D) printing is a cutting-edge technology in BTE. Especially in orthopedic implants, cell scaffolds fabricated via additive manufactured technology (3D bio-printing) have been widely studied (Bandyopadhyay et al. Citation2020; Chen et al. Citation2020). With advanced computer-aided design software and different newly developed materials, a 3D bio-printing technique allows researchers to make customized structures with different geometries, sizes, and porosities to obtain more osteo-inductive cell scaffolds (Jariwala et al. Citation2015). Meanwhile, 3D bio-printing processes are able to control the micro-architecture and composition at the micron-scale of the scaffolds (Zhang et al. Citation2019). Three dimensional printed Zn-doped mesoporous silica-incorporated poly-L-lactic acid and calcium phosphate cement by incorporating 3D plotted poly(lactic-co-glycolic acid) network have also used to modify osteogenic activity of polymer scaffolds (Qian et al. Citation2019, Citation2021).
Polycaprolactone (PCL), a synthetic biodegradable aliphatic polyester, has been widely investigated in cell scaffold applications (Siddiqui et al. Citation2018). PCL is a semi-crystalline thermoplastic with a low melting point of around 60 °C that provides easy formability (Shang et al. Citation2021). PCL has several advantages in tissue engineering, including a good biocompatibility, little immunological and inflammatory stimulation, and support of cell regulation (Shahin-Shamsabadi et al. Citation2018). PCL has been used as an implant material for FDA-approved devices (Kuchler-Bopp et al. Citation2017). However, insufficient mechanical strength remains a concern (Shahin-Shamsabadi et al. Citation2018). There is still room to improve bone tissue scaffolds.
Results from above mentioned studies have shown that graphene offers the potential to induce bone formation with other materials. Once combined with PCL, graphene could compensate the shortages of PCL by improving both its mechanical property and cellular affinity due to hydrophobicity. However, the effectiveness of combining the graphene-derived nanomaterial with PCL remains unclear and needs to be comprehensively evaluated. Also, the cytotoxicity of graphene related materials has been evaluated in eyes and reproductive system (Liang et al. Citation2015; Wu et al. Citation2016), but not in the brains. This study aimed to develop an evenly blended PCL/graphene (PCL/G) 3D printing filament. The physical characteristics of PCL and PCL/G filaments were evaluated. The biological responses of the cell scaffold fabricated by 3D printing were determined via an in-vitro cell culture model and in-vivo animal model. Three targets were performed in the current study. First, the physical characteristics of graphene-derived materials were tested and compared to that of the pure PCL one. Second, in-vitro and in-vivo biological responses of the graphene-derived materials were analyzed using well established cell culture and animal models. Third, a further electroencephalogram (EEG) evaluation was performed to determine whether the electrical conductivity of graphene-obtained material affects brain functions.
Materials and methods
Filament fabrication
Single layer graphene (Sigma–Aldrich, St. Louis, MO, USA) was used in our previous study (Chang et al. Citation2020). Graphene nanosheets were compounded with polycaprolactone (PCL) polymer (MeDFila®, Advanced biomedical technology, Taiwan) via gentle agitation in 2wt% as stock resin under 85 °C in a dry heat tank. The concentration of graphene was adjusted to 0.5wt% and extruded into a 1.75 mm filament with an automatic micro extrusion system in 95 °C. The concentration chosen to be 0.5wt% was obtained from Qi et al. (Citation2018) and Mahdavi et al. (Citation2020). Scaffolds containing around 0.5% graphene derivatives showed the best cell viability as compared to other percentages.
Thermogravimetric analysis (TGA)
A TGA analysis was conducted for filaments in vitro. The onset of thermal degradation and contents of graphene in these filaments were assessed using a thermogravimetric analyzer (Perkin Elmer, MA, USA) equipped with an evolved nitrogen gas analysis furnace. The TGA was performed on PCL and PCL/G filaments. Scans were performed in an air atmosphere with a flow rate 60 mL/min and a temperature ranging from room temperature to 800 °C at a rate of change of 10 °C/min. Measurements were obtained using a sample mass ranging from 11 to 17 mg in platinum pans (TA Instrument, New Castle, DE, USA), and performed in triplicate. The weight loss of the PCL-based graphene composite was monitored and used to calculate the final residual graphene contents.
Morphology observation
The morphology of graphene nanosheets was observed by transmission electron microscopy (TEM) (JEM-1400 Flash; JEOL Co., Tokyo, Japan) analyzed at 80 kV. The nanosheets were dispersed in ddH2O by ultrasonication for 10 min. The suspensions were lifted on a carbon-coated copper grid (# 200 mesh, CF200-Cu), and dried in air, followed by TEM inspection.
The cross-sections of PCL and PCL/G filaments were prepared by paraffin embedding, and sliced by microtome with a thickness of 60 μm. The samples were coated with platinum using a vacuum sputter coater (E-1030 ION SPUTTER; Hitachi, Tokyo, Japan) and analyzed at 15 kV by a scanning electron microscopy (SEM) (S-3500N; Hitachi, Tokyo, Japan).
Fourier-Transform infrared spectroscopy (FTIR) spectrum analysis
A Fourier-transform infrared spectroscopy (FTIR) spectrum was obtained using a Spotlight 200i Sp2 with an AutoATR System (Perkin Elmer, Waltham, MA, USA). The scan range was 500–4000 cm−1 at a resolution of 0.5 cm−1 (Ţucureanu et al. Citation2016).
Micro-Raman spectrum analysis
Micro-Raman Spectrum (ACRON; UniNanoTech Co., Yongin-si, Korea) was used to examine the composition of the graphene nanosheets and PCL/G composites. The excitation source was a 532 nm laser (Wu et al. Citation2018).
Apparent water-in-air contact angle
The hydrophilicity was determined by the water contact angle test using the Model 100SB (Sindatek, New Taipei, Taiwan). Contact angle of a water drop (Droplet volume: 0.2 μL) with a solid surface was measured. The tests were repeated for three times in each group.
Mechanical behaviors
Experimental study of tensile strength
Test specimens for mechanical behaviors were prepared following ASTM C1557-20 (International Citation2020). Testing fibers were printed with an Infinity X1 printer (INFINITY3DP, Taiwan) with an extruder temperature of 100–110 °C and bundled and glued onto card tabs with a central window cutout equal to the intended gage length of 10 mm for the test (Supplementary Figure 1(A)). An optical microscope (Leica MZ6, Wetzlar, Germany) was used to measure the fiber diameter. Five apparent diameter measurements were made for each fiber at random positions (Supplementary Figure 1(B)). Tensile properties of each sample were measured with a Material Testing System machine (Acumen, MTS Corporation, Minneapolis, MN, USA) according to ASTM C1557-20 (International Citation2020). This machine was equipped with a 100 N load cell. Tests were performed in displacement control at a cross-head speed of 5 mm/min (Kim et al. Citation2019). At least eight samples for each group were performed to obtain statistically reliable results (PCL, n = 14; PCL/G, n = 8).
Data collection, processing, and analysis
Raw data of force/displacement was collected using the software Multipurpose Elite Software (MTS Corporation, Minneapolis, MN, USA). All data points were plotted for each material on a diagram as a normative band including a linear elastic portion and nonlinear plastic portion. Elastic modulus, maximum strength, and yielding strain of the two groups were recorded for their comparison. The stress-strain curve was plotted and the values of stress and strain were then calculated using following equations:
where F indicates the normal force (N), Ao is the cross-sectional area (mm2), ΔL is the deformation, and L is the original length.
Scaffold fabrication
Scaffolds for the in vitro and in vivo models were designed with DesignSpark Mechanical (Ansys, Inc., Canonsburg, PA, U.S. & RS Components, Corby, UK) a free 3D CAD (computer-aided design) solid modeling software. Printing parameters were set via a slicing software KISSlicer (Orlando, FL, USA). The printing process used an Infinity X1 printer (INFINITY3DP, Taiwan) with a 0.2 mm diameter extruder. Scaffolds for in vitro tests were designed as a cylinder with a diameter of 11 mm and height of 0.4 mm (Wu et al. Citation2015). Printing parameters for animal model scaffolds had two layers with 0.2 mm of total layer thickness (Supplementary Figure 2(A)). Cylinders with 3 and 4 mm diameters were cut with dental trephine, and then combined with a 7–0 non-absorbable suture (UNIK surgical sutures Mfg. Co. Ltd., New Taipei city, Taiwan) to form the animal scaffolds (Supplementary Figure 2(B)).
Cell culture
The MC3T3-E1 murine preosteoblast cell line (ATCC CRL-2594, Manassas, VA, USA) was used. Cells were cultured in Minimum Essential Medium α (MEM α) (Gibco, Thermo Fisher Scientific, Waltham, MA, USA) supplemented with 1% penicillin/streptomycin (Gibco) and 10% fetal bovine serum (Gibco) at 37 °C in a humidified incubator with 5% CO2. Cells were then harvested using a 0.05% trypsin ethylenediaminetetraacetic acid (EDTA) solution (Gibco) for 10 min and washed with Dulbecco’s phosphate-buffered saline (Gibco). Viable cells were counted using trypan blue dye exclusion before seeding.
WST-1 assay
The amount of cell proliferation was assessed using the WST-1 Cell Proliferation Assay Kit (Cayman Chemical Company, Ann Arbor, MI, USA). A total of 1.5 × 104 MC3T3 cells were seeded on each scaffold in 400 μL of cell growth medium in a 48-well plate. After incubation for 3 and 7 days, 40 μL of WST-1 were added into each well and incubated for 2 h in a 37 °C CO2 incubator. The absorbance of each sample was measured by a microplate reader (SpectraMax 190, Molecular Devices, San Jose, CA, USA) at a wavelength of 450 nm.
Live/dead staining
Cell viability and adhesion were assessed using the Live/Dead Viability/Cytotoxicity Kit (Invitrogen, Waltham, MA, USA). A total of 1.5 × 104 MC3T3 cells were seeded on each scaffold in 400 μL cell growth medium in a 48-well plate. After incubation for 3 and 7 days, 150 μL of the combined Live/Dead assay reagents were added into each sample. The cells were incubated for 45 min at room temperature. The labeled cells were observed under the fluorescence microscope.
In vivo animal model
The protocol for this experiment was approved by the Institutional Animal Care and Use Committee of MacKay Memorial Hospital (MMH-A-S-107-53), where the study was performed. All animal procedures were performed according to the Guideline for the care and use of laboratory animals by Council of Agriculture, Executive Yuan, Taipei, Taiwan. A total of 20 fourteen-week-old C57BL/6J female mice were purchased from the National Laboratory Animal Center, Taipei, Taiwan, an AAALAC International Certified Biotechnology Company. Animals were kept in a room at 24 °C and 50% humidity, with a 12 h light/dark cycle (light from 7:00 a.m. to 7:00 p.m.).
Surgical procedure
The surgical techniques of critical-sized calvarial bone defect model were established and referenced from Samsonraj et al. (Citation2017) Animals were randomly separated into two groups, PCL (n = 10) and PCL/G (n = 10). Twenty-four hours before surgery, Carporfen (Rimadyl®) was added into the drinking water at a final concentration of 0.025 mg/mL. The mice were anesthetized with 2%–4% isoflurane via inhalation. An electric shaver was used to remove the hair between the eyes to the posterior end of the skull. A longitudinal skin incision of about 1.5 cm on the scalp of the mice was performed to expose the calvarium. The intact periosteum was gently removed, and two critical bone defects were created on both sides of the parietal bone with a 3 mm dental trephine (Tissue punches; Hager & Meisinger GmbH, Dusseldorf, Germany). After creating the defects, the surgical sites were washed with sterile saline. All the left defects were served as an empty control, and the scaffolds were placed into the right defects without applying pressure on the underlying brain. The incision was closed by a 5–0 Dafilon® non-absorbable suture (B. Braun, Hessen, Germany) with a simple interrupted suture pattern. Mice were provided Carporfen (Rimadyl®, Zoetis, NJ, USA) in drinking water with a final concentration of 0.025 mg/mL for 5 days post-operation.
Micro-CT imaging analysis
All live animals were anesthetized by isoflurane and analyzed at 1, 6, 12, 18, and 60 weeks post-surgery by Micro-CT (Skyscan 1176 Bruker Micro-CT, Kontich, Belgium) with the following parameters: voltage of 70 kV and electrical current of 353 μA. The Micro-CT was set in a 2048 × 2048 pixel matrix. The 3D images of calvarial defects were reconstructed with a voxel size of 18 μm. For quantitative analysis of bone formation, a spherical volume of interest was defined which consisted of a 3 mm diameter circle drawn around the edge of the defect to measure the differences in the bone mineral density (BMD, g/cm3), ratio of bone volume to tissue volume (BV/TV, %), and trabecular thickness (Tb.Th, mm) between each group.
Electroencephalography (EEG) analysis
After 50 weeks post-surgery, animals were studied with EEG (Hung-Shih Lin Citation2021). The non-treatment control group was included (n = 6) with PCL (n = 6) and PCL/G (n = 6). First, mice were anesthetized with 2% isoflurane for 3 min. We recorded resting-state EEG by applying electrodes to the mouse scalp for at least 6 min. Two electrodes were placed on frontal (Fz) and central (Cz) sides on the midline sagittal plane of the skull, and then one electrode was placed on the back skin as a background activity.
Data was recorded with Cadwell Easy III (Cadwell, Kennweick, WA). Persyst v 14 (Persyst Development Co., Prescott, AZ) was used to generate qEEG data, including the spectral power of the delta δ (1–4 Hz), theta θ (4–8 Hz), alpha α (8–13 Hz), and beta β (13–30 Hz) frequency bands. The relative delta power was calculated by dividing the spectrum power of the delta-band (1–4 Hz) by the summation power of all four frequency bands (delta, theta, alpha, and beta bands).
Statistical analysis
The data were first analyzed with a normality test and showed a normal distribution. Subsequently, data were analyzed by the one-way analysis of variance to determine the differences between groups. Multiple comparisons were adjusted with a Dunnett’s t3 post hoc test. The results were reported as mean ± standard deviation. A p-value lower than 0.05 was considered statistically significant.
Results
Morphology of graphene-derived nanomaterials
The morphology of graphene nanomaterials (sheet-liked) observed by TEM was shown in . The morphology of pristine graphene resembled two-dimensional irregularly shaped sheets. Particles were observed on the cross-section surface of 3D printing filament of PCL/G (), but no graphene particles or foreign matters were seen on the cross-section surface of the pure PCL filament.
Thermogravimetric analysis (TGA)
TGA was used to understand the composition of PCL-based filaments by characterizing the thermal properties of these filaments. The addition of graphene had a great effect on the decomposition temperature of PCL. The decomposition temperatures of PCL/G decreased from 397 °C to 332 °C. In other words, the decomposition of the polymer (PCL) chain started from 332 °C when graphene was added to the polymer. The reason can be attributed to endothermal properties of graphene resulting in a decrease in decomposition temperature.
Fourier-Transform infrared spectroscopy (FTIR) spectrum analysis
The FTIR spectrum of PCL and PCL/G showed the three prominent characteristic peaks at 1176, 1242, and 1722 cm−1 attributed to C–O stretching and C = O stretching in the ester group (). The bonds at 2872 and 2950 were attributed to H–C–H asymmetric and symmetric stretches (Mohammadi et al. Citation2017). Compared to pure PCL, the FTIR spectrum of PCL/G was observed with a similar location of the three peaks but with different intensity. The intensity of the peaks related to ester bonds (1176, 1242, and 1722 cm−1) increased, indicating that ester groups (COOR) formed in the PCL/G blend (Mohammadi et al. Citation2017).
Micro-Raman spectrum analysis
Raman spectroscopy is a widely used nondestructive tool for the characterization of carbon products (Kudin et al. Citation2008). Raman spectroscopy was used to determine the number of layers in graphene nanosheets and analyze their structural defects. All carbon-based materials tend to have a standard D band at the position of around 1350 cm−1 and G band around 1580 cm−1 () (Ferrari and Robertson Citation2000). The results showed that the graphene nanomaterials had the typical D and G bands. Compared with pure PCL, these bands confirmed the existence of graphene in the PCL/G.
Apparent water-in-air contact angle
The water contact angle of PCL was compared with that of PCL/G. A spherical water droplet with a contact angle of 88.51° (±1.83) was observed on the PCL surface. The water contact angle was 87.47° (±1.26) in the PCL/G group. There was no significant difference in water contact angle between PCL and PCL/G.
Mechanical properties
Mechanical strengths were significantly increased in the PCL/G group compared to PCL group (). The results of stress-strain curves of each group showed a good reproducibility. They significantly increased by 30.2% in elastic modulus and 19.4% in ultimate strength when compared with those in the PCL group. The yielding strain significantly increased by 20% in the PCL/G group compared to that in the PCL group, indicating that adding graphene into PCL improves ductility. It may also help to prevent sudden breakage when used in an orthopedic device.
Cell viability
The behaviors of the cells on different scaffolds were evaluated. Cell adhesion, proliferation, and morphology can be influenced by different biomaterials (Aidun et al. Citation2019). The Live/dead assay was used to observe the morphology of the cells and determine cell adhesion and viability. In the Live/Dead staining, cells in both PCL and PCL/G groups showed good adhesion following 3 days and the living cell intensity increased after 7 days (). However, PCL/G showed slightly more dead cells (red stained cell) compared to the PCL group at 3 days. The WST-1 assay confirmed the observed cell adhesion results (). After 3 days of incubation, PCL/G showed a lower viability compared to the PCL group (0.335 of PCL/G compared to 0.438 of PCL). However, PCL and PCL/G showed no significantly difference of cell viability at 7 days (0.580 of PCL/G compared to 0.631 of PCL).
In vivo animal model
No obvious changes in animal behaviors and body weight were observed for the two groups after surgery (Supplementary Figure 3). More importantly, after 6 weeks of operation, all mice in the PCL/G group showed hair loss at the posterior neck and back (). Fifty-six weeks after surgery, PCL and PCL/G groups both showed aging symptoms such as gray hair. But the hair loss condition was sustained in PCL/G group and the early onset of aging symptoms was more sever compared to that of the PCL only group (). Micro-CT images showed that the PCL/G group had a small amount of bone-like tissue in the right side of the defect, but there were no significant differences in the quantification of bone formation (Supplementary Figure 4).
Figure 6. (A) Hair condition of the PCL and PCL/G groups on week 12. (B) Hair condition of PCL and PCL/G groups on week 18 to 60.
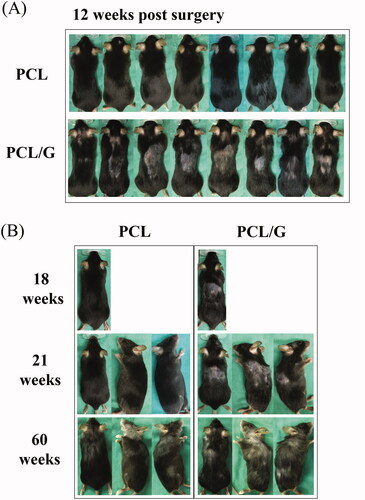
Because of the excellent electrical conductivity of graphene materials (Du et al. Citation2020), it was suspected that the early onset of aging symptoms might be due to the effects of graphene on the murine EEG. The results showed significant decreases in the relative delta spectra band (49.67%±10.67%) in the PCL/G group compared to that of the PCL and control groups (65.83%±9.03% and 67.42%±8.02%, respectively). Moreover, the relative theta power of the PCL/G group showed a significant increase compared to that of other groups (38.85%±10.36% in the PCL/G group versus 25.73%±7.35% in the PCL group, and 24.52 ± 8.23% in the control group) (). The relative beta power of PCL/G also was significantly higher than that of the other two groups (3.27%±0.78% in the PCL/G group versus 2.01%±0.87% in the PCL group and 1.73%±0.69% in the control group). There was no difference in the relative alpha power across all groups.
Discussion
Graphene-derived nanomaterials have been studied in the bone repair field for their promising potential in bone formation (Shin et al. Citation2018; Han et al. Citation2019). PCL and graphene composites have been shown to induce cell growth and tissue formation both in vitro and in vivo (Wang et al. Citation2016, Citation2019). This study attempted to develop a graphene-containing 3D printing PCL filament for application in an additive manufactured bone scaffold. Compared to other additive manufacturing methods, PCL has also been doped with other materials through electrospinning technology, the ingredients as silicate- and strontium-containing hydroxyapatite which can be added in the scaffold and has been demonstrated with satisfied antibacterial activity against gram-positive bacterium (Surmenev et al. Citation2019) with a controllable degradation rate (Melnik et al. Citation2019). Therefore electrospinning can be one of the beneficial fabrications for bone tissue regeneration (Melnik et al. Citation2019; Surmenev et al. Citation2019). On the other hand, the PCL-contained graphene filament developed in current study facilitates to adapt the porosity and the microstructures of scaffolds through additive manufacturing technology (3D printing), thus adjustable mechanical strength would be considered the clinical intended-use advantage to fit the defects (Nowicki et al. Citation2016). This technique can be more convenient for customized applications in bone defects with random or irregular shapes.
Although PCL composite materials have been widely studied (Bharadwaz and Jayasuriya Citation2020), its low mechanical strength weakness characteristics in mechanical strength restricts its application in the orthopedic field. Different contents of graphene derivatives (GD) have been used to reinforce chitosan/β-glycerophosphate hydrogel. 0.5% of GD addition showed the highest cellular viability compared to other contents (1% and 2%) (Qin et al. Citation2018). Mahdavi et al. mixed GD with gelatin–hydroxyapatite scaffold. 0.5% of GD showed the highest Young’s modulus, and the mechanical properties decreased with higher GD contents (Mahdavi et al. Citation2020). The cell viability also showed higher value in 0.5% of GD compared to other contents (1% and 2%). GD were also mixed with ultrahigh molecular weight polyethylene (UHMWPE) from 0.1wt% to1.0wt% (Pang et al. Citation2015). 0.5wt% of GD showed the highest tensile properties compared to higher or lower concentrations. We refer to the above-mentioned researches and choose 0.5% of graphene content in the current study. Our mechanical property tests showed that PCL/G had a significantly higher elastic modulus (30.2%) and ultimate strength (19.4%) compared to those of the PCL group. Similar improvement in mechanical strength was reported by Wang et al. (Citation2018) who tested the elastic modulus of PCL and a GD containing PCL. The PCL filament used in our study showed a higher elastic modulus than the PCL used in theirs (284.7 MPa and 202 MPa, respectively). The possible difference between the two studies might be due to the fiber preparing process. The tested fibers used in their research were produced from direct melt extrusion, while the production of the tested fibers in this study used a commercialized 3D printing filament heated by the nozzle of the 3D printer and drawn into tested fibers. The test material was subjected to a reheating step, which might affect the test value. The material properties of PCL might differ based on manufacturing methods. However, our method help improve the mechanical strength through filament development. Moreover, the yielding strain significantly increased by 20% in the PCL/G group compared to that in the PCL group, indicating that adding graphene improves ductility. As we know, the advantage of additive manufacturing technology facilitates to build an arbitrary structure to fill the bone defect. And through the technique, it facilitates adjustments of printing different micro-arrangement and porosity to target the desired mechanical strength of 3D structure scaffold to fit bone defects. Current results found out the filament with graphene significantly improved its mechanical strength in terms of yielding stress and Young’s modulus, and also revealed better mechanical characteristics of ductility and malleability as compared to pure PCL filament. Although current study did not highlight the superiority of the improved mechanical behaviors in the graphene-contained scaffold, we believe this study creates a promising technology to develop the optimal scaffold to approach better bone defect recovery and thus can be expected better mechanobiology environment for bone regeneration.
Murine calvarial bone defect model in the current study has been widely used for evaluating osteogenesis potential. GD composite materials showed promising osteogenicity (Hermenean et al. Citation2017; Qi et al. Citation2020). In the animal study, Micro-CT (Micro computed tomography) analysis showed that the PCL/G group had a small amount of bone-like tissue in the defect sites, but there were no significant differences in the quantity of bone formation (BMD, Tb. Th, BV/TV) between PCL and PCL/G groups. The possible reason might be that graphene nanosheets blended into PCL filament reduced the contact ratio between the bone and the graphene nanosheets. The degradation profile of PCL is approximately 1 ∼ 2 years (52–104 weeks) (Bliley and Marra Citation2015; Manoukian et al. Citation2019). Research also indicated that PCL scaffold showed negligible mass loss after 2-years implantation in rabbit calvaria (Lam et al. Citation2009). The exposure rate of the nanosheets to bone cells is much lower than our previous study which providing 1 mg of abundant nanosheets. So the bone formation did not show promising results compared to our previous study which graphene particles directly contacted to calvarial bone (Chang et al. Citation2020). On the other hand, hair loss was observed in the PCL/G group after 6 weeks post-operation. The possible reason to these signs could be spontaneous overgrooming, or related strains in C57BL6/J (De Biase et al. Citation2019). We first provided an enriched environment, such as giving some wood toys for all groups to reduce adult alopecia in mice (Bechard et al. Citation2011). The hair growth in the PCL/G group then slightly improved, but it still showed more hair loss than the PCL alone group (). In our studies, only the PCL/G group showed a significant hair loss compared to the PCL group up to 60 weeks after implantation.
Blood samples from two mice sacrificed due to sever ulcerative dermatitis in the PCL/G group were collected for testing. The complete blood count results showed %NEUT (%neutrophils, 36.7 and 32.2) and %EOS (%eosinophils, 2.0 and 3.6) which were higher than normal (16.13%NEUT and 1.09%EOS). Increased neutrophil counts are associated with responses to stress or excitement (Schwab et al. Citation2005), bacterial infection and acute inflammation (Fernandez et al. Citation2006), and eosinophils are involved in parasitic and allergic reactions (Yamada et al. Citation2009; O'Connell et al. Citation2015). It was speculated that these alterations may result from graphene which is released from the PCL/G scaffold. To ensure graphene biosafety and elucidate whether it induced the behavior of plucking fur, further comprehensive study designs are necessary. This is the first study of its kind to observe the long-term in vivo biological responses. Although all animals were alive for over 60-weeks post-operation before being sacrificed, the group with the graphene-containing scaffolds showed distinguished signs of hair loss and gray hair color ().
Comparing the EEG power spectrum from wild-type mice on a C57BL/6J background in other studies (Born et al. Citation2017; Kent et al. Citation2018; Copping and Silverman Citation2021), the PCL and control groups showed a similar relative EEG power spectrum. The results of PCL/G showed that a lower delta and higher theta power could account for the cause of the behavior-associated hair loss. It has been reported that graphene derivatives have the potential to cross the blood-brain barrier and cause neurotoxicity (Ou et al. Citation2016). We hypothesized that the presence of graphene with electrical conductivity around the brain might influence electrophysiological phenotypes, caused more stress, and induced more severe barbering-induced alopecia. Barbering is a complex behavior, and further studies are necessary.
The safety concerns of graphene family have been discussed in the past few years (Tonelli et al. Citation2015; Ou et al. Citation2016). Few-layer graphene could induce cytoskeletal dysfunction, reduction in metabolic activity, and oxidative stress mediated genotoxicity in human primary endothelial cells (Sasidharan et al. Citation2016). Graphene oxide might influence the functions of different signal pathways including insulin/insulin-like growth factor, target of rapamycin and germline signaling pathways controlled by miRNAs and reduce the lifespan of animals (Wu et al. Citation2014). It has also been reported that through different exposure ways, graphene family could be distributed in different organs of laboratory animals (Kim et al. Citation2016; Ema et al. Citation2017). However, there is no study discussing the toxicity of graphene in animal skin or brain. The current study is the first to report the biosafety concern of graphene in long-term animal models.
There were limitations to this study. First, different contents of graphene derivative would affect its characteristics. Only 0.5% concentration was tested in the current study. In Qin et al. (Citation2018) and Mahdavi et al. (Citation2020) scaffolds containing 0.5% GD particles showed the best cell viability compared to those containing other percentages. Therefore, the current study only chose the concentration of 0.5% for analysis. Second, ossification observation has been extensively demonstrated in many in vitro and in vivo studies which determined the beneficial effect of adding graphene-derives nanomaterials (Wang et al. Citation2019; Ge et al. Citation2021). This current study further attempted to evaluate the long-term safety of these novel materials. Research on graphene inducing bone regeneration with an in vivo model has been usually maintained for 8–17 weeks after surgery (Lu et al. Citation2016; Wang et al. Citation2019; Ge et al. Citation2021). To the best of our knowledge, this is the first study analyzing the effects of graphene-containing materials with an in vivo model over 60 weeks of long-term follow-up. The current results found some specific hair signs which could be considered as possible adverse effects of the novel materials. Finally, in order to eliminate possible bias of hormones, only female was considered in current study (Lee et al. Citation2019; Lienemann et al. Citation2015). Besides the above-mentioned limitations, the authors believe that this study provides important information to understand in vivo biosafety of graphene-containing nanomaterials when used for biomedical implants or orthopedic bone regeneration.
Conclusions
The mechanical properties of PCL/G are significantly improved by the addition of graphene. The Young’s modulus of PCL/G increased by 30.2%, and showed a better ductility approximately 20.0% compared to that of pure PCL. Cell viability in the fabricated PCL/G cell scaffold showed no significant difference as compared to the controls. However, the present study found abnormal EEG signals and early signs of aging in the group with graphene-containing scaffold but not in the group with pure PCL scaffold. This is the first study detected brain functions when implanting graphene-derived bone scaffold with electrical conductivity. The biosafety of graphene-containing materials needs further comprehensive evaluation before clinical application particular if use around the brain system. The methodology established in the present study can be used to assess the biosafety of other novel graphene-derived nanoparticles such as graphene oxide and reduced graphene oxide.
Supplemental Material
Download MS Word (3.5 MB)Disclosure statement
The authors declare that there is no conflict of interests.
Additional information
Funding
References
- Aidun, A., A. Safaei Firoozabady, M. Moharrami, A. Ahmadi, N. Haghighipour, S. Bonakdar, and S. Faghihi. 2019. “Graphene Oxide Incorporated Polycaprolactone/Chitosan/Collagen Electrospun Scaffold: Enhanced Osteogenic Properties for Bone Tissue Engineering.” Artificial Organs 43 (10): E264–E281. doi:https://doi.org/10.1111/aor.13474.
- Alagarsamy, K. N., S. Mathan, W. Yan, A. Rafieerad, S. Sekaran, H. Manego, and S. Dhingra. 2021. “Carbon Nanomaterials for Cardiovascular Theranostics: Promises and Challenges.” Bioactive Materials 6 (8): 2261–2280.
- Bandyopadhyay, A., I. Mitra, and S. Bose. 2020. “3D Printing for Bone Regeneration.” Current Osteoporosis Reports 18 (5): 505–514.
- Bechard, A., R. Meagher, and G. Mason. 2011. “Environmental Enrichment Reduces the Likelihood of Alopecia in Adult C57BL/6J Mice.” Journal of the American Association for Laboratory Animal Science 50 (2): 171–174.
- Bharadwaz, A., and A. C. Jayasuriya. 2020. “Recent Trends in the Application of Widely Used Natural and Synthetic Polymer Nanocomposites in Bone Tissue Regeneration.” Materials Science & Engineering. C, Materials for Biological Applications 110: 110698.
- Bliley, J. M., and K. G. Marra. 2015. “Chapter 11 - Polymeric Biomaterials as Tissue Scaffolds.” In: Vishwakarma, A., P. Sharpe, S. Shi, & M. Ramalingam (eds.) Stem Cell Biology and Tissue Engineering in Dental Sciences. Boston: Academic Press.
- Born, H. A., A. T. Dao, A. T. Levine, W. L. Lee, N. M. Mehta, S. Mehra, E. J. Weeber, and A. E. Anderson. 2017. “Strain-Dependence of the Angelman Syndrome Phenotypes in Ube3a Maternal Deficiency Mice.” Scientific Reports 7 (1): 8451.
- Campana, V., G. Milano, E. Pagano, M. Barba, C. Cicione, G. Salonna, W. Lattanzi, and G. Logroscino. 2014. “Bone Substitutes in Orthopaedic Surgery: From Basic Science to Clinical Practice.” Journal of Materials Science. Materials in Medicine 25 (10): 2445–2461.
- Chang, T. K., Y. C. Lu, S. T. Yeh, T. C. Lin, C. H. Huang, and C. H. Huang. 2020. “In Vitro and in Vivo Biological Responses to Graphene and Graphene Oxide: A Murine Calvarial Animal Study.” International Journal of Nanomedicine 15: 647–659.
- Chen, Y., W. Li, C. Zhang, Z. Wu, and J. Liu. 2020. “Recent Developments of Biomaterials for Additive Manufacturing of Bone Scaffolds.” Advanced Healthcare Materials 9 (23): e2000724. doi:https://doi.org/10.1002/adhm.202000724.
- Copping, N. A., and J. L. Silverman. 2021. “Abnormal Electrophysiological Phenotypes and Sleep Deficits in a Mouse Model of Angelman Syndrome.” Molecular Autism 12: 9.
- Crowder, S. W., D. Prasai, R. Rath, D. A. Balikov, H. Bae, K. I. Bolotin, and H. J. Sung. 2013. “Three-Dimensional Graphene Foams Promote Osteogenic Differentiation of Human Mesenchymal Stem Cells.” Nanoscale 5 (10): 4171–4176.
- DE Biase, D., F. Esposito, M. DE Martino, C. Pirozzi, A. Luciano, G. Palma, G. M. Raso, et al. 2019. “Characterization of Inflammatory Infiltrate of Ulcerative Dermatitis in C57BL/6NCrl-Tg(HMGA1P6)1Pg Mice.” Laboratory Animals 53 (5): 447–458.
- Du, Z., C. Wang, R. Zhang, X. Wang, and X. Li. 2020. “Applications of Graphene and Its Derivatives in Bone Repair: Advantages for Promoting Bone Formation and Providing Real-Time Detection, Challenges and Future Prospects.” International Journal of Nanomedicine 15: 7523–7551. doi:https://doi.org/10.2147/IJN.S271917.
- Eivazzadeh-Keihan, R., A. Maleki, M. DE LA Guardia, M. S. Bani, K. K. Chenab, P. Pashazadeh-Panahi, B. Baradaran, A. Mokhtarzadeh, and M. R. Hamblin. 2019. “Carbon Based Nanomaterials for Tissue Engineering of Bone: Building New Bone on Small Black Scaffolds: A Review.” Journal of Advanced Research 18: 185–201. doi:https://doi.org/10.1016/j.jare.2019.03.011.
- Ema, M., M. Gamo, and K. Honda. 2017. “A Review of Toxicity Studies on Graphene-Based Nanomaterials in Laboratory Animals.” Regulatory Toxicology and Pharmacology: RTP 85: 7–24.
- Fernandez, G. C., M. F. Lopez, S. A. Gomez, M. V. Ramos, L. V. Bentancor, R. J. Fernandez-Brando, V. I. Landoni, et al. 2006. “Relevance of Neutrophils in the Murine Model of Haemolytic Uraemic Syndrome: mechanisms Involved in Shiga Toxin Type 2-Induced Neutrophilia.” Clinical and Experimental Immunology 146 (1): 76–84.
- Ferrari, A. C., and J. Robertson. 2000. “Interpretation of Raman Spectra of Disordered and Amorphous Carbon.” Physical Review B 61 (20): 14095–14107. doi:https://doi.org/10.1103/PhysRevB.61.14095.
- Ge, Y. W., X. L. Liu, D. G. Yu, Z. A. Zhu, Q. F. Ke, Y. Q. Mao, Y. P. Guo, and J. W. Zhang. 2021. “Graphene-Modified CePO4 Nanorods Effectively Treat Breast Cancer-Induced Bone Metastases and Regulate Macrophage Polarization to Improve Osteo-Inductive Ability.” Journal of Nanobiotechnology 19 (1): 11. doi:https://doi.org/10.1186/s12951-020-00753-9.
- Han, S., J. Sun, S. He, M. Tang, and R. Chai. 2019. “The Application of Graphene-Based Biomaterials in Biomedicine.” American Journal of Translational Research 11 (6): 3246–3260.
- Hermenean, A., A. Codreanu, H. Herman, C. Balta, M. Rosu, C. V. Mihali, A. Ivan, S. Dinescu, M. Ionita, and M. Costache. 2017. “Chitosan-Graphene Oxide 3D Scaffolds as Promising Tools for Bone Regeneration in Critical-Size Mouse Calvarial Defects.” Scientific Reports 7 (1): 16641.
- Hung-Shih Lin, H.-W F. 2021. “EEG-Based Repetitive Transcranial Magnetic Stimulation for Treatment of Autism Spectrum Disorder.” Biomedical Journal of Scientific & Technical Research 34: 27186–27189.
- International, A. 2020. ASTM C1557-20, Standard Test Method for Tensile Strength and Young's Modulus of Fibers. West Conshohocken, PA: ASTM International.
- Jariwala, S. H., G. S. Lewis, Z. J. Bushman, J. H. Adair, and H. J. Donahue. 2015. “3D Printing of Personalized Artificial Bone Scaffolds.” 3D Printing and Additive Manufacturing 2 (2): 56–64.
- Kent, B. A., S. M. Strittmatter, and H. B. Nygaard. 2018. “Sleep and EEG Power Spectral Analysis in Three Transgenic Mouse Models of Alzheimer's Disease: APP/PS1, 3xTgAD, and Tg2576.” Journal of Alzheimer's Disease 64 (4): 1325–1336.
- Kim, J. K., J. H. Shin, J. S. Lee, J. H. Hwang, J. H. Lee, J. E. Baek, T. G. Kim, et al. 2016. “28-Day Inhalation Toxicity of Graphene Nanoplatelets in Sprague-Dawley Rats.” Nanotoxicology 10 (7): 891–901.
- Kim, T., G. Han, and Y. Jung. 2019. “Facile Fabrication of Polyvinyl Alcohol/Edge-Selectively Oxidized Graphene Composite Fibers.” Materials (Basel, Switzerland) [Online] 12(21): 3525.
- Kuchler-Bopp, S., A. Larrea, L. Petry, Y. Idoux-Gillet, V. Sebastian, A. Ferrandon, P. Schwinte, M. Arruebo, and N. Benkirane-Jessel. 2017. “Promoting Bioengineered Tooth Innervation Using Nanostructured and Hybrid Scaffolds.” Acta Biomaterialia 50: 493–501.
- Kudin, K. N., B. Ozbas, H. C. Schniepp, R. K. Prud'Homme, I. A. Aksay, and R. Car. 2008. “Raman Spectra of Graphite Oxide and Functionalized Graphene Sheets.” Nano Letters 8 (1): 36–41.
- Lam, C. X. F., D. W. Hutmacher, J.-T. Schantz, M. A. Woodruff, and S. H. Teoh. 2009. “Evaluation of Polycaprolactone Scaffold Degradation for 6 Months in Vitro and in Vivo.” Journal of Biomedical Materials Research 90(3):906–919.
- Lee, M. S., D. Lee, J. Jin, G. Tae, Y. M. Shin, and H. S. Yang. 2019. “Biofabrication and Application of Decellularized Bone Extracellular Matrix for Effective Bone Regeneration.” Journal of Industrial and Engineering Chemistry 83:323–332.
- Liang, S., S. Xu, D. Zhang, J. He, and M. Chu. 2015. “Reproductive Toxicity of Nanoscale Graphene Oxide in Male Mice.” Nanotoxicology 9 (1): 92–105.
- Lienemann, P. S., S. Metzger, A. Sofia-Kiveli, A Blanc, P. Papageorgiou, A. Astolfo, B. R. Pinzer, et al. 2015. “Longitudinal In Vivo Evaluation of Bone Regeneration by Combined Measurement of Multi-Pinhole SPECT and Micro-CT for Tissue Engineering.” Scientific Report 5:10238.
- Lu, J., C. Cheng, Y. S. He, C. Lyu, Y. Wang, J. Yu, L. Qiu, D. Zou, and D. Li. 2016. “Multilayered Graphene Hydrogel Membranes for Guided Bone Regeneration.” Advanced Materials (Deerfield Beach, Fla.) 28 (21): 4025–4031.
- Mahdavi, R., G. Belgheisi, M. Haghbin-Nazarpak, M. Omidi, A. Khojasteh, and M. Solati-Hashjin. 2020. “Bone Tissue Engineering Gelatin-Hydroxyapatite/Graphene Oxide Scaffolds with the Ability to Release Vitamin D: fabrication, Characterization, and in Vitro Study.” Journal of Materials Science. Materials in Medicine 31 (11): 97.
- Manoukian, O. S., N. Sardashti, T. Stedman, K. Gailiunas, A. Ojha, A. Penalosa, C. Mancuso, M. Hobert, and S. G. Kumbar. 2019. “Biomaterials for Tissue Engineering and Regenerative Medicine.” In: NARAYAN, R. (ed.) Encyclopedia of Biomedical Engineering. Oxford: Elsevier.
- Melnik, E.,. S. Shkarina, S. Ivlev, V. Weinhardt, T. Baumbach, M. Chaikina, M. Surmeneva, and R. Surmenev. 2019. “In Vitro Degradation Behaviour of Electrospun Hybrid Scaffolds of Polycaprolactone and Strontium-Containing Hydroxyapatite Microparticles.” Polymer Degradation and Stability 167:21–32.
- Mittal, S. K., D. Goyal, A. Chauhan, and R. K. Dang. 2020. “Graphene Nanoparticles: The Super Material of Future.” Materials Today: Proceedings 28: 1290–1294.
- Mohammadi, S., S. S. Shafiei, M. Asadi-Eydivand, M. Ardeshir, and M. Solati-Hashjin. 2017. “Graphene Oxide-Enriched Poly(ε-Caprolactone) Electrospun Nanocomposite Scaffold for Bone Tissue Engineering Applications.” Journal of Bioactive and Compatible Polymers 32 (3): 325–342. doi:https://doi.org/10.1177/0883911516668666.
- Nowicki, M. A., N. J. Castro, M. W. Plesniak, and L. G. Zhang. 2016. “3D Printing of Novel Osteochondral Scaffolds with Graded Microstructure.” Nanotechnology 27 (41): 414001.
- O'Connell, K. E., A. M. Mikkola, A. M. Stepanek, A. Vernet, C. D. Hall, C. C. Sun, E. Yildirim, J. F. Staropoli, J. T. Lee, and D. E. Brown. 2015. “Practical Murine Hematopathology: A Comparative Review and Implications for Research.” Comparative Medicine 65 (2): 96–113.
- Ou, L., B. Song, H. Liang, J. Liu, X. Feng, B. Deng, T. Sun, and L. Shao. 2016. “Toxicity of Graphene-Family Nanoparticles: A General Review of the Origins and Mechanisms.” Particle and Fibre Toxicology 13 (1): 57.
- Pang, Wenchao, Zifeng Ni, Guomei Chen, Guodong Huang, Huadong Huang, and Yongwu Zhao. 2015. “Mechanical and Thermal Properties of Graphene Oxide/Ultrahigh Molecular Weight Polyethylene Nanocomposites.” Royal Society of Chemistry Advances 5 (77): 63063–63072. doi:https://doi.org/10.1039/C5RA11826C.
- Peng, Z., T. Zhao, Y. Zhou, S. Li, J. Li, and R. M. Leblanc. 2020. “Bone Tissue Engineering via Carbon-Based Nanomaterials.” Advanced Healthcare Materials 9 (5): e1901495.
- Qi, C., Y. Deng, L. Xu, C. Yang, Y. Zhu, G. Wang, Z. Wang, and L. Wang. 2020. “A Sericin/Graphene Oxide Composite Scaffold as a Biomimetic Extracellular Matrix for Structural and Functional Repair of Calvarial Bone.” Theranostics 10 (2): 741–756.
- Qian, G., L. Zhang, G. Wang, Z. Zhao, S. Peng, and C. Shuai. 2021. “3D Printed Zn-Doped Mesoporous Silica-Incorporated Poly-L-Lactic Acid Scaffolds for Bone Repair.” International Journal of Bioprinting 7 (2): 346.
- Qian, Guowen, Peirong Fan, Fupo He, and Jiandong Ye. 2019. “Novel Strategy to Accelerate Bone Regeneration of Calcium Phosphate Cement by Incorporating 3D Plotted Poly(Lactic-co-Glycolic Acid) Network and Bioactive Wollastonite.” Advanced Healthcare Materials 8 (9): e1801325. doi:https://doi.org/10.1002/adhm.201801325.
- Qin, H., J. Wang, T. Wang, X. Gao, Q. Wan, and X. Pei. 2018. “Preparation and Characterization of Chitosan/beta-Glycerophosphate Thermal-Sensitive Hydrogel Reinforced by Graphene Oxide.” Frontiers in Chemistry 6: 565.
- Qu, Y., F. He, C. Yu, X. Liang, D. Liang, L. Ma, Q. Zhang, J. Lv, and J. Wu. 2018. “Advances on Graphene-Based Nanomaterials for Biomedical Applications.” Materials Science & Engineering. C, Materials for Biological Applications 90: 764–780.
- Samsonraj, R. M., A. Dudakovic, P. Zan, O. Pichurin, S. M. Cool, and A. J. VAN Wijnen. 2017. “A Versatile Protocol for Studying Calvarial Bone Defect Healing in a Mouse Model.” Tissue Engineering Part C: Methods 23 (11): 686–693. doi:https://doi.org/10.1089/ten.tec.2017.0205.
- Sasidharan, A., S. Swaroop, P. Chandran, S. Nair, and M. Koyakutty. 2016. “Cellular and Molecular Mechanistic Insight into the DNA-Damaging Potential of Few-Layer Graphene in Human Primary Endothelial Cells.” Nanomedicine: Nanotechnology, Biology and Medicine 12 (5): 1347–1355. doi:https://doi.org/10.1016/j.nano.2016.01.014.
- Schwab, C. L., R. Fan, Q. Zheng, L. P. Myers, P. Hebert, and S. B. Pruett. 2005. “Modeling and Predicting Stress-Induced Immunosuppression in Mice Using Blood Parameters.” Toxicological Sciences: An Official Journal of the Society of Toxicology 83 (1): 101–113.
- Shahin, M., K. Munir, C. Wen, and Y. Li. 2020. “Magnesium-Based Composites Reinforced with Graphene Nanoplatelets as Biodegradable Implant Materials.” Journal of Alloys and Compounds 828: 154461. doi:https://doi.org/10.1016/j.jallcom.2020.154461.
- Shahin-Shamsabadi, A., A. Hashemi, M. Tahriri, F. Bastami, M. Salehi, and F. Mashhadi Abbas. 2018. “Mechanical, Material, and Biological Study of a PCL/Bioactive Glass Bone Scaffold: Importance of Viscoelasticity.” Materials Science & Engineering. C, Materials for Biological Applications 90: 280–288.
- Shang, F., Y. Yu, S. Liu, L. Ming, Y. Zhang, Z. Zhou, J. Zhao, and Y. Jin. 2021. “Advancing Application of Mesenchymal Stem Cell-Based Bone Tissue Regeneration.” Bioactive Materials 6 (3): 666–683.
- Shin, Y. C., S.-J. Song, S. J. Jeong, B. Kim, I. K. Kwon, S. W. Hong, J.-W. Oh, and D.-W. Han. 2018. “Graphene-Based Nanocomposites as Promising Options for Hard Tissue Regeneration.” Advances in Experimental Medicine and Biology 1078: 103–117. doi:https://doi.org/10.1007/978-981-13-0950-2_6.
- Siddiqui, N., S. Asawa, B. Birru, R. Baadhe, and S. Rao. 2018. “PCL-Based Composite Scaffold Matrices for Tissue Engineering Applications.” Molecular Biotechnology 60 (7): 506–532.
- Surmenev, R. A., S. Shkarina, D. S. Syromotina, E. V. Melnik, R. Shkarin, I. I. Selezneva, A. M. Ermakov, et al. 2019. “Characterization of Biomimetic Silicate- and Strontium-Containing Hydroxyapatite Microparticles Embedded in Biodegradable Electrospun Polycaprolactone Scaffolds for Bone Regeneration.” European Polymer Journal 113: 67–77. doi:https://doi.org/10.1016/j.eurpolymj.2019.01.042.
- Tonelli, F. M., V. A. Goulart, K. N. Gomes, M. S. Ladeira, A. K. Santos, E. Lorencon, L. O. Ladeira, and R. R. Resende. 2015. “Graphene-Based Nanomaterials: biological and Medical Applications and Toxicity.” Nanomedicine (London, England) 10 (15): 2423–2450.
- Ţucureanu, V., A. Matei, and A. Avram. 2016. “FTIR Spectroscopy for Carbon Family Study.” Critical Reviews in Analytical Chemistry 46 (6): 502–520.
- Wang, H., M. Domingos, and F. Scenini. 2018. “Advanced Mechanical and Thermal Characterization of 3D Bioextruded Poly(e-Caprolactone)-Based Composites.” Rapid Prototyping Journal 24 (4): 731–738. doi:https://doi.org/10.1108/RPJ-10-2016-0165.
- Wang, W., G. Caetano, W.-H. Chiang, A. L. Sousa, J. Blaker, M. Frade, C. Frade, and P. BñRTOLO. 2016. “Morphological, Mechanical and Biological Assessment of PCL/Pristine Graphene Scaffolds for Bone Regeneration.” International Journal of Bioprinting 2 (2): 95–105. doi:https://doi.org/10.18063/IJB.2016.02.009.
- Wang, W., J. R. P. Junior, P. R. L. Nalesso, D. Musson, J. Cornish, F. Mendonca, G. F. Caetano, and P. Bartolo. 2019. “Engineered 3D Printed Poly(Varepsilon-Caprolactone)/Graphene Scaffolds for Bone Tissue Engineering.” Materials Science & Engineering. C, Materials for Biological Applications 100: 759–770.
- Wu, C., L. Xia, P. Han, M. Xu, B. Fang, J. Wang, J. Chang, and Y. Xiao. 2015. “Graphene-Oxide-Modified β-Tricalcium Phosphate Bioceramics Stimulate in Vitro and in Vivo Osteogenesis.” Carbon 93: 116–129. doi:https://doi.org/10.1016/j.carbon.2015.04.048.
- Wu, J. B., M. L. Lin, X. Cong, H. N. Liu, and P. H. Tan. 2018. “Raman Spectroscopy of Graphene-Based Materials and Its Applications in Related Devices.” Chemical Society Reviews 47 (5): 1822–1873.
- Wu, Q., Y. Zhao, G. Zhao, and D. Wang. 2014. “microRNAs Control of in Vivo Toxicity from Graphene Oxide in Caenorhabditis elegans.” Nanomedicine: Nanotechnology, Biology, and Medicine 10 (7): 1401–1410.
- Wu, W., L. Yan, Q. Wu, Y. Li, Q. Li, S. Chen, Y. Yang, Z. Gu, H. Xu, and Z. Q. Yin. 2016. “Evaluation of the Toxicity of Graphene Oxide Exposure to the Eye.” Nanotoxicology 10 (9): 1329–1340. doi:https://doi.org/10.1080/17435390.2016.1210692.
- Yamada, Y., J. A. Cancelas, and M. E. Rothenberg. 2009. “Murine Model of Hypereosinophilic Syndromes/Chronic Eosinophilic Leukemia.” International Archives of Allergy and Immunology 149 Suppl 1 (Suppl 1): 102–107.
- Yan, Y., H. Chen, H. Zhang, C. Guo, K. Yang, K. Chen, R. Cheng, et al. 2019. “Vascularized 3D Printed Scaffolds for Promoting Bone Regeneration.” Biomaterials 190-191: 97–110.
- Yang, W., Y. Zhong, C. He, S. Peng, Y. Yang, F. Qi, P. Feng, and C. Shuai. 2020. “Electrostatic Self-Assembly of pFe3O4 Nanoparticles on Graphene Oxide: A co-Dispersed Nanosystem Reinforces PLLA Scaffolds.” Journal of Advanced Research 24: 191–203. doi:https://doi.org/10.1016/j.jare.2020.04.009.
- Yang, Y., Y. Cheng, S. Peng, L. Xu, C. He, F. Qi, M. Zhao, and C. Shuai. 2021. “Microstructure Evolution and Texture Tailoring of Reduced Graphene Oxide Reinforced Zn Scaffold.” Bioactive Materials 6 (5): 1230–1241.
- Zhang, L., G. Yang, B. N. Johnson, and X. Jia. 2019. “Three-Dimensional (3D) Printed Scaffold and Material Selection for Bone Repair.” Acta Biomaterialia 84: 16–33.
- Zhao, M., J. Shi, W. Cai, K. Liu, K. Shen, Z. Li, Y. Wang, and D. Hu. 2021. “Advances on Graphene-Based Nanomaterials and Mesenchymal Stem Cell-Derived Exosomes Applied in Cutaneous Wound Healing.” International Journal of Nanomedicine 16: 2647–2665.