Abstract
Carbon black nanoparticles (CBNPs) have a large surface area/volume ratio and are known to generate oxidative stress and inflammation that may result in genotoxicity and cancer. Here, we evaluated the primary and inflammatory response-driven (i.e. secondary) genotoxicity of two CBNPs, Flammruss101 (FL101) and PrintexXE2B (XE2B) that differ in size and specific surface area (SSA), and cause different amounts of reactive oxygen species. Three doses (low, medium and high) of FL101 and XE2B were assessed in vitro in the lung epithelial (A549) and activated THP-1 (THP-1a) monocytic cells exposed in submerged conditions for 6 and 24 h, and in C57BL/6 mice at day 1, 28 and 90 following intratracheal instillation. In vitro, we assessed pro-inflammatory response as IL-8 and IL-1β gene expression, and in vivo, inflammation was determined as inflammatory cell infiltrates in bronchial lavage (BAL) fluid and as histological changes in lung tissue. DNA damage was quantified in vitro and in vivo as DNA strand breaks levels by the alkaline comet assay. Inflammatory responses in vitro and in vivo correlated with dosed CBNPs SSA. Both materials induced DNA damage in THP-1a (correlated with dosed mass), and only XE2B in A549 cells. Non-statistically significant increase in DNA damage in vivo was observed in BAL cells. In conclusion, this study shows dosed SSA predicted inflammation both in vivo and in vitro, whereas dosed mass predicted genotoxicity in vitro in THP-1a cells. The observed lack of correlation between CBNP surface area and genotoxicity provides little evidence of inflammation-driven genotoxicity in vivo and in vitro.
Introduction
Carbon black (CB) consists of elemental carbon in the form of particles that are produced industrially by highly controlled partial combustion, or thermal decomposition, of gaseous or liquid hydrocarbons. CB particles are poorly soluble, and the commercially available grades of CB differ in particle size, surface area, average aggregate mass, content of poly-aromatic hydrocarbons (PAH), morphology, and structure (IARC Citation2010). Potential health effects of CB nanoparticles (CBNPs) have been investigated extensively in cell and laboratory animal experiments, as well as in epidemiological studies of CB production workers (Sorahan and Harrington Citation2007; Morfeld et al. Citation2016; Dell et al. Citation2015; Morfeld and McCunney Citation2007; Ramanakumar et al. Citation2008; Greene et al. Citation1979; Straif et al. Citation2000). CBNPs have been used as a benchmark material for in vivo toxicological evaluation of diesel exhaust particles and urban air particulate matter, and included as reference material (i.e. Printex 90) in studies of different nanomaterials (Saber, Jacobsen, et al. Citation2012; Wallin et al. Citation2017; Poulsen et al. Citation2017; Knudsen et al. Citation2019; Danielsen, Bendtsen et al. Citation2020; Barfod et al. Citation2020; Bengtson et al. Citation2017, Bendtsen et al. Citation2019, Bendtsen et al. Citation2020).
Following pulmonary exposure, CBNPs cause oxidative stress, inflammation, and genotoxicity in terms of DNA damage (Bourdon, Halappanavar, et al. Citation2012; Bourdon, Saber, et al. Citation2012; Husain et al. Citation2015; Nymark et al. Citation2021; Jacobsen et al. Citation2011; Modrzynska et al. Citation2018; Bourdon et al. Citation2013). In a murine lung epithelial cell line (MML cells), CB induced mutations, and the mutation spectrum was consistent with being caused by oxidative damage to DNA and by reactive oxygen species (ROS) production (Jacobsen et al. Citation2007; Jacobsen et al. Citation2011). Further, CB induced clastogenic effects in some in vitro and in vivo studies, and the results have been put into perspectives by Chaudhuri et al. Citation2018 in a review on CB genotoxicity. The International Agency for Research on Cancer (IARC Citation2010) classified CB as possibly carcinogenic to humans (Group 2B) based on evidence of cancer development following inhalation in rats (IARC Citation2010).
Insoluble particles including carbon-based particles have been suggested to cause cancer by primary and secondary genotoxicity (Donaldson, Poland, and Schins Citation2010; Nymark et al. Citation2021). Primary genotoxicity is caused by direct interaction between particles and DNA, or by indirect interactions, i.e. via particle-induced generation of ROS (Evans et al. Citation2017, Citation2019; Donaldson, Poland, and Schins Citation2010). Secondary genotoxicity is genotoxicity caused by chronic inflammation (Bartek, Mistrik, and Bartkova et al. Citation2010; Evans et al. Citation2017). Carbon-based particles generate high amounts of ROS in cellular and acellular systems (Jacobsen et al. Citation2008), which may cause primary (direct) genotoxicity in terms of DNA strand breaks. Chronic inflammation following particle exposure has been proposed to cause secondary (indirect) genotoxicity, whereby ROS are formed as result of metabolic activities of inflammatory cells, leading to damage of DNA in adjacent cells (Evans et al. Citation2017; Møller et al. Citation2010). To further investigate the association between inflammatory and genotoxic responses, in this study, we hypothesized that CBNPs, which are highly inflammogenic, and produce ROS, would cause genotoxicity by particle-generated ROS production or by inflammatory responses, which are both mainly driven by the particle surface area. We compared the hazard of CBNPs by comparing the toxicological responses caused by Printex XE2B (XE2B), with large surface area, and Flammrus101 (FL101), with low surface area. We then compared the toxicity of these two to the benchmark CB Printex 90 (P90), and finally assessed whether deposited dose in terms of surface area or mass is a driver of toxicity in vivo and in vitro. We assessed the toxicity of FL101 and XE2B in vitro by exposing alveolar epithelial cells and macrophages to three doses (low, medium and high), and vehicle, followed by quantification of cell viability, pro-inflammatory responses and DNA strand breaks levels after 6 and 24 h exposure in submerged conditions. We used alveolar epithelial cells (A549) and macrophages (THP-1a) as in vitro pulmonary models from a screening of the literature that indicated these models to be extensively used in respiratory nanoparticle toxicology, thus increasing chances for comparisons. Further, we evaluated the pulmonary toxicity of FL101 and XE2B in mice lungs following single exposure via intra-tracheal instillation with three doses (low, medium and high), and vehicle, following 1-, 28- and 90-days post-exposure. We assessed the inflammatory cell infiltrates in broncho-alveolar lavage (BAL) fluid, histological changes in lung tissue, and DNA strand breaks in BAL cells, lung and liver tissue.
Methods
Carbon black particles
Two carbon black particles (CBNPs) were included in this study; Flammrus101 (FL101) and PrintexXE2B (XE2B). Both CBNPs were gifts from The Grolman Group (Grolman Nordic Speciality Chemicals, Lystrup, Denmark). This study includes also Printex 90 (P90), a gift from Degussa-Hüls (today Evonik), Frankfurt, Germany. Previously published data of P90 inflammogenicity and genotoxicity in cells and mice are included in statistical analyses to compare the toxicity profile of FL101 and XE2B to the benchmark material P90. P90 has been used as reference material in many studies previously performed at the National Research Center for the Working Environment.
Particle characterization
For in vitro assessment, CBNPs were weighed (17–20 mg) in glass vials, to which 5–6 mL of complete cell culture medium (RPMI-1640 containing 10% fetal bovine serum (FBS) and 1% penicillin/streptomycin (P/S)) was added to obtain a final particle suspension of 3.34 mg/mL. The particle suspensions were sonicated with a Branson Sonifier for 16 min, at 10% amplitude, and 10 s impulses, as previously described (Di Ianni, Møller, et al. Citation2021). During sonication, the vials containing nanomaterial suspensions were kept in a water bath with ice. The stock suspensions were then diluted to 10, 40 or 160 μg/mL (2.8, 11.4, and 45.7 μg/cm2) for cell exposure under submerged conditions. Complete cell culture medium was used as vehicle control (not sonicated, reported as 0 μg/mL).
For the in vivo assessment, 3.24 mg/mL of XE2B, FL101 and P90 (1 dose as positive control) were suspended in water containing 2% mouse serum as previously described (Hadrup et al. Citation2017).
In order to aid the interpretation of their toxicity, we characterized CBNPs properties as bulk (specific surface area (SSA)) and when suspended in exposure media (hydrodynamic diameter and in vitro sedimented dose).
The bulk particle size of both FL101 and XE2B were provided by the supplier (95 and 30 nm, respectively), and FL101 size previously confirmed by electron microscopy (Saber, Jacobsen, et al. Citation2012). The size of P90 has been previously reported to be 14 nm, based on information from the supplier and confirmed by electron microscopy (Bourdon, Saber, et al. Citation2012; Saber, Jacobsen, et al. Citation2012). Following particle dispersion in exposure media, we determined the hydrodynamic diameter by dynamic light scattering (DLS), as previously described in detail (Di Ianni, Møller, et al. Citation2021).
The Brunauer, Emmett, and Teller (BET) specific surface area (SSA) was determined by the multi-point method (Braunauer, Emmett, and Teller Citation1938). The analyses were conducted on a QUANTACHROME AUTOSORB- 3 using N2 at 77 Kelvin (QUANTACHROME GmbH & Co. KG Odelzhausen, Germany), as previously described (Saber, Jacobsen, et al. Citation2012).
A-cellular ROS production was quantified by the cell-free version of the 2′,7′ dichlorodihydrofluorescein diacetate (DCFH2-DA) assay, as previously described (Jacobsen et al. Citation2008; Boyles et al. Citation2022; Høgsberg et al. Citation2013).
For the in vitro studies, we determined the effective doses to which cells are exposed during incubation with the materials in submerged conditions. We employed a quantitative method (Di Ianni, Samulin Erdem, et al. Citation2021) that uses thermo-gravimetric analysis coupled to QMS D Aëolos mass spectrometer (Netzsch-Gerätebau GmbH, Selb, Germany)(TGA-MS). Briefly, the method assesses the mass change of a carbonaceous material during a burning program (25–800 °C), and the mass change is calculated in a temperature range where CO2 emission is identified. For the TGA-MS analysis, cells were cultured in 12-well plates (similar as for assessment of toxicity endpoints) and 160 µg/mL of CBNPs were administered to each well. Following 24 h-exposure, the supernatant in each well was removed and cells were scraped off from the wells and pelleted by centrifugation (20,000 g), after which the pellets obtained were transferred into a crucible for the TGA-MS analysis.
In vitro toxicity assessment
Cell cultures
In the in vitro studies, we used human-derived cell lines, namely alveolar epithelial cells, A549, and monocytic cells, THP-1, and tested CBNPs toxicity in these cells exposed under submerged conditions. These models have been extensively used in in vitro assessment of pulmonary toxicity on nanomaterials, thus increasing chances of comparison with literature (Di Ianni, Samulin Erdem, et al. Citation2021; Di Ianni, Møller, et al. Citation2021; Loret et al. Citation2018; Wang et al. Citation2011; Pal et al. Citation2015; Cao et al. Citation2016). In addition, A549 cells and THP-1 cells are used as components of more advanced co-culture models (Loret et al. Citation2016; Hilton et al. Citation2019; Cappellini et al. Citation2020; Di Ianni, Samulin Erdem, et al. Citation2021). Alveolar epithelial cells (A549) and monocytic cells (THP-1) were purchased from the American Type Cell Collection. Both cell lines were cultured in RPMI-1640 cell culture medium supplemented with 10% FBS and 1% P/S. A549 cells were cultured in T75 and T175 culture flasks, trypsinized (0.05% Trypsin-EDTA) and sub-cultured when 70% cell confluence was reached. Monocytic THP-1 cells were cultured in suspension at a cell density of 3 − 9 × 105 cells/mL medium. A549 and THP-1 cells were sub-cultured for up to 15 passages and then discarded. Twenty-four hours prior to exposure, 2 mL of A549 cell suspensions (2 × 105/mL) were seeded in Nunc 12-well plates. Before exposure, the old medium was removed and fresh medium added for the exposure. Monocytic THP-1 cells were diluted to 3 × 105 cells/mL in 2 mL complete cell culture medium (supplemented with 10% FBS) and differentiated into macrophages (THP-1a) with 10 ng/mL of phorbol-12-myristate-13-acetate (PMA), for 48 h (Maeß et al. Citation2014), in 12-well plates, followed by a rest period of 5 hours. Prior to CBNP exposure, the medium was removed and fresh medium added.
Viability
Following 6 or 24 h of cell exposure to CBNPs in submerged conditions, cell medium was discarded and cells (A549 or THP-1a) were washed twice with PBS. Trypsin was added at 200 µL per well, and the plates were incubated for 4 min after which 300 μL of cell culture medium was added. Cells were gently pipetted to obtain a single cell suspension and further detach those cells that were still adherent to the cell culture plate. Cell viability was measured by NucleCounter (ChemoMetec A/S, Allerød, Denmark). We studied effects of CBNPs in cells exposed for 6 and 24 h as these time points worked well in detection of endpoints we have previously investigated (Di Ianni, Samulin Erdem et al., Citation2021; Bornholdt et al. Citation2007).
Pro-inflammatory response
Total RNA was isolated from harvested cell samples with Macherey-Nagel® NucleoSpin 96-well RNA Core Kit (Macherey-Nagel, Düren, Germany), as recommended by the manufacturer. RNA concentration and purity were determined by NanoDrop 2000 C (Thermo-Fisher, Wilmington, USA) according to the manufacturer’s instructions. cDNA was synthesized with Taqman® reverse transcription reagents (Applied Biosystems, USA), as recommended by the manufacturer. The quantitative PCR was performed with ViiA7 Real-Time qPCR (RT-qPCR) system, using Universal Mastermix (Applied Biosystems, Naerum, Denmark) and pre-developed primers for IL-8 (part. no. 4327042 F) and r18S (part. no.4310881E) as reference gene from Applied Biosystems. Further, we used IL-1β (part. no. 4331182) and r18S primers to assess Il1β mRNA levels. The samples were run in triplicate. The mRNA levels were then further normalized to controls (0 μg/mL of tested CBNPs) to adjust for day-to-day variation of the cell culture and assay.
Genotoxicity
DNA strand break levels were quantified by the comet assay, as previously described (Jackson et al. Citation2013; Di Ianni, Samulin Erdem et al. Citation2021). Following 6 or 24 h-cell exposure, cell medium was discarded, and cells (A549 or THP-1a) were washed twice with PBS. Trypsin was added at 200 µL per well, and the plates were incubated for 4 min after which 300 μL of freezing medium (80% FBS and 20% DMSO) was added. Cells were stored at −80 °C. Frozen cell samples were then thawed quickly at 37 °C and suspended in agarose at 37 °C with final agarose concentration of 0.7%. Cells were embedded on 20-well Trevigen CometSlides™ (30 mL per well). Slides were cooled and placed in lysis buffer overnight at 4 °C, after which these were placed in electrophoresis buffer for 40-min alkaline treatment. Electrophoresis was run with 70 mL/min circulation (5%) of the solution for 25 min with applied voltage at 38 V (1.15 V/cm in the whole electrophoresis tank) and current of 294 mA. The slides were neutralized in Tris buffer (2 × 5 min), fixed in ethanol for 5 min and dried on a warm plate at 45 °C for 15 min. Cells on slides were stained in 40 ml/slide bath with SYBR® Green in TE buffer (10 mM Tris–HCl, 1 mM EDTA, pH 7.6) for 30 min, dried at 37 °C for 10 min after which UV-filter and cover slips were placed on slides. DNA damage was quantified using the automated Imstar Pathfinder comet assay system (ImstarDx, Paris, France). The results are presented as averaged % tail of DNA of all cells scored on each Trevigen CometSlide™. All comet assay runs included A549 cells exposed to PBS or 45 µM H2O2 as negative or positive controls, respectively, for the electrophoresis. The DNA strand break levels were 1.8 ± 0.8 and 21.5 ± 2.6% tail DNA for the negative and positive control, respectively.
In vivo
Animals
Female C57BL/6 mice 5–7 weeks old were purchased from Taconic (Ry, Denmark). General procedures for grouping of animals and housing conditions were performed as previously described (Saber, Jacobsen, et al. Citation2012, Danielsen et al. Citation2020). All mice were exposed at 8 weeks of age. The study complied with the EC Directive 86/609/EEC on the use of animals for experiments and they were approved by the Danish ‘Animal Experiments Inspectorate’ (permission 2020-15-0201-00485).
Study design and exposure
Mice were exposed to vehicle or 3 doses of CBNPs to have low, medium or high exposure levels, and followed for 1, 28 or 90 days. We included six mice per dose group, and 11 mice in the vehicle-exposed groups, at each time point. Mice were intra-tracheally instilled with 18, 54 and 162 μg of FL101, or 6, 18 and 54 μg of XE2B. For the latter, a pilot test with three mice exposed to 162 μg was performed prior to the experimental work to assess dose tolerability, given the large surface area of this particle. Due to the resulting large inflammatory response and weight loss in the three mice, we did not pursue assessment of 162 μg of XE2B in the final experimental setup. Nevertheless, we included the data of the inflammatory response in the assessment of CBNP-surface area relationship with inflammatory response. Mice were exposed by intratracheal instillation to 50 µl of sonicated particles suspensions or vehicle as previously described (Jackson et al. Citation2011; Saber, Jacobsen, et al. Citation2012). We also instilled mice (n = 6) with 162 μg of P90 as positive control at all post-exposure time points. Each test material (including vehicle control) was prepared and instilled shortly after. The dose levels (18, 54, and 162 μg) correspond to the estimated pulmonary deposition during 1, 3, and 9 working days at the Danish occupational exposure limit (3.5 mg/m3 CB), based on an inhalation study using P90, reporting 34.8% of the inhaled mass deposits in the pulmonary region, and assuming air inhalation at 1.8 l/h in mice, and 8 hour working days, while ignoring pulmonary clearance (Jackson et al. Citation2012).
We used intratracheal instillation as it allows control of the deposited dose (Bendtsen et al. Citation2020), although inhalation is the gold standard for risk assessment. We have previously demonstrated that intratracheal instillation leads to particle exposure of all lung lobes in mice (Poulsen et al. Citation2016; Mikkelsen et al. Citation2011) and good concordance between inflammation and genotoxicity in rats exposed to two different MWCNT by inhalation or instillation (Gaté et al. Citation2019).
Preparation of cells and tissue from mice
One, 28 and 90 days after intratracheal instillation, the mice were anesthetized with isoflurane and euthanized (Jackson et al. Citation2011). The mice lungs were flushed four times with 0.8 mL of 0.9% sterile saline through the trachea. BAL fluid was kept on ice and the samples were centrifuged at 400 × g at 4 °C for 10 min to recover cells, which were resuspended in 100 mL HAM’s-F12 medium (#31765035; Gibco, Carlsbad, CA) containing 10% FBS. Forty microliters of the BAL fluid was mixed with 160 µL of cell medium containing 10% DMSO and stored at −80 °C for analysis in the comet assay. A piece of liver tissue per mice was frozen in Nunc™ cryotubes in liquid N2 and stored at −80 °C for the comet assay on liver cells. A sample of lung tissue per mouse was preserved in 4% neutral buffered formaldehyde for histological examination. These procedures have been previously described in detail (Saber, Jacobsen, et al. Citation2012; Bengtson et al. Citation2017).
Pulmonary influx of inflammatory cells
Pulmonary influx of inflammatory cells was quantified by differential counting of immune cells in BAL fluid as described (Kyjovska et al. Citation2015). Briefly, 50 mL of the cell resuspension in HAM’s-F12 medium and FBS was transferred to a microscope slide and centrifuged at 1000 rpm (55 x g) for 4 min by use of a Cytofuge 2 (StatSpin, Bie and Berntsen, Rødovre, Denmark). Cells were fixed by the addition of 96% ethanol and incubated with May-Grunwald-Giemsa stain. A total of 200 cells were counted per sample for differentiation. The total number of cells in the resuspension was measured with a NucleoCounter NC-200 (Chemometec, Allerød, Denmark) Live/Dead Assay.
Comet assay
DNA strand breaks levels were quantified by the alkaline comet assay in BAL cells, lung and liver tissue samples as previously described (Bornholdt et al. Citation2007; Saber, Jacobsen, et al. Citation2012, Jackson et al. Citation2013). Briefly, the frozen samples of BAL cells suspended in 10% DMSO were thawed, and approximately 40 mg of frozen lung and liver tissue samples were squeezed through a metal stapler (diameter 0.5 cm, mesh size 0.4 mm) into cold Merchant’s medium (0.14 M NaCl, 1.47 mM KH2PO4, 2.7 mM KCl, 8.1 mM Na2HPO4,10mM Na2EDTA, pH 7.4). All samples were embedded in agarose and transferred to GelBond films, which were placed in lysis buffer overnight. The films were then subjected to alkaline electrophoresis, fixed, and stained for scoring as previously described (Saber, Jacobsen, et al. Citation2012). A negative and a positive assay control were included on each GelBond film, by embedding samples of cryopreserved A549 cells previously exposed to 0 and 45 mM H2O2 for positive control. DNA% tail was 1.5 ± 0.5 and 20.0 ± 1.6 for the negative and positive control, respectively.
Lung histology
Lung specimens fixed in 4% neutral buffered formaldehyde originating from 4 to 6 mice from the vehicle control and from the high-dose groups terminated 28 or 90 days after instillation were paraffin-embedded. Thereafter, sections of 4–6 µm were made and stained with hematoxylin and eosin for histological examination. The tissues were evaluated using a structured scoring sheet for recording diverse histological changes as described earlier (Knudsen et al. Citation2019; Danielsen, Bendtsen et al., Citation2020). In , ‘lymphocytic infiltrates’ are defined as areas of tissue where the density of lymphocytes is higher than background so that the lymphocytes collection has a shape and size. These are usually present around bronchi and blood vessels. The minimum requirement is that they should contain 50 or more lymphocytes. The average of the number of infiltrates and their range are reported. ‘Macrophage aggregates’ are defined as areas of tissue where the density of macrophages is higher than background so that the macrophages can be seen as group. The minimum requirement is that they should contain 5 or more macrophages. The average of the number of infiltrates and their range is reported. The numbers of mice where (any) material could be seen in pulmonary macrophages are also presented in . In addition to these parameters, a number of other parameters including the presence of fibrosis and pulmonary alveolar proteinosis was evaluated.
Table 1. Main physicochemical characteristics of carbon black nanoparticles included in this study.
Statistics
All data on CBNPs toxicity were first assessed for normal distribution by Shapiro test. Normally distributed log-transformed-data on pro-inflammatory response were assessed by one-way ANOVA followed by post-hoc Tukey test for multiple comparisons. We assessed dose-response effects of CBNPs on DNA strand breaks in A549 and THP-1a cells with linear regression analysis with doses as predictor. For the statistical analysis of the BAL fluid cell composition, the data for the vehicle controls instilled with 50 μL sonicated vehicle in the present and the previously published study (2% serum in water in the present study and 10% BAL fluid in saline in the previous studies listed in ) were similar, and were therefore pooled in the statistical analysis (n = 22). All data on CBNPs-induced inflammatory cell infiltrates in BAL fluid were first assessed for normal distribution by Shapiro test. Normally distributed log-transformed-data were assessed by one-way ANOVA followed by post-hoc Tukey test for multiple comparisons. When data were not normally distributed, these were assessed by Kruskal-Wallis non-parametric test followed by multiple pairwise-comparison by using Wilcox test. Statistical significance was tested at the p < 0.05 level. Correlations of CBNPs surface area with inflammatory response in vitro and in vivo were assessed by linear regression analyses with administered (in vitro), or instilled (in vivo), surface area dose as predictor. For the in vivo dataset used in the correlation analysis, the historical in vivo data on P90-induced neutrophil influx were pooled and combined with the data generated in this study (FL101, XE2B and P90 [1 dose only as positive control]).
We assessed the association between inflammatory and DNA damage responses in the cell cultures and lungs by regression analyses, to test the hypothesis of inflammatory-driven genotoxicity (secondary genotoxicity). The models performed take into account that nanoparticle surface area causes ROS and inflammatory responses, leading to DNA damage responses. We assessed the associations in four regression analyses (supplementary Figure S2), namely (M1) SSA and DNA damage, (M2) SSA and IL-8 or neutrophil influx, (M3) IL-8 or neutrophil influx and DNA damage, and (M4) IL-8 or neutrophil influx and DNA damage adjusted by SSA. These models were applied to dataset of A549 and THP-1a cells (24 h post-exposure), and to in vivo dataset, namely BAL cells and lung tissue of mice exposed for 1, 28 and 90 days. The statistical analyses were performed in R (The R Project for Statistical Computing version 3.5.3). The package ggplot2 was implemented in R for plotting of data (Valero-Mora Citation2010).
Results
Characterization
shows the main physical characteristics of FL101 and XE2B, as well as previously reported data for P90. FL101 has a primary particle size of 95 nm and a correspondingly low SAA of 24 m2/g. XE2B has a primary particle size of 30 nm and a very large SSA of 1000 m2/g. In comparison, the smaller P90 has a primary particle size of 14 nm and a SSA of 295 m2/g. The large SSA of XE2B is likely due to its porous structure and therefore the contribution of internal surface area. For the in vitro studies, we assessed the amount of FL101 and XE2B that precipitated onto the cells following 24 h exposure of 160 µg/mL of materials, when these are suspended in RPMI-1640 cell culture medium, by quantifying total carbon content in harvested cells (). The effective deposited doses of FL101 and XE2B resulted to be 69 and 66 µg, respectively, corresponding to 43 and 41%, respectively, of the administered dose.SSA)
Table 2. Effect of carbon black nanoparticle exposure on IL-8 gene induction in A549 and THP-1a cells for administered doses and effective doses quantified by TGA-MS.
A-cellular ROS production was assessed by DCF fluorescence (). XE2B produced the greatest amount of ROS, followed by P90 and FL101. ROS production correlated with the dosed surface area of the CBNPs, and did not correlate with CBNPs mass ().
Figure 1. A-cellular reactive oxygen species (ROS) production of (A) FL101, (B) P90 and (C) XE2B in the concentration range causing ROS steady increase. ROS production poorly correlated with CBNP concentration (D) (p > 0.05), whereas surface area was significantly correlated with ROS production (E) (p < 0.001 and R2:0.75). The lines represent the linear regression of ROS production as function of CBNP (D) concentration or (E) surface area.
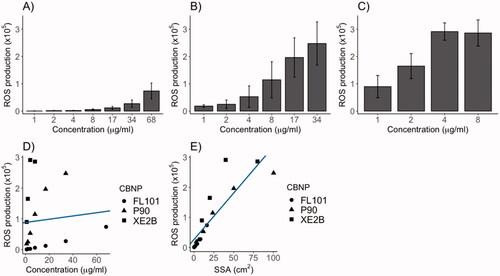
In vitro toxicity assessment
Cell death
We assessed dead/live cell ratio following cell exposure to CBNPs by NucleoCounter NC-200. As shown in supplementary Table S1, no decrease in cell viability was observed in A549 and THP-1a cells following exposure to any of the applied dose levels of FL101 or XE2B, at 6 and 24 h.
Pro-inflammatory response
We quantified pro-inflammatory responses in terms of IL-8 and IL-1β gene expression levels in A549 and THP-1a cells after 6 and 24 h-exposure by RT-qPCR. shows the IL-8 gene induction caused by FL101 and XE2B. In A549, FL101 caused a dose-dependent increase of IL-8 gene expression levels at 24 h post-exposure, with a statistically significant 4 fold-increase in the cells exposed to highest concentration (160 µg/mL), as compared to control cells. XE2B caused a dose-dependent increase of IL-8 gene expression levels in A549 cells at 6 h, which further increased after 24 h to statistically significant 15-fold in cells exposed to the highest concentration (160 µg/mL), with respect to controls. In THP-1a cells, only XE2B caused a dose-dependent increase, with a statistically significant effect (7-fold) observed in cells exposed for 24 h to 160 µg/mL.
Figure 2. Pro-inflammatory response in terms of IL-8 gene expression in (A) A549 and (B) THP-1a cells exposed to FL101 and XE2B for 6 and 24 h. The data represent mean ± SEM of three independent replicates. Stars represent significance at p < 0.05 from ANOVA test of significance (*:p < 0.05:**:p < 0.01).
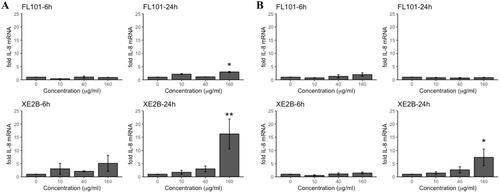
After adjusting the highest administered dose (160 µg/mL) to sedimented doses quantified by TGA-MS (), XE2B remained the strongest inducer of IL-8 gene expression, whereas smaller differences in the ranking were observed between P90 and FL101 (). We also assessed IL-1β gene expression in the two cell lines exposed to FL101, XE2B and P90 (supplementary Figure S1), but found no IL-1β induction in either of the cell lines, at any time point and concentration tested.
Table 3. Effects of carbon black nanoparticle exposure on DNA strand breaks levels (fold) in A549 and THP-1a cells for administered doses and effective doses quantified by TGA-MS.
DNA damage
DNA damage in terms of DNA strand breaks levels was quantified in the A549 and THP-1a cells exposed to FL101 and XE2B (). In THP-1a cells, both CBNPs caused a dose-dependent increase of DNA strand breaks levels, with statistically significant effects in cells exposed to 160 µg/mL. Following adjustment of highest dose (160 µg) by sedimented dose of F101 and XE2B, the ranking of genotoxic potential in the two cell lines did not change ().
Figure 3. DNA damage (% tail) in A549 (A) and THP-1a (B) cells exposed to FL101, P90 and XE2B. The data represent mean values ± SD from three independent replicates, the error bars represent the SD. Stars represent significance at p < 0.05.
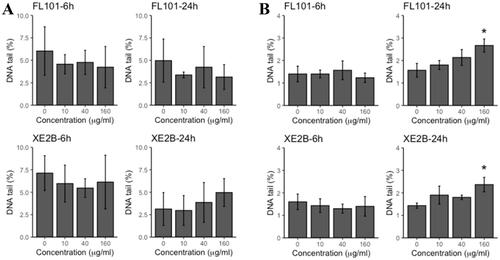
Table 4. Inflammatory cell infiltrates in broncho-alveolar lavage fluid from mice exposed to vehicle and CB nanoparticles; XE2B, FL101 and Printex 90 (P90) (positive control in this study), at day 1, 28 and 90.
Correlation of CBNP dose (mass or surface area) and toxicological responses in cells
In order to assess the correlation between CBNPs surface area and inflammatory responses, we included in the evaluation also data on P90 that were previously reported (Di Ianni, Samulin Erdem et al. Citation2021). As shown in , panel A and B, the dosed surface area of the three CBNPs was significantly and positively correlated with IL-8 gene expression (R2=0.61 for both cell lines).
Figure 4. Correlation of administered CBNP surface area with IL-8 mRNA induction in (A) A549 cells and (B) THP-1a macrophages following 24 h exposure in submerged conditions. Correlations assessed by linear regression analysis with dose and CBNP type as predictor, and IL-8 induction as outcome (p < 0.001 and R2: 0.61 in both A and B). Lines in A and B show trends with SSA dose on a logarithmic scale. C) Correlation of DNA tail (%) and administered dose as surface area in THP-1a cells (p = 0.292 and R2:0.02). (D) Correlation of DNA tail (%) and administered doses as mass in THP-1a cells (p < 0.01 and R2:0.58).
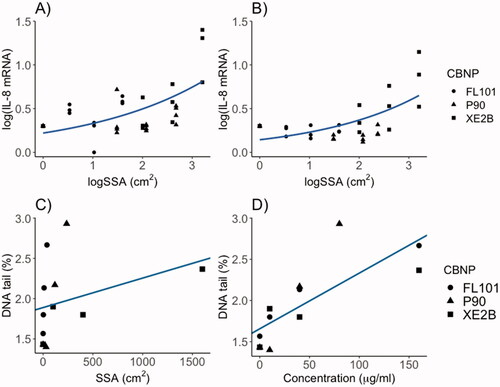
When DNA damage was assessed as function of surface area or mass across the three CBNPs (including also P90 data from Di Ianni, Samulin Erdem et al., Citation2021), mass dose was a stronger predictor of DNA strand break levels in THP-1a cells (R2=0.58; p < 0.01) than surface area dose (R2:0.02; p = 0.29) (, panel C and D).
Assessment of the extent of genotoxicity caused by pro-inflammatory response by regression analyses resulted in a contribution of inflammatory responses to DNA strand break levels of 4% (p > 0.05) in A549 cells, 0.3% (p > 0.05) in THP-1a cells, indicating poor evidence of surface area-caused DNA damage, or inflammation-caused DNA damage (secondary genotoxicity).
In vivo toxicity assessment
Inflammation
shows CBNPs-induced inflammation as quantification of inflammatory cell infiltrates in BAL fluid of mice exposed to vehicle or three dose levels of test materials for 1 and 28 days, and one high dose for 90 days. A single dose (162 µg) of P90 was included as benchmark particle. Both FL101 and XE2B caused dose-dependent influx of neutrophils in BAL fluid. On day 1, XE2B resulted in a more pronounced inflammatory response, with significant ∼8 fold increase in the 54 µg-group (high dose) compared to the negative control, whereas 162 µg (high dose) of FL101 induced a significant 3-fold increase. XE2B-induced neutrophil influx increased 20-fold at day 28 compared to controls, while at this time point neutrophil cell numbers in mice exposed to FL101 had returned to baseline levels. At day 90, a small, yet significantly higher neutrophil influx was observed in the group exposed to high dose of XE2B compared to controls. The strong inflammatory reaction caused by XE2B was observed also in terms of the numbers of lymphocytes in BAL of mice exposed for 28 and 90 days, with ∼25- and 6-fold increase respectively. A small change (2-fold) in the numbers of eosinophils was observed only in lungs of mice exposed to 162 µg of FL101 at day one, and no significant difference was observed in the numbers of macrophages between exposed and control animals. Following intratracheal instillation of P90 (162 µg), the neutrophil influx in BAL fluid was significantly increased in the three time points, with 12-, 21- and 12-fold increase at 1, 28 and 90 days, respectively. P90-exposed mice also had significantly elevated numbers of macrophages and eosinophils in BAL at day 1, and lymphocytes at all assessed time points.
Table 5. Histological data including the numbers of lymphocyte infiltrates and macrophage aggregate separately and together.
As shown in , the inflammatory response of all CBNPs in terms of neutrophil influx in BAL fluid was positively and significantly correlated with CBNP surface area 1-, 28-, and 90-days post-exposure using the present data and previously published data on P90 (Poulsen et al. Citation2016; Kyjovska et al. Citation2015; Bengtson et al. Citation2017; Hadrup et al. Citation2017; Barfod et al. Citation2020; Bendtsen et al. Citation2019; Modrzynska et al. Citation2018; Saber et al. Citation2015; Jacobsen et al. Citation2009; Hadrup et al. Citation2020; Bendtsen et al. Citation2020; Hadrup et al. Citation2021).
Figure 5. Correlation of surface area with the inflammatory responses in terms of neutrophil influx in mice 1 (A), 28 (B) and 90 days post-exposure (C), by linear regression analysis with specific surface area (SSA) as predictor, and neutrophil influx as dependent variable. The data shown include data from the present study as well as previously published studies. D1: R-squared = 0.64 (p < 0.001); D28: R-squared = 0.35 (p < 0.001); D90: R-squared = 0.29(p = 0.06). Study 1: Saber et al. (Citation2015): Study 2: Kyjovska et al. (Citation2015); Study 3: Modrzynska et al. (Citation2018); Study 4: Billing et al. (Citation2020); Study 5: Barfod et al. (Citation2020); Study 6: Hadrup et al. (Citation2021); Study 7: Bourdon, Saber, et al. (Citation2012); Study 8: Poulsen et al. (Citation2016); Study 9: Bengtson et al. (Citation2017); Study 10: Hadrup et al. (Citation2020) and Husain et al. (Citation2015); Study 11: Bendtsen et al. (Citation2019); Study 12: Bendtsen et al. (Citation2020); Study 13: CBNPs tested in the present study; FL101 and XE2B.
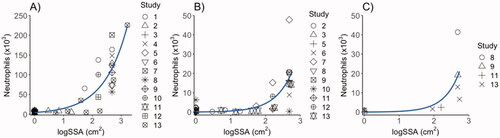
Lung tissue histology
The histological evaluation of the lung tissue at 28 and 90 days post-exposure shows an increased amount of macrophage aggregates and lymphocytes infiltrates in the lungs of mice exposed to XE2B, compared to controls and FL101 ( and ). At post-exposure day 90, presence of material was still observed in XE2B-exposed lung tissue, together with lymphocytic and macrophage infiltrates ( and ). In contrast, in FL101 exposed mice at day 90 after exposure, the material had largely disappeared together with the inflammatory activity. Fibrotic changes in the lungs were not observed for either material.
Figure 6. Histological changes in lung tissue 90 days post-exposure at 20× magnification. (A) Tissue from mouse exposed to a total carbon black surface area of 540 cm2 (corresponding to instilled mass dose of 54 ug) XE2B, (B) tissue from mouse exposed to 39 cm2 (corresponding to 162 ug) FL101. Alveolar walls in A appear widened due to capillary congestion, probably related to the euthanasia.
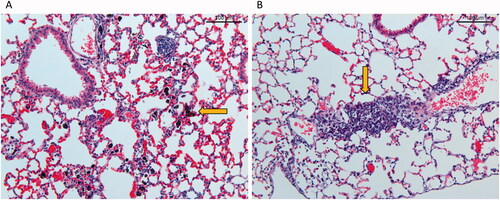
Alveolar walls of mice exposed to XE2B () appear widened due to capillary congestion, probably related to the euthanasia.
DNA damage
DNA damage was assessed by quantification of DNA strand breaks levels in BAL cells, and lung and liver tissue. There was no treatment-related increase in DNA strand break levels in BAL cells, lung and liver tissue, at any time point (). Assessment of the secondary genotoxicity by regression analysis (supplementary Figure S2), with the data generated in this study combined to the historical P90 data (Poulsen et al. Citation2016; Kyjovska et al. Citation2015; Bengtson et al. Citation2017; Hadrup et al. Citation2017; Barfod et al. Citation2020; Bendtsen et al. Citation2019; Modrzynska et al. Citation2018; Saber et al. Citation2015; Jacobsen et al. Citation2009; Hadrup et al. Citation2020; Bendtsen et al. Citation2020; Hadrup et al. Citation2021), indicates a significant contribution of the inflammatory responses to DNA strand break levels in BAL cells of 13% (p < 0.001) at day 1, and 7% (p < 0.001) at day 28 post-exposure.
Discussion
In this study, we compared the pulmonary toxicity of CBNPs with different size and specific surface area in vitro and in vivo. All three materials induced surface-area dependent increase of ROS production in the a-cellular DCFH2-DA assay. XE2B caused the strongest pro-inflammatory response in both cell lines and highest increase of inflammatory cell infiltrates in mouse lungs. Inflammatory responses in vitro and in vivo were positively and significantly correlated to dosed surface area of the CBNPs. Both FL101 and XE2B caused dose-dependent, significant increase of DNA strand breaks levels in THP-1a cells, while no DNA damage was observed in A549 cells, nor in mice BAL cells and lung tissue. We assessed the association between inflammatory response and DNA damage, adjusted by surface area, which indicated ≤13% of the variation in DNA strand breaks levels were explained by the variation in inflammatory responses in the cell cultures and animal lungs, thus indicating poor evidence of secondary genotoxic effects due to CBNPs exposure.
We have quantified the amount of XE2B and FL101 that sedimented onto the cells in the in vitro toxicity assessment, for the highest nominal dose. The TGA-MS method has proven to be a valuable method to quantify the administered doses in vitro that reaches the adherent cells over the exposure time (Di Ianni, Samulin Erdem, et al. Citation2021; Di Ianni, Møller, et al. Citation2021). In vitro dosimetry has been shown to have implications on the interpretation of toxicity data if the sedimentation rate differs between the studied materials (Pal et al. Citation2015). However, we observed similar deposition rate of the two CBNPs in this cell culture medium, which was similar to what we have previously observed for P90 (Di Ianni, Samulin Erdem et al. Citation2021), thus not affecting the comparison of the three CBNPs. The method has been applied to quantify elemental carbon content in carbonaceous materials such as CBNPs, MWCNT, SWCNT, and graphene oxide materials (Wils et al. Citation2021, Di Ianni, Samulin Erdem et al. Citation2021). The TGA-MS method we developed, however, does not distinguish between nanoparticles that get in contact with cells and nanoparticles that are up-taken by cells. Thus, we have not specifically assessed particle uptake. As both cell lines were exposed in the same complete cell culture medium, we have used A549 cells as the model for determination of dose deposition. The deposition of CBNPs might be dependent on medium viscosity, particle size and effective density. That is, the same dose of P90 administered to A549 cells in F12 medium led to smaller particle size and a lower deposited dose (Di Ianni, Samulin Erdem et al. Citation2021).
In vitro, pro-inflammatory responses and DNA damage were assessed at concentrations that did not cause cell death (supplementary Table S1), which is known to otherwise interfere with measurements of endpoints that involve genome stability. We quantified IL-1β and IL-8 gene expression levels as markers of pro-inflammatory responses, which were increased in transcriptomic analyses of lung tissue following nanoparticle deposition (Halappanavar et al. Citation2020). Only IL-8 expression levels were increased in the two cell lines (), whereas no differential gene expression was observed for IL-1β (supplementary Figure S1). We chose to quantify these chemokines at gene expression levels rather than at protein level, as we have previously demonstrated good correlation between gene and protein levels for IL-8 in A549 cells (Bornholdt et al. Citation2007). IL-8 expression had larger dynamic range than protein secretion (Bornholdt et al. Citation2007) and therefore we have used gene expression in recent studies (Di Ianni, Samulin Erdem, et al. Citation2021; Di Ianni, Møller, et al. Citation2021) to increase the response gradient.
CBNPs surface area dose predicted IL-8 gene expression in A549 cells and THP-1a cells across particle types (), in agreement with what has been found in vivo, where retained particle surface area is a strong predictor of pulmonary inflammation (Cosnier et al. Citation2021; Elder et al. Citation2005, Baisch et al. Citation2014; Tran et al. Citation2000; Stoeger et al. Citation2006; Schmid and Stoeger Citation2016). To the best of our knowledge, there is currently no study assessing pulmonary toxicity of XE2B, and a previous study that compared the toxicity of FL101 and P90 in vitro, reported FL101 to cause stronger pro-inflammatory response in murine macrophages (Peebles et al. Citation2011). Similarly, FL101 induced stronger IL-8 gene expression in A549 cells compared to P90 (Di Ianni, Samulin Erdem, et al. Citation2021), however neither FL101 nor P90 caused inflammatory responses in THP-1a cells. The results on lung cell inflammatory response by P90 in vitro are sparse. Burgum and coworkers found significantly elevated levels of IL-8 protein secretion in supernatant of epithelial cells (16HBE14o) exposed to P90 for 6 h (Burgum et al. Citation2021). Zhao and coworkers found increased levels of IL-1β in bronchial epithelial cells (16HBE) exposed to CB (size reported; 200–400 nm, surface area; 74.85 m2/g), suggesting the inflammatory response to be mediated by NLRP3 inflammasome (Zhou et al. Citation2020), in contrast to other studies that did not report such induction of IL-1β (Boland, Hussain, and Baeza-Squiban Citation2014). The incongruent results might reflect the difference in particles tested (different size, surface area, impurities), as well as preparation of particles in different exposure media, with consequent different sedimented doses. Based on the larger IL-8 gene induction in A549 than THP-1a cells, and, given that we used the same exposure medium for both cell lines thus resulting in similar CBNPs deposition rate, A549 cells might be slightly more sensitive in terms of pro-inflammatory response to CBNPs exposure than THP-1a cells. The latter are phagocytic cells and might tolerate higher doses of spherical particles, as shown in a previous study where increased levels of TNF-α, IL-6, IL-1β and IL-8 proteins were observed in THP-1 cells exposed to high concentrations of nanosized CB (540 µg/mL) (Sahu, Kannan, and Vijayaraghavan Citation2014). These results might suggest that spherical particles are less inflammogenic in macrophages as compared to high-aspect ratio engineered nanomaterials like multi-walled carbon nanotubes (long and rigid) and nanoclays, which resulted in much larger pro-inflammatory responses potentially due to frustrated phagocytosis (Di Ianni, Samulin Erdem, et al. Citation2021; Di Ianni, Møller, et al. Citation2021; Murphy et al. Citation2012).
We assessed DNA damage in cells exposed to XE2B and FL101 by means of DNA strand break levels in the comet assay. DNA damage is an intermediary step in the mechanism leading to mutations and ultimately cancer, therefore is an important biomarker of genotoxicity. We observed dose-dependent increase in DNA strand breaks levels following exposure for 24 h in THP-1a cells, although the magnitude of effect was relatively low (approximately 1.5–2.5%Tail DNA in controls and high concentration, respectively). It has been previously postulated that some engineered nanomaterials may interfere with the comet assay (Karlsson et al. Citation2015). We have tested this hypothesis in a previous study (Di Ianni, Samulin Erdem, et al. Citation2021) with a set of carbonaceous materials with large surface area (similar to CB P90), but did not observe any indications of interference. Previous studies on P90 have reported increased level of DNA strand breaks (Jacobsen et al. Citation2007; Mroz et al. Citation2007, Citation2008; di Giorgio et al. Citation2011; Vesterdal et al. Citation2014; Frikke-Schmidt et al. Citation2011; Di Ianni, Samulin Erdem, et al. Citation2021) or unaltered effects (Migliore et al. Citation2010; Gerloff et al. Citation2009; Bengtson et al. Citation2016). We have hypothesized that CBNPs-induced genotoxicity might be explained by the increased ROS production (Danielsen et al. Citation2011; Modrzynska et al. Citation2018; Jacobsen et al. Citation2011), as well as by inflammatory responses leading to secondary genotoxicity (Burgum et al. Citation2021). In the current study, dosed mass was a better predictor of DNA strand break levels in THP-1a cells than dosed particle surface area. Only 4% (A549 cells) or 0.3% (THP-1a cells) of the variation in the DNA strand breaks levels could be explained by the variation in the inflammatory response. This indicates little evidence of secondary genotoxicity in these experimental models. It could be argued that these cancer cell lines have genomic instability and conclusions on genotoxicity should be cautious. To prevent cell genomic changes, we cultured these cell lines and used in toxicity testing for relatively few passages. We have previously used these models to assess concordance of genotoxic effects between in vitro and in vivo models, and have shown that THP-1 cells might better predict genotoxic response than A549 cells (Di Ianni, Samulin Erdem, et al. Citation2021; Di Ianni, Møller, et al. Citation2021).
We assessed inflammation in mice following pulmonary exposure by assessing the composition of inflammatory cell infiltrates in BAL fluid. The molecular mechanism of CBNP-induced inflammation is not completely clear. In mice, CBNP-induced neutrophil influx was unaffected in TLR4−/− and in TLR2−/− mice, whereas lipopolysaccharide (LPS)-induced inflammation was reduced in TLR4−/− mice, suggesting that the initiation of inflammation differs between LPS and CBNPs (Danielsen et al. Citation2021). In addition to the observed neutrophil influx, XE2B exposure also increased the number of lymphocytes at 28 and 90 days post-exposure, which is similar to what we have observed in earlier studies for P90 (Bendtsen et al. Citation2019; Kyjovska et al. Citation2015). Inflammation, in terms of neutrophil influx, was strongly correlated to the SSA of CBNPs, similarly to what was previously shown for other spherical carbon-particles (diesel exhaust particles, and airport emission particles), TiO2 and other metal oxide nanoparticles, high-aspect ratio nanoparticles (carbon nanotube and halloysite tubes), and for plates (nanoclays) (Bendtsen et al. Citation2019; Danielsen, Knudsen, et al. Citation2021; Poulsen et al. Citation2016; Hadrup et al. Citation2021; Barfod et al. Citation2020; Di Ianni et al. Citation2020; Stoeger et al. Citation2006; Schmid and Stoeger Citation2016; Cosnier et al. Citation2021). The CBNP-induced inflammation may have a threshold at 30–40 cm2, which is comparable to the 20 cm2 reported previously. XE2B has a larger SSA than P90 and FL101, although XE2B has a larger particle than P90. This is due to porosity and therefore large internal surface area. As shown in , XE2B seems to cause an inflammatory response that deviates slightly from that of P90 and FL101, suggesting that nanoparticle external surface area is a stronger driver of the inflammatory response, as compared to internal surface area of porous particles. A similar trend was observed previously with high porosity silica particles (Hadrup et al. Citation2021). Histopathological evaluation confirmed particle presence in lung tissue at day 28 for both FL101 and XE2B, whereas at day 90 post-exposure, FL101 seemed to be less retained as compared to XE2B, suggesting that the clearance of the larger FL101 occurs faster than clearance of the smaller XE2B in concordance with previous findings (Elder et al. Citation2005).
DNA damage was quantified in terms of DNA strand breaks levels determined by alkaline version of the comet assay in BAL cells, lung and liver tissue. Following intratracheal instillation of mice, we observed a dose dependent increase in mice after one day exposure to high doses of XE2B and FL101, yet the effects were statistically non-significant. No changes were observed in lung and liver tissue at any dose and time points for XE2B, FL101 and P90 (only one dose- 162 µg). We have previously observed P90 to induce significantly increased levels of DNA strand breaks in BAL cells of mice exposed to lower doses (0.67 and 2 µg) (Kyjovska et al. Citation2015) as well as at 18, 54 and 162 µg (Bourdon, Saber, et al. Citation2012). However, across 10 previously published studies of P90 or where P90 was included as a benchmark material, increased DNA strand break levels were reported for one or more dose levels at one or more time points for BAL cells in 5/9 studies, for lung tissue in 4/9 studies and for liver tissue in 4/8 studies (Bourdon, Saber, et al. Citation2012; Kyjovska et al. Citation2015; Husain et al. Citation2015; Modrzynska et al. Citation2018; Bengtson et al. Citation2017; Bendtsen et al. Citation2019, Citation2020; Poulsen et al. Citation2016). We have previously discussed that particle-induced DNA stand breaks only result in 50 to 100% increase in DNA strand break levels in vivo, and this may be close to the detection level of the assay due to high inter-animal variation (Kyjovska et al. Citation2015). This may be the reason why statistically significantly increased levels of DNA damage are only observed in some studies (Kyjovska et al. Citation2015; Bourdon, Saber, et al. Citation2012; Bengtson et al. Citation2017; Modrzynska et al. Citation2018; Bendtsen et al. Citation2020; Saber et al. Citation2005; Husain et al. Citation2015) and not in others (Barfod et al. Citation2020; Bendtsen et al. Citation2019; Poulsen et al. Citation2016). Further, the apparent lack of dose-response relationship may reflect a dose-dependent increase at a low dose levels reaching a plateau at higher dose levels (Kyjovska et al. Citation2015). This lack of dose-response relationship was observed previously in mice instilled with P90 (Bourdon, Saber, et al. Citation2012). The alkali-labile sites and DNA strand breaks detected by the comet assay may be repaired by DNA repair enzymes or result in mutations if left unrepaired. While we employed the comet assay in the in vitro and in vivo testing to assess genotoxicity of these materials and compare the response to studies we performed historically in our group, future studies could include other biomarkers of genotoxicity such as micronuclei assay for further understanding of the genotoxic properties of these materials.
To test the hypothesis of inflammation-driven genotoxicity, the data generated in this study were combined with the historical data on P90, and the associations were tested in regression analyses (supplementary Figure S2). This showed that 13% and 7% of the variation in the DNA stand breaks levels in BAL cells at day 1 and 28, respectively, could be explained by the inflammatory response in terms of neutrophil influx. The same analysis on DNA strand breaks in lung tissue indicated that less than 0.8% of the variation could be explained by inflammation. In vivo evidence of primary genotoxicity was found in a previous study, where CB, TiO2 and CeO2 NPs translocated from lung to liver following pulmonary exposure in mice (Modrzynska et al. Citation2018). Collectively, the results indicate poor evidence of secondary genotoxic effects in vivo.
Conclusions
In conclusion, we have assessed CBNP toxicity in terms of inflammatory and genotoxic responses for CBNPs of different sizes in vivo and in vitro. Dosed surface area was a strong predictor of pro-inflammatory response in vitro and inflammatory response in vivo. In THP-1a cells, dose-dependent genotoxicity in terms of DNA strand break levels was observed and the level of genotoxicity was predicted by CBNPs mass rather than surface area. The observed lack of correlation between CBNP surface area and genotoxicity provides little evidence for inflammation-driven genotoxicity in vivo and in vitro.
Supplemental Material
Download MS Word (61 KB)Supplemental Material
Download MS Power Point (43.8 KB)Acknowledgement
The authors gratefully acknowledge the excellent technical assistance of Michael Guldbrandsen, Eva Terrida, Yasmin Akhtar, Noor Irmam and Anne Abildtrup.
Disclosure statement
No potential conflict of interest was reported by the author(s).
Data availability statement
Data are available upon reasonable request.
Additional information
Funding
References
- Baisch, Brittany L., Nancy M. Corson, Pamela Wade-Mercer, Robert Gelein, Andrea J. Kennell, Günter Oberdörster, and Alison Elder. 2014. “Equivalent Titanium Dioxide Nanoparticle Deposition by Intratracheal Instillation and Whole Body Inhalation: The Effect of Dose Rate on Acute Respiratory Tract Inflammation.” Particle and Fibre Toxicology 11: 5. doi:10.1186/1743-8977-11-5..
- Barfod, Kenneth Klingenberg, Katja Maria Bendtsen, Trine Berthing, Antti Joonas Koivisto, Sarah Søs Poulsen, Ester Segal, Eveline Verleysen, et al. 2020. “Increased Surface Area of Halloysite Nanotubes Due to Surface Modification Predicts Lung Inflammation and Acute Phase Response after Pulmonary Exposure in Mice.” Environmental Toxicology and Pharmacology 73: 103266. (July 2019): doi:10.1016/j.etap.2019.103266.
- Bartek, Jiri, Martin Mistrik, and Jirina Bartkova. 2010. “Long-Distance Inflammatory and Genotoxic Impact of Cancer in Vivo.” Proceedings of the National Academy of Sciences of the United States of America 107 (42): 17861–17862. doi:10.1073/pnas.1013093107.
- Bendtsen, Katja Maria, Anders Brostrøm, Antti Joonas Koivisto, Ismo Koponen, Trine Berthing, Nicolas Bertram, Kirsten Inga Kling, et al. 2019. “Airport Emission Particles: Exposure Characterization and Toxicity following Intratracheal Instillation in Mice.” Particle and Fibre Toxicology 16 (1): 23–23. doi:10.1186/s12989-019-0305-5.
- Bendtsen, Katja Maria, Louise Gren, Vilhelm Berg Malmborg, Chandra Shukla, Martin Tunér, Yona J. Essig, Annette M. Krais, et al. 2020. “Particle Characterization and Toxicity in C57BL/6 Mice Following Instillation of Five Different Diesel Exhaust Particles Designed to Differ in Physicochemical Properties.” Particle and Fibre Toxicology 17: 38. doi:10.1186/s12989-020-00369-9.
- Bengtson, S., K. Kling, A. M. Madsen, A. W. Noergaard, N. R. Jacobsen, P. A. Clausen, B. Alonso, et al. 2016. “No Cytotoxicity or Genotoxicity of Graphene and Graphene Oxide in Murine Lung Epithelial FE1 Cells in Vitro.” Environmental and Molecular Mutagenesis 57 (6): 469–482. doi:10.1002/em.22017.
- Bengtson, Stefan, Kristina B. Knudsen, Zdenka O. Kyjovska, Trine Berthing, Vidar Skaug, Marcus Levin, Ismo K. Koponen, et al. 2017. “Differences in Inflammation and Acute Phase Response but Similar Genotoxicity in Mice following Pulmonary Exposure to Graphene Oxide and Reduced Graphene Oxide.” PLoS One 12 (6): e0178355. doi:10.1371/journal.pone.0178355.
- Billing, A. M., K. B. Knudsen, A. J. Chetwynd, L. A. Ellis, S. V. Y. Tang, T. Berthing, H. Wallin, I. Lynch, U. Vogel, and F. Kjeldsen. 2020. “Fast and Robust Proteome Screening Platform Identifies Neutrophil Extracellular Trap Formation in the Lung in Response to Cobalt Ferrite Nanoparticles.” ACS Nano 14 (4): 4096–4110. doi:10.1021/acsnano.9b08818.
- Boland, Sonja, Salik Hussain, and Armelle Baeza-Squiban. 2014. “Carbon Black and Titanium Dioxide Nanoparticles Induce Distinct Molecular Mechanisms of Toxicity.” Wiley Interdisciplinary Reviews. Nanomedicine and Nanobiotechnology 6 (6): 641–652. doi:10.1002/wnan.1302.
- Bornholdt, Jette, Anne T. Saber, Anoop K. Sharma, Kai Savolainen, Ulla Vogel, and Håkan Wallin. 2007. “Inflammatory Response and Genotoxicity of Seven Wood Dusts in the Human Epithelial Cell Line A549.” Mutation Research 632 (1–2): 78–88. doi:10.1016/j.mrgentox.2007.04.016.
- Bourdon, Julie A., Andrew Williams, Byron Kuo, Ivy Moffat, Paul A. White, Sabina Halappanavar, Ulla Vogel, Håkan Wallin, and Carole L. Yauk. 2013. “Gene Expression Profiling to Identify Potentially Relevant Disease Outcomes and Support Human Health Risk Assessment for Carbon Black Nanoparticle Exposure.” Toxicology 303: 83–93. doi:10.1016/j.tox.2012.10.014.
- Bourdon, Julie A., Anne T. Saber, Nicklas R. Jacobsen, Keld A. Jensen, Anne M. Madsen, Jacob S. Lamson, Håkan Wallin, et al. 2012. “Carbon Black Nanoparticle Instillation Induces Sustained Inflammation and Genotoxicity in Mouse Lung and Liver.” Particle and Fibre Toxicology 9 (1): 5. doi:10.1186/1743-8977-9-5.
- Bourdon, Julie A., Sabina Halappanavar, Anne T. Saber, Nicklas R. Jacobsen, Andrew Williams, Håkan Wallin, Ulla Vogel, and Carole L. Yauk. 2012. “Hepatic and Pulmonary Toxicogenomic Profiles in Mice Intratracheally Instilled with Carbon Black Nanoparticles Reveal Pulmonary Inflammation, Acute Phase Response, and Alterations in Lipid Homeostasis.” Toxicological Sciences 127 (2): 474–484. doi:10.1093/toxsci/kfs119.
- Boyles, Matthew, Fiona Murphy, William Mueller, Wendel Wohlleben, Nicklas Raun Jacobsen, Hedwig Braakhuis, Anna Giusti, and Vicki Stone. 2022. “Development of a Standard Operating Procedure for the DCFH2-DA Acellular Assessment of Reactive Oxygen Species Produced by Nanomaterials.” Toxicology Mechanisms and Methods 32 (6): 439–452. doi:10.1080/15376516.2022.2029656.
- Boyles, M., F. Murphy, W. Mueller, W. Wohlleben, N. R. Jacobsen, H. Braakhuis, A. Giusti, and V. Stone. 2022. “Development of a Standard Operating Procedure for the DCFH2-DA Acellular Assessment of Reactive Oxygen Species Produced by Nanomaterials.” Toxicology Mechanisms and Methods 32 (6): 439–452. doi:10.1080/15376516.2022.2029656.
- Brunauer, Stephen, P. H. Emmett, and Edward Teller. 1938. “Adsorption of Gases in Multimolecular Layers.” Journal of the American Chemical Society 60 (2): 309–319. doi:10.1021/ja01269a023.
- Burgum, Michael J., Martin J. D. Clift, Stephen J. Evans, Nicole Hondow, Mark Miller, Sandra Bustamante Lopez, Adam Williams, Afshin Tarat, Gareth J. Jenkins, and Shareen H. Doak. 2021. “In Vitro Primary-Indirect Genotoxicity in Bronchial Epithelial Cells Promoted by Industrially Relevant Few-Layer Graphene.” Small 17 (15): 2002511–2002551. doi:10.1002/smll.202002551.
- Cao, Yi, Martin Roursgaard, Nicklas Raun Jacobsen, Peter Møller, and Steffen Loft. 2016. “Monocyte Adhesion Induced by Multi-Walled Carbon Nanotubes and Palmitic Acid in Endothelial Cells and Alveolar-Endothelial Co-cultures.” Nanotoxicology 10 (2): 235–244. doi:10.3109/17435390.2015.1048325.
- Cappellini, Francesca, Sebastiano Di Bucchianico, Venkatanaidu Karri, Siiri Latvala, Maria Malmlöf, Maria Kippler, Karine Elihn, et al. 2020. “Dry Generation of CeO 2 Nanoparticles and Deposition onto a Co-Culture of A549 and THP-1 Cells in Air-Liquid Interface — Dosimetry Considerations and Comparison to Submerged Exposure.” Nanomaterials 10 (4): 618. doi:10.3390/nano10040618.
- Chaudhuri, Ishrat, Claudia Fruijtier-Pölloth, Yufanyi Ngiewih, and Len Levy. 2018. “Evaluating the Evidence on Genotoxicity and Reproductive Toxicity of Carbon Black: A Critical Review.” Critical Reviews in Toxicology 48 (2): 143–169. doi:10.1080/10408444.2017.1391746.
- Cosnier, Frédéric, Carole Seidel, Sarah Valentino, Otmar Schmid, Sébastien Bau, Ulla Vogel, Jérôme Devoy, and Laurent Gaté. 2021. “Retained Particle Surface Area Dose Drives Inflammation in Rat Lungs following Acute, Subacute, and Subchronic Inhalation of Nanomaterials.” Particle and Fibre Toxicology 18 (1): 29. doi:10.1186/s12989-021-00419-w.
- Danielsen, Pernille Høgh, Katja Maria Bendtsen, Kristina Bram Knudsen, Sarah Søs Poulsen, Tobias Stoeger, and Ulla Vogel. 2021. “Nanomaterial-and Shape-Dependency of TLR2 and TLR4 Mediated Signaling Following Pulmonary Exposure to Carbonaceous Nanomaterials in Mice.” Particle and Fibre Toxicology 18: 40. doi:10.1186/s12989-021-00432-z.
- Danielsen, Pernille Høgh, Kristina Bram Knudsen, Janez Štrancar, Polona Umek, Tilen Koklič, Maja Garvas, Esa Vanhala, et al. 2020. “Effects of Physicochemical Properties of TiO2 Nanomaterials for Pulmonary Inflammation, Acute Phase Response and Alveolar Proteinosis in Intratracheally Exposed Mice.” Toxicology and Applied Pharmacology 386: 114830. doi:10.1016/j.taap.2019.114830.
- Danielsen, Pernille Høgh, Peter Møller, Keld Alstrup Jensen, Anoop Kumar Sharma, Håkan Wallin, Rossana Bossi, Herman Autrup, et al. 2011. “Oxidative Stress, DNA Damage, and Inflammation Induced by Ambient Air and Wood Smoke Particulate Matter in Human A549 and THP-1 Cell Lines.” Chemical Research in Toxicology 24 (2): 168–184. doi:10.1021/tx100407m.
- Dell, Linda D., Alexa E. Gallagher, Lori Crawford, Rachael M. Jones, and Kenneth A. Mundt. 2015. “Cohort Study of Carbon Black Exposure and Risk of Malignant and Nonmalignant Respiratory Disease Mortality in the US Carbon Black Industry.” Journal of Occupational & Environmental Medicine 57 (9): 984–997. doi:10.1097/JOM.0000000000000511.
- Di Giorgio, M. L., S. Di Bucchianico, A. M. Ragnelli, P. Aimola, S. Santucci, and A. Poma. 2011. “Effects of Single and Multi Walled Carbon Nanotubes on Macrophages: Cyto and Genotoxicity and Electron Microscopy.” Mutation Research 722 (1): 20–31. doi:10.1016/j.mrgentox.2011.02.008.
- Di Ianni, Emilio, Johanna Samulin Erdem, Peter Møller, Nicklas Mønster Sahlgren, Sarah Søs Poulsen, Kristina Bram Knudsen, Shan Zienolddiny, et al. 2021. “In Vitro-in Vivo Correlations of Pulmonary Inflammogenicity and Genotoxicity of MWCNT.” Particle and Fibre Toxicology 18 (1): 1–16. doi:10.1186/s12989-021-00413-2.
- Di Ianni, Emilio, Peter Møller, Alicja Mortensen, Józef Szarek, Per Axel Clausen, Anne Thoustrup Saber, Ulla Vogel, and Nicklas Raun Jacobsen. 2020. “Organomodified Nanoclays Induce Less Inflammation, Acute Phase Response, and Genotoxicity than Pristine Nanoclays in Mice Lungs.” Nanotoxicology 14 (7): 869–892. doi:10.1080/17435390.2020.1771786.
- Di Ianni, Emilio, Peter Møller, Ulla Birgitte Vogel, and Nicklas Raun Jacobsen. 2021. “Pro-Inflammatory Response and Genotoxicity Caused by Clay and Graphene Nanomaterials in A549 and THP-1 Cells.” Mutation Research. Genetic Toxicology and Environmental Mutagenesis 872: 503405. doi:10.1016/j.mrgentox.2021.503405.
- Donaldson, Ken., Craig A. Poland, and Roel P. F. Schins. 2010. “Possible Genotoxic Mechanisms of Nanoparticles: Criteria for Improved Test Strategies.” Nanotoxicology 4 (4): 414–420. doi:10.3109/17435390.2010.482751.
- Elder, Alison, Robert Gelein, Jacob N. Finkelstein, Kevin E. Driscoll, Jack Harkema, and Günter Oberdörster. 2005. “Effects of Subchronically Inhaled Carbon Black in Three Species. I. Retention Kinetics, Lung Inflammation, and Histopathology.” Toxicological Sciences : An Official Journal of the Society of Toxicology 88 (2): 614–629. doi:10.1093/toxsci/kfi327.
- Evans, Stephen J., Martin J. D. Clift, Neenu Singh, John W. Wills, Nicole Hondow, Thomas S. Wilkinson, Michael J. Burgum, et al. 2019. “In Vitro Detection of in Vitro Secondary Mechanisms of Genotoxicity Induced by Engineered Nanomaterials.” Particle and Fibre Toxicology 16 (1): 8–13. doi:10.1186/s12989-019-0291-7.
- Evans, Stephen J., Martin J. D. Clift, Neenu Singh, Jefferson De Oliveira Mallia, Michael Burgum, John W. Wills, Thomas S. Wilkinson, Gareth J. S. Jenkins, and Shareen H. Doak. 2017. “Critical Review of the Current and Future Challenges Associated With Advanced in Vitro Systems towards the Study of Nanoparticle (Secondary) Genotoxicity.” Mutagenesis 32: 233–241. doi:10.1093/mutage/gew054.
- Frikke-Schmidt, H., M. Roursgaard, J. Lykkesfeldt, S. Loft, J. K. Nøjgaard, and P. Møller. 2011. “Effect of Vitamin C and Iron Chelation on Diesel Exhaust Particle and Carbon Black Induced Oxidative Damage and Cell Adhesion Molecule Expression in Human Endothelial Cells.” Toxicology Letters. 203 (3): 181–189. doi:10.1016/j.toxlet.2011.03.011.
- Gaté, Laurent, Kristina Bram Knudsen, Carole Seidel, Trine Berthing, Laëtitia Chézeau, Nicklas Raun Jacobsen, Sarah Valentino, et al. 2019. “Pulmonary Toxicity of Two Different Multi-Walled Carbon Nanotubes in Rat: Comparison Between Intratracheal Instillation and Inhalation Exposure.” Toxicology and Applied Pharmacology 375: 17–31. doi:10.1016/j.taap.2019.05.001..
- Gerloff, Kirsten, Catrin Albrecht, Agnes W. Boots, Irmgard Förster, and Roel P. F. Schins. 2009. “Cytotoxicity and Oxidative DNA Damage by Nanoparticles in Human Intestinal Caco-2 Cells.” Nanotoxicology 3 (4): 355–364. doi:10.3109/17435390903276933.
- Greene, Mark H., Robert N. Hoover, Raymond L. Eck, and Joseph F. Fraumeni. 1979. “Cancer Mortality among Printing Plant Workers.” Environmental Research 20 (1): 66–73. doi:10.1016/0013-9351(79)90085-9.
- Hadrup, Niels, Anne T. Saber, Zdenka O. Kyjovska, Nicklas R. Jacobsen, Minnamari Vippola, Essi Sarlin, Yaobo Ding, et al. 2020. “Pulmonary Toxicity of Fe2O3, ZnFe2O4, NiFe2O4 and NiZnFe4O8 Nanomaterials: Inflammation and DNA Strand Breaks.” Environmental Toxicology and Pharmacology 74: 103303. doi:10.1016/j.etap.2019.103303.
- Hadrup, Niels, Kukka Aimonen, Marit Ilves, Hanna Lindberg, Rambabu Atluri, Nicklas M. Sahlgren, Nicklas R. Jacobsen, et al. 2021. “Pulmonary Toxicity of Synthetic Amorphous Silica–Effects of Porosity and Copper Oxide Doping.” Nanotoxicology 15 (1): 96–113. doi:10.1080/17435390.2020.1842932.
- Hadrup, Niels, Stefan Bengtson, Nicklas R. Jacobsen, Petra Jackson, Marek Nocun, Anne T. Saber, Keld A. Jensen, Håkan Wallin, and Ulla Vogel. 2017. “Influence of Dispersion Medium on Nanomaterial-Induced Pulmonary Inflammation and DNA Strand Breaks: Investigation of Carbon Black, Carbon Nanotubes and Three Titanium Dioxide Nanoparticles.” Mutagenesis 32 (6): 581–597. doi:10.1093/mutage/gex042.
- Halappanavar, Sabina, Sybille Van Den Brule, Penny Nymark, Laurent Gaté, Carole Seidel, Sarah Valentino, Vadim Zhernovkov, et al. 2020. “Adverse Outcome Pathways as a Tool for the Design of Testing Strategies to Support the Safety Assessment of Emerging Advanced Materials at the Nanoscale.” Particle and Fibre Toxicology 17 (1): 16. doi:10.1186/s12989-020-00344-4.
- Hilton, G., H. Barosova, A. Petri-Fink, B. Rothen-Rutishauser, and M. Bereman. 2019. “Leveraging Proteomics to Compare Submerged Versus Air-Liquid Interface Carbon Nanotube Exposure to a 3D Lung Cell Model.” Toxicology In Vitro : An International Journal Published in Association with BIBRA 54: 58–66. doi:10.1016/j.tiv.2018.09.010.
- Høgsberg, Trine, Nicklas Raun Jacobsen, Per Axel Clausen, and Jørgen Serup. 2013. “Black Tattoo Inks Induce Reactive Oxygen Species Production Correlating with Aggregation of Pigment Nanoparticles and Product Brand but Not with the Polycyclic Aromatic Hydrocarbon Content.” Experimental Dermatology 22 (7): 464–469. doi:10.1111/exd.12178.
- Husain, Mainul, Zdenka O. Kyjovska, Julie Bourdon-Lacombe, Anne T. Saber, Keld A. Jensen, Nicklas R. Jacobsen, Andrew Williams, et al. 2015. “Carbon Black Nanoparticles Induce Biphasic Gene Expression Changes Associated with Inflammatory Responses in the Lungs of C57BL/6 Mice following a Single Intratracheal Instillation.” Toxicology and Applied Pharmacology 289 (3): 573–588. doi:10.1016/J.TAAP.2015.11.003.
- IARC. 2010. “IARC Monographs on the Evaluation of Carcinogenic Risks to Humans.” IARC Monographs on the Evaluation of Carcinogenic Risks to Humans 93: 9–38. doi:10.1136/jcp.48.7.691-a.
- Jackson, P., K. S. Hougaard, A. M. Boisen, N. R. Jacobsen, K. A. Jensen, P. Møller, G. Brunborg, et al. 2012. “Pulmonary Exposure to Carbon Black by Inhalation or Instillation in Pregnant Mice: Effects on Liver DNA Strand Breaks in Dams and Offspring.” Nanotoxicology 6 (5): 486–500. doi:10.3109/17435390.2011.587902.
- Jackson, Petra, Lourdes M. Pedersen, Zdenka O. Kyjovska, Nicklas R. Jacobsen, Anne T. Saber, Karin S. Hougaard, Ulla Vogel, and Håkan Wallin. 2013. “Validation of Freezing Tissues and Cells for Analysis of DNA Strand Break Levels by Comet Assay.” Mutagenesis 28 (6): 699–707. doi:10.1093/mutage/get049.
- Jackson, Petra, Søren P. Lund, Gitte Kristiansen, Ole Andersen, Ulla Vogel, Håkan Wallin, and Karin S. Hougaard. 2011. “An Experimental Protocol for Maternal Pulmonary Exposure in Developmental Toxicology.” Basic & Clinical Pharmacology & Toxicology 108 (3): 202–207. doi:10.1111/j.1742-7843.2010.00644.x.
- Jacobsen, Nicklas Raun, Anne Thoustrup Saber, Paul White, Peter Møller, Giulio Pojana, Ulla Vogel, Steffen Loft, et al. 2007. “Increased Mutant Frequency by Carbon Black, but Not Quartz, in the lacZ and cII Transgenes of Muta Mouse Lung Epithelial Cells.” Environmental and Molecular Mutagenesis 48 (6): 451–461. doi:10.1002/em.20300.
- Jacobsen, Nicklas Raun, Giulio Pojana, Paul White, Peter Møller, Corey Alexander Cohn, Karen Smith Korsholm, Ulla Vogel, Antonio Marcomini, Steffen Loft, and Håkan Wallin. 2008. “Genotoxicity, Cytotoxicity, and Reactive Oxygen Species Induced by Single-Walled Carbon Nanotubes and C60 Fullerenes in the FE1-MutaTMmouse Lung Epithelial Cells.” Environmental and Molecular Mutagenesis 49 (6): 476–487. doi:10.1002/em.20406.
- Jacobsen, Nicklas Raun, Paul A. White, John Gingerich, Peter Møller, Anne Thoustrup Saber, George R. Douglas, Ulla Vogel, and Håkan Wallin. 2011. “Mutation Spectrum in FE1-Muta-Mouse Lung Epithelial Cells Exposed to Nanoparticulate Carbon Black.” Environmental and Molecular Mutagenesis 52 (4): 331–337. doi:10.1002/em.20629.
- Jacobsen, Nicklas Raun, Peter Møller, Keld Alstrup Jensen, Ulla Vogel, Ole Ladefoged, Steffen Loft, and Hålan Wallin. 2009. “Lung Inflammation and Genotoxicity following Pulmonary Exposure to Nanoparticles in ApoE-/-Mice.” Particle and Fibre Toxicology 6 (1): 2. doi:10.1186/1743-8977-6-2.
- Karlsson, Hanna L., Muhammet S. Toprak, and Bengt Fadeel. 2015. “Chapter 4 – Toxicity of Metal and Metal Oxide Nanoparticles.” In Handbook on the Toxicology of Metals. 4th ed. edited by Gunnar F. Nordberg, Bruce A. Fowler, and Monica Nordberg, 75–112. Cambridge: Academic Press.
- Knudsen, Kristina B., Trine Berthing, Petra Jackson, Sarah S. Poulsen, Alicja Mortensen, Nicklas R. Jacobsen, Vidar Skaug, et al. 2019. “Physicochemical Predictors of Multi-Walled Carbon Nanotube–Induced Pulmonary Histopathology and Toxicity One Year after Pulmonary Deposition of 11 Different Multi-Walled Carbon Nanotubes in Mice.” Basic & Clinical Pharmacology & Toxicology 124 (2): 211–227. doi:10.1111/bcpt.13119.
- Kyjovska, Zdenka O., Nicklas R. Jacobsen, Anne T. Saber, Stefan Bengtson, Petra Jackson, Håkan Wallin, and Ulla Vogel. 2015. “DNA Damage Following Pulmonary Exposure by Instillation to Low Doses of Carbon Black (Printex 90) Nanoparticles in Mice.” Environmental and Molecular Mutagenesis 56 (1): 41–49. doi:10.1002/em.21888.
- Loret, Thomas, Emmanuel Peyret, Marielle Dubreuil, Olivier Aguerre-Chariol, Christophe Bressot, Olivier le Bihan, Tanguy Amodeo, et al. 2016. “Air-Liquid Interface Exposure to Aerosols of Poorly Soluble Nanomaterials Induces Different Biological Activation Levels Compared to Exposure to Suspensions.” Particle and Fibre Toxicology 13 (1): 58. doi:10.1186/s12989-016-0171-3.
- Loret, Thomas, Françoise Rogerieux, Bénédicte Trouiller, Anne Braun, Christophe Egles, and Ghislaine Lacroix. 2018. “Predicting the in Vivo Pulmonary Toxicity Induced by Acute Exposure to Poorly Soluble Nanomaterials by Using Advanced in Vitro Methods.” Particle and Fibre Toxicology 15 (1): 1–20. doi:10.1186/s12989-018-0260-6.
- Maeß, Marten B., Berith Wittig, Andrea Cignarella, and Stefan Lorkowski. 2014. “Reduced PMA Enhances the Responsiveness of Transfected THP-1 Macrophages to Polarizing Stimuli.” Journal of Immunological Methods 402 (1–2): 76–81. doi:10.1016/j.jim.2013.11.006.
- Migliore, L., D. Saracino, A. Bonelli, R. Colognato, M. R. D'Errico, A. Magrini, A. Bergamaschi, and E. Bergamaschi. 2010 May. “Carbon Nanotubes Induce Oxidative DNA Damage in RAW 264.7 Cells.” Environmental and Molecular Mutagenesis 51 (4): NA–303. doi:10.1002/em.20545.
- Mikkelsen, Lone, Majid Sheykhzade, Keld A. Jensen, Anne T. Saber, Nicklas R. Jacobsen, Ulla Vogel, Håkan Wallin, Steffen Loft, and Peter Møller. 2011. “Modest Effect on Plaque Progression and Vasodilatory Function in Atherosclerosis-Prone Mice Exposed to Nanosized TiO2.” Particle and Fibre Toxicology 8: 32. doi:10.1186/1743-8977-8-32.
- Modrzynska, Justyna, Trine Berthing, Gitte Ravn-Haren, Nicklas Raun Jacobsen, Ingrid Konow Weydahl, Katrin Loeschner, Alicja Mortensen, Anne Thoustrup Saber, and Ulla Vogel. 2018. “Primary Genotoxicity in the Liver following Pulmonary Exposure to Carbon Black Nanoparticles in Mice.” Particle and Fibre Toxicology 15 (1): 2–12. doi:10.1186/s12989-017-0238-9.
- Møller, P., N. R. Jacobsen, J. K. Folkmann, P. H. Danielsen, L. Mikkelsen, J. G. Hemmingsen, L. K. Vesterdal, L. Forchhammer, H. Wallin, and S. Loft. 2010. “Role of Oxidative Damage in Toxicity of Particulates.” Free Radical Research 44 (1): 1–46. doi:10.3109/10715760903300691.
- Morfeld, Peter, and, Robert J. McCunney. 2007. “Carbon Black and Lung Cancer: Testing a New Exposure Metric in a German Cohort.” American Journal of Industrial Medicine 50 (8): 565–567. doi:10.1002/ajim.20491.
- Morfeld, Peter, Kenneth Mundt, Linda Dell, Tom Sorahan, and Robert McCunney. 2016. “Meta-Analysis of Cardiac Mortality in Three Cohorts of Carbon Black Production Workers.” International Journal of Environmental Research and Public Health 13 (3): 302. doi:10.3390/ijerph13030302.
- Mroz, R. M., R. P. Schins, H. Li, E. M. Drost, W. Macnee, and K. Donaldson. 2007. “Nanoparticle Carbon Black Driven DNA Damage Induces Growth Arrest and AP-1 and NFkappaB DNA Binding in Lung Epithelial A549 Cell Line.” Journal of Physiolology and Pharmacology 58 Suppl 5(Pt 2): 461–470.
- Mroz, R. M., R. P. Schins, H. Li, L. A. Jimenez, E. M. Drost, A. Holownia, W. MacNee, and K. Donaldson. 2008. “Nanoparticle-Driven DNA Damage Mimics Irradiation-Related Carcinogenesis Pathways.” European Respiratory Journal 31 (2): 241–251. doi:10.1183/09031936.00006707.
- Murphy, Fiona A., Anja Schinwald, Craig A. Poland, and Ken Donaldson. 2012. “The Mechanism of Pleural Inflammation by Long Carbon Nanotubes: Interaction of Long Fibres with Macrophages Stimulates Them to Amplify Pro-inflammatory Responses in Mesothelial Cells.” Particle and Fibre Toxicology 9 (1): 8. doi:10.1186/1743-8977-9-8.
- Nymark, Penny, Hanna L. Karlsson, Sabina Halappanavar, and Ulla Vogel. 2021. “Adverse Outcome Pathway Development for Assessment of Lung Carcinogenicity by Nanoparticles.” Frontiers in Toxicology 3: 653311–653386. doi:10.3389/ftox.2021.653386.
- Pal, Anoop K., Dhimiter Bello, Joel Cohen, and Philip Demokritou. 2015. “Implications of in Vitro Dosimetry on Toxicological Ranking of Low Aspect Ratio Engineered Nanomaterials.” Nanotoxicology 9 (7): 871–885. doi:10.3109/17435390.2014.986670.
- Peebles, Brian C., Prabir K. Dutta, W. James Waldman, Frederick A. Villamena, Kevin Nash, Michael Severance, and Amber Nagy. 2011. “Physicochemical and Toxicological Properties of Commercial Carbon Blacks Modified by Reaction with Ozone.” Environmental Science & Technology 45 (24): 10668–10675. doi:10.1021/ES202984T.
- Poulsen, Sarah S., Kristina B. Knudsen, Petra Jackson, Ingrid E. K. Weydahl, Anne T. Saber, Håkan Wallin, and Ulla Vogel. 2017. “Multi-Walled Carbon Nanotubephysicochemical Properties Predict the Systemic Acute Phase Response following Pulmonary Exposure in Mice.” PLoS One 12 (4): e0174167. doi:10.1371/journal.pone.0174167.
- Poulsen, Sarah S., Petra Jackson, Kirsten Kling, Kristina B. Knudsen, Vidar Skaug, Zdenka O. Kyjovska, Birthe L. Thomsen, et al. 2016. “Multi-Walled Carbon Nanotube Physicochemical Properties Predict Pulmonary Inflammation and Genotoxicity.” Nanotoxicology 10 (9): 1263–1275. doi:10.1080/17435390.2016.1202351.
- Ramanakumar, Agnihotram V., Marie-Elise Parent, Benoit Latreille, and Jack Siemiatycki. 2008. “Risk of Lung Cancer Following Exposure to Carbon Black, Titanium Dioxide and Talc: Results from Two Case-Control Studies in Montreal.” International Journal of Cancer 122: 183–189. doi:10.1002/ijc23021.
- Saber, A. T., J. Bornholdt, M. Dybdahl, A. K. Sharma, S. Loft, U. Vogel, and H. Wallin. 2005. “Tumor Necrosis Factor is Not Required for Particle-Induced Genotoxicity and Pulmonary Inflammation.” Archives of Toxicology 79 (3): 177–182. doi:10.1007/s00204-004-0613-9.
- Saber, Anne T., Nicklas R. Jacobsen, Alicia Mortensen, Józef Szarek, Petra Jackson, Anne M. Madsen, Keld A. Jensen, et al. 2012. “Nanotitanium Dioxide Toxicity in Mouse Lung is Reduced in Sanding Dust from Paint.” Particle and Fibre Toxicology 9 (1): 4. doi:10.1186/1743-8977-9-4.
- Saber, Anne Thoustrup, Alicja Mortensen, Józef Szarek, Ismo Kalevi Koponen, Marcus Levin, Nicklas Raun Jacobsen, Maria Elena Pozzebon, et al. 2015. “Epoxy Composite Dusts with and without Carbon Nanotubes Cause Similar Pulmonary Responses, but Differences in Liver Histology in Mice following Pulmonary Deposition.” Particle and Fibre Toxicology 13 (1): 37. doi:10.1186/s12989-016-0148-2.
- Saber, Anne Thoustrup, Keld Alstrup Jensen, Nicklas Raun Jacobsen, Renie Birkedal, Lone Mikkelsen, Peter Moller, Steffen Loft, Håkan Wallin, and Ulla Vogel. 2012. “Inflammatory and Genotoxic Effects of Nanoparticles Designed for Inclusion in Paints and Lacquers.” Nanotoxicology 6 (5): 453–471. doi:10.3109/17435390.2011.587900.
- Sahu, Devashri, G. M. Kannan, and R. Vijayaraghavan. 2014. “Carbon Black Particle Exhibits Size Dependent Toxicity in Human Monocytes.” International Journal of Inflammation 2014: 1–10. doi:10.1155/2014/827019.
- Schmid, Otmar, and Tobias Stoeger. 2016. “Surface Area is the Biologically Most Effective Dose Metric for Acute Nanoparticle Toxicity in the Lung.” Journal of Aerosol Science 99: 133–143. doi:10.1016/j.jaerosci.2015.12.006.
- Sorahan, Tom, and J. Malcolm Harrington. 2007. “A “lugged” Analysis of Lung Cancer Risks in UK Carbon Black Production Workers, 1951–2004.” American Journal of Industrial Medicine 50 (8): 555–564. doi:10.1002/ajim.20481.
- Stoeger, Tobias, Claudia Reinhard, Shinji Takenaka, Andreas Schroeppel, Erwin Karg, Baerbel Ritter, Joachim Heyder, and Holger Schulz. 2006. “Instillation of Six Different Ultrafine Carbon Particles Indicates a Surface Area Threshold Dose for Acute Lung.” Environmental Health Perspectives 114 (3): 328–333. doi:10.1289/ehp.8266.
- Straif, Kurt, Ulrich Keil, Dirk Taeger, Dagmar Holthenrich, Yi Sun, Martina Bungers, and Stephan K. Weiland. 2000. “Exposure to Nitrosamines, Carbon Black, Asbestos, and Talc and Mortality from Stomach, Lung, and Laryngeal Cancer in a Cohort of Rubber Workers.” American Journal of Epidemiology 152: 297–306. https://academic.oup.com/aje/article/152/4/297/67638.
- Tran, C. L., D. Buchanan, R. T. Cullen, A. Searl, A. D. Jones, and K. Donaldson. 2000. “Inhalation of Poorly Soluble Particles. II. Influence of Particle Surface Area on Inflammation and Clearance.” Inhalation Toxicology 12: 1113–1126. doi:10.1080/08958370050166796.
- Valero-Mora, P. M. 2010. ggplot2: Elegant Graphics for Data Analysis. New York: Springer-Verlag.
- Vesterdal, L. K., P. H. Danielsen, J. K. Folkmann, L. F. Jespersen, K. Aguilar-Pelaez, M. Roursgaard, S. Loft, and P. Møller. 2014. “Accumulation of Lipids and Oxidatively Damaged DNA in Hepatocytes Exposed to Particles.” Toxicology and Applied Pharmacology 274 (2): 350–360. doi:10.1016/j.taap.2013.10.001.
- Wallin, Håkan, Zdenka O. Kyjovska, Sarah S. Poulsen, Nicklas R. Jacobsen, Anne T. Saber, Stefan Bengtson, Petra Jackson, and Ulla Vogel. 2017. “Surface Modification Does Not Influence the Genotoxic and Inflammatory Effects of TiO2 Nanoparticles after Pulmonary Exposure by Instillation in Mice.” Mutagenesis 32 (1): 47–57. doi:10.1093/mutage/gew046.
- Wang, Xiang, Tian Xia, Susana Addo Ntim, Zhaoxia Ji, Sijie Lin, Huan Meng, Choong-Heui Chung, et al. 2011. “Dispersal State of Multiwalled Carbon Nanotubes Elicits Profibrogenic Cellular Responses That Correlate with Fibrogenesis Biomarkers and Fibrosis in the Murine Lung.” ACS Nano 5 (12): 9772–9787. doi:10.1021/nn2033055.
- Wils, R. S., N. R. Jacobsen, E. Di Ianni, M. Roursgaard, and P. Møller. 2021. “Reactive Oxygen Species Production, Genotoxicity and Telomere Length in FE1-Muta™Mouse Lung Epithelial Cells Exposed to Carbon Nanotubes.” Nanotoxicology 15 (5): 661–672. doi:10.1080/17435390.2021.1910359.
- Zhou, Lixiao, Peiyuan Li, Mengyue Zhang, Bin Han, Chen Chu, Xuan Su, Binghua Li, et al. 2020. “Carbon Black Nanoparticles Induce Pulmonary Fibrosis through NLRP3 Inflammasome Pathway Modulated by MiR-96 Targeted FOXO3a.” Chemosphere 241: 125075. doi:10.1016/j.chemosphere.2019.125075.