Abstract
Metal-based nanomaterials (MNMs) have gained particular interest in nanotechnology industry. They are used in various industrial processes, in biomedical applications or to improve functional properties of several consumer products. The widescale use of MNMs in the global consumer market has resulted in increases in the likelihood of exposure and risks to human beings. Human exposure to MNMs and assessment of their potential health effects through the concomitant application of biomarkers of exposure and effect of the most commonly used MNMs were reviewed in this paper. In particular, interactions of MNMs with biological systems and the nanobiomonitoring as a prevention tool to detect the early damage caused by MNMs as well as related topics like the influence of some physicochemical features of MNMs and availability of analytical approaches for MNMs testing in human samples were summarized in this review. The studies collected and discussed seek to increase the current knowledge on the internal dose exposure and health effects of MNMs, highlighting the advantages in using biomarkers in primary prevention and health surveillance.
1. Human exposure to metal-based nanomaterials (MNMs)
In 2022, the European Commission (EC) updated the previous definition of nanomaterial (NM) included in the 2011/696 EC Recommendation (EC Citation2011) considering the scientific progress and the regulatory experience (EC Citation2022). According to the 2022 EC Recommendation, a ’Nanomaterial’ means a natural, incidental or manufactured material consisting of solid particles that are present, either on their own or as identifiable constituent particles in aggregates or agglomerates, and where for 50% or more of these particles in the number-based size distribution, one or more external dimensions of the particle are in the size range 1 nm–100 nm.
Engineered metal-based nanomaterials (MNMs) are manufactured to have specific chemical and physical properties different than their conventional counterparts (higher chemical reactivity, surface charge, efficient electrical conductivity, higher strength, hardness, etc.) and enable new and enhanced products, devices and applications. Among engineered MNMs, the pure form of metallic NMs as Ag are commonly used for their antibacterial properties or Au in medicine, whilst, for example, Ir, Pd and Pt are highly used as catalysts and in industry. The other types of NMs are metal oxide NMs as Al, Cr, Ni and Zr oxides that have enormous applications in cosmetic, food, textile, plastics, etc. In addition, Ti and Zn oxides have been approved as sunscreens, and they have other applications in medicine and textile, plastic, and food industries. Cerium, Co, Cu, In, and Mn oxides, on the other hand, have applications in the electrical industry, such as catalysts, solar energy, waste treatment, environment remediation, etc.
Researchers are now focusing on modified MNMs for their higher stability, higher antimicrobial activity, excellent drug-loading capacity, good biodegradability, and good biocompatibility. Emerging types of MNMs are doped metal/metal oxide NMs, metal sulfide NMs and metal-organic frameworks (MOFs) (Yaqoob et al. Citation2020). Doped metal/metal oxide NMs (e.g. Ag-TiO2, Au-CuO, Pt-ZnO NMs) generally are characterized by an improved antibacterial efficiency than non-doped ones and are recommended to be used in different applications in pharmaceuticals (Yaqoob et al. Citation2020). In addition, metal sulfide NMs – CuS, Ag2S, FeS NMs – have fluorescence, optical, thermal, magnetic, and structural stability characteristics and are considered the most promising semiconductor resource (Vena, Jobbágy, and Bilmes Citation2016). The MOFs (Zn-based, Cu-based, Mn-based MOF) are nanoporous materials with higher porosity and higher surface area than conventional porous materials which can offer many opportunities for applications in drug delivery, biosensing and bioimaging (Arenas-Vivo et al. Citation2019).
Then, natural MNMs are not created directly through human actions but are formed during natural physicochemical processes such as volcanic activity and forest fires. It was observed that Ni, Zn, Ag, Cd, Tl, Pb, Bi, Te, and Hg were present in volcanic ash at diameters between 70 and 500 nm (Ermolin et al. Citation2021). Chromium, Cu, and As were the dominant elements in the ashes of combustion of vegetation and burn structural materials as hetero-aggregates of single-metal and multi-metal NMs (Alam et al. Citation2023).
Incidental MNMs occurred during human processes such as welding gases, vehicle exhaust gases, solid fuel heating (home heathers), combustion during cooking, and the 3D printing process. The inhalable particulate matter analysis showed that welders were exposed to <100 nm particles constituted by Cr2O3 > Mn3O4 > NiO (Bocca et al. Citation2023). It was also reported the presence of ca. 5–100 nm large Fe3O4 particles, in form of agglomerates, in diesel engines exhaust (Liati et al. Citation2015). In addition, personal exposure to quantifiable levels of metals as Al, Cr, Cu, Fe and Ti in particles <300 nm during 3D printing tasks was reported (Du Preez et al. Citation2018).
The application of even more innovative nanomaterials has the consequence of increasing the likelihood of environmental and human exposure to low-dose concentrations of MNMs (Iavicoli et al. Citation2018). Even if MNMs continue to bring promising advances in science and technology, on the other hand their potential effects on the health of workers and the community at large, as well as the environment, are largely unknown. MNMs represent a land of contrast which leaves open questions: Are they an economic opportunity and a scientific advancement or a health risk? Are they useful or unwanted?
The outcome of various nanotoxicology studies indicates that MNMs can produce multiple adverse effects in biological systems mainly due to the production of reactive oxygen species (ROS) that results in oxidative stress; moreover, MNMs can also produce toxic effects on cells by ROS-independent mechanisms such as those associated with morphology, size, and zeta potential of MNMs (Augustine et al. Citation2020). Also in the case of modified MNMs, there is still the necessity to shed more light on their toxicity and biocompatibility in the context of their applications, in order to fully exploit the potential of this versatile class of materials in the future. For example, the toxicity of MNMs could be increased by the introduction of a metal-doping. Surface doping with CuO increased pulmonary toxicity of SiO2 nanomaterials, and Cu-doped TiO2 NMs induced cytotoxicity and oxidative stress in a dose-dependent manner (Ahmad et al. Citation2018; Hadrup et al. Citation2021). The potential toxicity of MOFs is affected by their chemistry, particle size, morphology, and particle aggregation (Menon and Chakraborty Citation2023). The available in vitro and in vivo studies revealed they can cause cellular damage, oxidative stress, inflammation, and genotoxicity in vitro, while in vivo studies have shown that they can cause organ damage and immune system dysregulation (Wiśniewska et al. Citation2023).
There are already some papers in which the necessities for regulations and exposure limits of NMs in environmental settings are discussed and the difficulties also addressed (Rodríguez-Ibarra et al. Citation2020). In general terms, the metal oxide NMs and carbon based NMs are the most advanced in terms of limits of exposure according to different regulatory entities (Rodríguez-Ibarra et al. Citation2020). For example, an occupational exposure limit <0.3 mg/m3 for the inhalable TiO2 fraction has been established by the NIOSH (National Institute for Occupational Safety and Health) (NIOSH Citation2011). In other cases, some regulatory entities consider the bulk material as a reference for establishing limits of exposure to NMs, without considering their nanospecific physicochemical characteristics.
2. Exposure assessment
Exposure assessment is an important part of the health risk estimation process and the tool for measuring the extent, frequency, and duration of exposure to a substance in the general population, workers or in a specific group of individuals. It allows to answer the questions: What populations are exposed? What are the exposure levels? What are the frequency and duration of exposure? Depending on the conditions of manufacture, formulation, use, and final disposal, the exposure assessment of MNMs may need to address: (i) worker exposure during the manufacture of MNMs; (ii) the exposure of consumers using products that contain MNMs; (iii) exposure of local populations due to chronic or acute release of MNMs from manufacturing and/or processing facilities; (iv) the potential for human exposure through the environment.
According to the opinion of the SCENIHR (Scientific Committee on Emerging and Newly Identified Health Risks) (SCENIHR Citation2006), the exposure assessment to NMs should include: (i) the identification and quantification of relevant exposure; (ii) the determination of the absorption of NMs by the appropriate route(s) of exposure at relevant doses; (iii) the characterization and quantification of NMs in body tissues; (iv) the potential for bioaccumulation following repeated exposure to NMs. However, this is a complex process due to the large number and type of available NMs, possible changes in biological properties during the life cycle of the NM, lack of standard operating procedures (SOPs) to assess the exposure and risk, selection of the appropriate and harmonized measurement methods and metrics (OECD Citation2022).
The presence of a hazard does not in itself constitute a real risk to health, as this derives from contact with the body during work or use. Therefore, the assessment of exposure has a basic position in the risk assessment, allowing to estimate the actual contact between the hazard and the body (Abbott and Maynard Citation2010). Given the presence of MNMs in various media (air, water, food, consumer products), there are many possibilities for human exposure (). It may results from different sources (environment, workplace, consumer products) and by different routes that are inhalation through the respiratory tract, exudation through the skin, and ingestion through the digestive tract (Kuhlbusch, Wijnhoven, and Haase Citation2018; Zoroddu et al. Citation2014). Currently, for exposure to MNMs, the inhalation route is considered the main concern both in the workplace (Bocca, Leso, et al. Citation2023; Pietroiusti and Magrini Citation2014) and for consumers of different products such as spray or paints (Sharma, Kumar, and Dhawan Citation2012). Food or food contact materials are the most relevant source of oral exposure that includes also the accidental ingestion of residues (e.g. cosmetics) or migration from an article to a food (EFSA Citation2011, Citation2016). Skin exposure can occur from contact with the skin of a consumer product containing or releasing MNMs. A major products category for dermal exposure to MNMs of TiO2 and ZnO are cosmetics and sunscreens (Bocca et al. Citation2018; EC Citation2009). The Environmental Protection Agency (EPA) conclusion on dermal absorption of MNMs, as Ag, Au, Co, CuO, Fe2O3, SiO2, TiO2, and ZnO, is that absorption of particles in the nano-range through the skin is possible but it occurs to a very low degree (Poland et al. Citation2013). In addition, intradermal injection of tattoo inks may represent an additional route of exposure to Al, Cr, Cu, Pb, Ti, and Zn micro- and nanoparticles (Battistini et al. Citation2020). Moreover, a number of MNMs incorporated into toner formulations (e.g. SiO2, Al2O3, TiO2, Fe2O3, ZnO, CuO, CeO2, and carbon black among others) are released into the air during printing (Pirela et al. Citation2015). Unintentional release of Ag, Al2O3, SiO2, TiO2 and ZnO nanoparticles also includes accidental spills or industrial liquid effluents such as those emitted by textile industries during the washing of nanotextile (Saleem and Zaidi Citation2020). In recent years, particular attention is given to products that are deliberately used in NM form in the environment, e.g. nanopesticides, biocides, environment improving agents (Bocca et al. Citation2020). Another aspect is the human exposure involved in the disposal or recycling of NM dependant products. This includes the potential for MNMs to gradually escape from waste disposal sites including industrial waste, household waste, and hospital waste well as their impact on sewage treatment plants (Suman and Pei Citation2022).
The research on exposure to MNMs started firstly at workplaces for the reasons that (a) the kind of NM to identify and quantify is clearly defined, (b) the concentrations are the highest to be expected and hence likely of the highest relevance, and (c) well defined conditions are available facilitating the use of experimental measurement set-ups (Iavicoli, Leso, and Schulte Citation2016). Company workers that produce and use NMs or involved in processes that generate NMs as by-products are typically exposed to higher levels of MNMs and for more prolonged periods of time compared to the general population. Workplace screening and surveillance for workers exposed to MNMs could potentially help early detection of health effects, serve as a source of data to evaluate epidemiological links between exposure and health outcomes and inform actions to prevent diseases due to exposure to MNMs (Gulumian et al. Citation2016). According to a 2011 forecast, 6 million workers worldwide would be exposed to NMs by the end of 2020 (Roco Citation2011). Consumers can be potentially exposed to MNMs in products during different phases of the product lifecycle: production, processing, use phase, end-of-life cycle. Exposure assessment of consumers to MNMs is even much complex because all releases and transformation processes should be considered and important information on the use of MNMs in consumer products is still lacking (Kuhlbusch, Wijnhoven, and Haase Citation2018). Exposure via the environment is still the least developed area (Colvin Citation2003). The main problem is the identification of MNMs in the environment. In matrices like natural waters, particle agglomerates in ambient air, or soil it is unclear how a specific MNM can clearly be identified and quantified due to the complexity of the matrix but also partially due to a high natural particle background (Vladitsi et al. Citation2022).
The exposure assessment can be done by environmental monitoring, measuring the concentration of chemicals in potential sources of exposure (e.g. air, food, water), or by human biomonitoring which involves measurements of internal dose of chemicals or their effects in human matrices (as blood and urine) in subjects exposed or who have been exposed in the past (CDC Citation2005).
In this study, the recent progress in biomarkers of exposure and effect in human biomonitoring of MNMs were summarized, since manufactured, natural or incidental MNMs are produced, transformed, and exported into our environment daily, and human exposure to MNM is a major concern for public health. This topic is discussed in the following paragraphs, in terms of MNMs properties and interaction with biological systems, presence of MNMs in the body, biomarkers of exposure and effect in the risk assessment of MNMs, activities of human biomonitoring of MNMs, and analytical characterization of MNMs in human samples.
3. Interaction of MNMs with biological systems
The MNMs once enter in the body can remain intact, be subjected to dissolution, speciation, transformation, agglomeration, interact with cells, proteins, and tissues. Concerns arose when MNMs showed the following specific biological properties: (i) translocation to secondary target organs (liver, spleen, lymph nodes, brain); (ii) the poor clearance by macrophages; (iii) the ability to reach intracellular structures such as mitochondria and the nucleus; (iv) the ability to transmit signals and sensory information to or from the central nervous system (Pietroiusti and Magrini Citation2014).
As reported in , the properties of NMs, such as particle size, shape, surface charge (usually stated as zeta-potential), aggregation/agglomeration and dissolution, and other characteristics must be taken into consideration when talking about interaction of MNMs with biological systems and nanotoxicology. The OECD developed a number of test guidelines (TGs) addressing physico-chemical endpoints of NMs, i.e. on particle size and size distribution, dissolution rate in biological media, surface chemistry, surface area, etc., and Rasmussen et al. (Citation2019) presents an overview of OECD TGs that are applicable to testing NMs. These properties indeed have a great influence on the bioavailability and toxicity of the NM since they can modify the absorption, accumulation, translocation, and toxicological response; a specific property may give rise to either a safe particle or to a dangerous one (Zoroddu et al. Citation2014).
Table 1. Characteristics of metal-based nanomaterials (MNMs) affecting exposure and health effects.
In particular, it is biologically plausible that as the particle size reduces, a sudden increase in absorption and/or toxicity arises. Nanosized materials with a primary particle or agglomerate size between 10 and 100 nm were deposited more efficiently in the alveolar region than particles with a larger size (Braakhuis et al. Citation2014). Among the MNMs diffused in the alveoli the absorbed portion increased from 30 to 40% for particles of diameter ca. 200 nm to 80% for particles smaller than 30 nm (ICRP Citation1994; Kaminski et al. Citation2015). The highest absorption across intestinal barriers and accumulation in secondary target organs was mostly found for very small Au particles (1.4 nm) (Schleh et al. Citation2012), and 18 nm Au particles showed accumulation in the brain and heart. In healthy subjects, MNMs with sizes <40 nm in diameter could penetrate the stratum corneum through the lipid intercellular route or aqueous pores, while the largest ones did not cross the skin layer (Gautam, Singh, and Vijayaraghavan Citation2011).
Williams et al. (Citation2015) investigated the influence of size NMs on organ targeting: microparticles (>1000 nm) exhibited nonspecific deposition in the lungs; the mesoscale particles of 350 to 400 nm were accumulated in the kidney, while particles between 10 and 250 nm were phagocytosed by the reticuloendothelial system and bioaccumulated in liver and spleen. Similar results were found for Ag nanoparticles of 20 and 200 nm that translocated from blood to main organs, mainly liver and kidney (Dziendzikowska et al. Citation2012). Choi et al. (Citation2010) demonstrated the more rapid translocation from lung to lymph nodes (<3 min) and to blood (<10 min) for the smaller (5 nm) inorganic/organic hybrid nanoparticles, with measurable particle accumulation in kidney and excretion in urine at 30 min post-administration. A suspension of SiO2 particles (150 nm) was introduced into the circulatory system of rats, and results showed the presence of dissolved SiO2 in urine at 4 d after administration, and accumulation of SiO2 in the heart, lungs, kidneys, and liver (Borak et al. Citation2012).
The smaller MNMs can be more bioavailable resulting in a higher likelihood of interaction with cells and a more prominent toxicity (Dong et al. Citation2020). For example, a size-dependent genotoxic effects of Ag, Au, and Pt nanoparticles at 5 and 50 nm were reported (Lebedová et al. Citation2018). Also Au nanoparticles at a size of 1.4 nm showed a direct interaction with DNA with important associated toxicity (Tsoli et al. Citation2005). Studies also indicated that the protein corona formed when NMs interact with serum and extracellular matrix proteins could significantly change the size (and also the surface charge) and influence cellular uptake. For instance incubation of magnetite (Fe3O4) cores of 25–30 nm in a cell culture medium for a few minutes generated protein adsorption and a 5-fold increase of the hydrodynamic diameter (Calatayud et al. Citation2014).
Moreover, different shapes showed different cellular uptake mechanisms (Graf et al. Citation2018). Tak et al. (Citation2015) observed that different forms of Ag NPs (rod-shaped, spherical, and triangular) had different skin penetration rates and penetration depths. In addition, the efficiency of cellular uptake of Au nanoparticles was found to be easier for stars, followed by rods and triangles (Xie et al. Citation2017). The shape also affected levels of toxicity. In the paper of Steckiewicz et al. (Citation2019) the cytotoxicity of Au nanoparticles was shape-dependent with stars having the most cytotoxic potential of tested nanoparticles, whereas spheres appeared the safest ones. In other papers, rod-shaped Fe2O3, TiO2, ZnO, and CeO2 nanoparticles were found to produce much higher cytotoxic responses than spherical Fe2O3, TiO2, and ZnO nanoparticles (Breza and Šimon Citation2020; Hsiao and Huang Citation2011; Lee et al. Citation2014) and octahedron or cubic CeO2 nanoparticles (Forest et al. Citation2017). Exposure to nanorods of Al2O3 affected more metabolic pathways in astrocytes than did exposure to nanoflakes. The most perturbed metabolic pathways affected by nanorods were those closely linked to oxidative stress, inflammation, and neurodegeneration, including the metabolism of linoleic acid and glycerophospholipids (Dong et al. Citation2019).
Regarding surface charge, greater absorption has been demonstrated for negatively charged Au particles compared to positively charged Au particles (Lee et al., Citation2013; Schleh et al. Citation2012). Nevertheless, the charged Au nanoparticles displayed higher toxicity than neutral mediating toxicity (cell morphology, mitochondrial stress, and gene expression) (Schaeublin et al. Citation2011). Similarly, positively charged Si nanoparticles proved to be more cytotoxic in terms of reducing mitochondrial metabolic activity and effects on phagocytosis than neutral Si nanoparticles, while negatively charged Si nanoparticles showed very little or no cytotoxicity (Bhattacharjee et al. Citation2010). Lung-administered cationic nanoparticles were rapidly taken up by pulmonary macrophage and/or epithelial cells shortly after administration, and remained in pulmonary cells for a long time causing severe lung injury (Choi et al. Citation2010).
In addition, the extent to which MNMs may produce soluble available metal ions in the media or the state of agglomeration or aggregation may trigger different toxicity end-points and should therefore be considered (ECHA Citation2016). Okuda-Shimazaki et al. (Citation2010) demonstrated that larger aggregates of TiO2 showed a greater effect on cell viability and gene expression than smaller aggregates. To the opposite, the rapid dissolution rate of some MNMs, such as ZnO, CuO or CoO, and the release of metal ions was associated to acute toxicity on site, provoking for example oxidative stress in the lung or skin sensitization (Fukui et al. Citation2012; Kim et al. Citation2021).
Other properties as the surface area, dose, surface coating, functionalization, and porosity are recognized influencing the bioavailability in the media and thus the nanotoxicology of MNMs. Fubini, Ghiazza, and Fenoglio (Citation2010) noted that NMs may show effects orders of magnitude greater than that of bulk particles at the same mass dose due to vastly greater surface area. This reflects the different spectrum of toxicity depending on differences in surface area. Regarding the dose, a high dose-dependent association was observed between exposure to nanoparticles of TiO2 and biomarkers of lipid oxidative and cardiopulmonary damage (Pelclova et al. Citation2017; Zhao et al. Citation2018). Functionalization can inhibit protein corona formation when it comes into contact with serum proteins. Owing to the ability to resist protein adoption, NMs can be decorated with polyethylene glycol (PEG) to minimize nonspecific membrane interactions. Papers reported that the PEG coating of MMNs (Cu and Fe2O3) reduced cellular damage and lessened ROS production (Shukla et al. Citation2015; Worthington et al. Citation2013; Yu et al. Citation2012a). Moreover, membrane penetration and subsequent target binding were much higher for oligonucleotide functionalized Au nanoparticles compared to unmodified ones (Dhar et al. Citation2009). Finally, the toxicity of SiO2 was found to be influenced by nanoparticle porosity, geometry, and surface characteristics (Yu et al. Citation2012b).
4. The presence of MNMs in the body
Some studies have shown the presence of MNMs in the body as reported in . Four postmortem studies found the existence of a background level of SiO2, TiO2, NiO nanoparticles in the lung, liver, spleen, kidney and intestine tissues of the general population (Heringa et al. Citation2018; Peters et al. Citation2020; Phillips et al. Citation2010). Peters et al. (Citation2020) observed Si and Ti particles in various postmortem tissues; the detected TiO2 particles had a size <100 nm, whilst Si particle sizes ranged from 150 to 850 nm (Peters et al. Citation2020). Heringa et al. (Citation2018) found TiO2 particles in the human liver and spleen, with ≥24% of particles <100 nm. The levels were below the doses regarded as safe in animals, but half data were above the dose that is deemed safe for liver damage in humans (Heringa et al. Citation2018). Aggregates of particles ranging between 50 nm and 100 μm and composed mainly of Si and Fe, and to a lesser extent of Al, Ni, Pb, Ti and Hg, were observed in 94% of livers and in 97% of kidney autopsy samples of 35 subjects living in Sardinia (Italy) (Locci et al. Citation2019). Another three studies on volunteers demonstrated the possibility of transcutaneous absorption of TiO2 and ZnO NMs after the application of sunscreens (Gulson et al. Citation2010; Naess et al. Citation2016; Pelclova et al. Citation2019). Naess et al. (Citation2016) observed that during realistic consumer use of sunscreens - on both intact and UVB sunburned skin - TiO2 nanoparticles can penetrate the skin beyond the stratum corneum into viable cells in the epidermis. This result was confirmed by Pelclova et al. (Citation2019) and Gulson et al. (Citation2010) that measured Ti and Zn level in blood, plasma and urine in all sunscreen users suggesting the systemic absorption of these metals. Another study found a portion of TiO2 particles in blood after oral administrations to healthy volunteers (Pele et al. Citation2015). The results showed a bimodal pattern of TiO2 particle uptake occurring in human blood with an early absorption after 2 h and a maximum at 6 h post ingestion.
Table 2. Presence of metal-based nanomaterials (MNMs) in human fluids, tissues and organs.
Detection of nanoparticles in humans after exposure to MNMs at work is the subject of a few case reports. A study demonstrated the inhalation absorption of SiO2 particle after exposure of workers to polyacrylate spray paint and damage to vascular endothelial cells, alveolar epithelial cells, and macrophages was observed (Song et al. Citation2011). Nanoparticles of TiO2 were detected in the pulmonary cells of a 58-year-old man who developed bronchiolitis obliterans organizing pneumonia after working with polyester powder paint containing TiO2 nanoparticles (Cheng et al. Citation2012). At autopsy, Ni nanoparticles were detected in a huge amount of a worker during the spraying activity within the lungs where they can be able to induce lung damages (Phillips et al. Citation2010).
5. Human biomonitoring of MNMs as a prevention tool
The strengths of human biomonitoring are that it measures the actual exposure and absorption – what has actually entered the body from all sources or pathways – rather than the potential exposure, and it takes into account variables that it would be difficult to consider, such as the different routes of exposure active at the same time (e.g. air plus water plus food) (Frazzoli et al. Citation2022; Frazzoli, Bocca, and Mantovani Citation2015). In addition, the individual level of exposure can only be quantified and assessed with the use of human biomonitoring.
Human biomonitoring is on a continuum that includes the emission of a contaminant into the environment, exposure and absorption by humans (called biomonitoring of exposure) until a health effect takes place (Jones Citation2020) (). As exposure and absorption continue, early and reversible effects begin to be observed in cells and tissues, and the measurement of these changes is referred to as biomonitoring of effect. Therefore, human biomonitoring in public health helps to evaluate possible interventions before any health effects occur, and primary prevention is the guiding principle of human biomonitoring (WHO Citation2015).
In the area of occupational medicine or hygiene, the term human biomonitoring is to be understood as the measurement of chemicals in biological materials of workers as an integral part of the overall occupational health and safety strategy (Schaller, Angerer, and Drexler Citation2002). It can help assess actual worker risk, where air monitoring alone may seriously underestimate the total uptake of certain chemicals. The aim is to assess the exposure and health risk posed to workers, to compare the analysis values obtained with appropriate biological reference values and to propose possible suitable measures (improvement and prevention) for reducing the risks posed to health.
In particular, nanobiomonitoring, i.e. human biomonitoring of NMs - is essential (Bergamaschi et al. Citation2015; Schulte et al. Citation2018a): (i) to discover which MNMs are absorbed by humans and at what concentration; (ii) to establish reference ranges for use by physicians and scientists to identify people and groups with unusually higher exposures to MNMs; (iii) to assess effectiveness of public health efforts to reduce exposure to certain MNMs in the environmental or occupational settings; (iv) to determine if exposure levels are higher in certain groups (children women of childbearing age, senior citizen) or to discover geographic locations where the internal dose of MNMs is higher from the general population; (v) to track trends in levels of population exposure to MNMs over time; (vi) to give powerful tool for epidemiologic studies (provides unequivocal evidence of exposure and reduces need for calculated approximations); (vii) to set priorities for research on human health effects for certain MNMs.
Currently, human biomonitoring of NMs is not considered by authoritative or regulatory agencies in the frame of risk assessment to chemicals, but there is a growing knowledge base to support such biomonitoring (Schulte et al. Citation2018a). According to REACH (Registration, Evaluation, Authorization, and Restriction of Chemicals), human biomonitoring can be used for risk assessment, but REACH requirements do not include a concrete use of human biomonitoring, and therefore, the development of knowledge on the relationship between the internal doses and effects of chemicals is not a common practice. According to Forest and Pourchez (Citation2023), the human biomonitoring of MNMs in subjects appears as a new and alternative approach to time- and resource- consuming epidemiological studies to highlight relationships between exposure to MNMs and diseases development.
The above mentioned advantages of human biomonitoring have also some sensitive aspects (Bergamaschi et al. Citation2015, Citation2019; Gulumian et al. Citation2016; Iavicoli et al. Citation2014): (i) the presence of MNMs in matrices does not imply disease but represents only a snapshot in time of a person’s exposure to MNM; (ii) the relationship between health status and concentration of MNMs is not clear at present; (iii) it is necessary to be familiar with the production process and the uses of MNMs, the exposure control measures in the company such as personal protection equipment (PPE) and the behavior of workers/consumers; (iv) it requires the administration of questionnaires to acquire all the necessary information (e.g. age, gender, tobacco exposure, residential area, working area, housing, diet, hobbies, etc.) to inform on possible exposure sources and to control for possible confounders; (v) erroneous interpretation of human biomonitoring data could lead people to change their behavior in an unfounded way; (viii) complexity in communicating results (what is the message? who is it for?).
6. Biomarkers in risk assessment to MNMs
Human biomonitoring relies on the use of biomarkers as tools to protect the health of the population and workers and biomarkers presents advantages respect to epidemiology, environmental monitoring, in vitro and animal models in risk assessment to MNMs () (Schulte et al. Citation2018a). Indeed the in vitro experiments uses high exposure concentrations which are unrealistic, the physico-chemical characteristics of the MNMs can interfere with in vitro tests resulting in erroneous data interpretation, as well as different cell lines can produce different responses (Krug Citation2014). When using animals, dosimetric adjustments to account for differences in NMs toxicokinetic and toxicodynamic between animals and workers must be used (Schulte et al. Citation2018a). Instead, the use of biomarkers allow to obtain long-term exposure data and explore lower end of dose-response curve, and they are seen as particularly important in assessing mixed/multiple exposures and variable exposures (e.g. magnitude and frequency) (Viegas et al. Citation2020).
Figure 2. Advantages and limitations of various approaches to assess the complex link between dose and adverse effects.
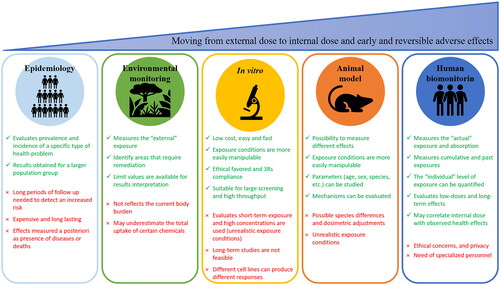
Biomarkers of exposure are an exogenous substance, metabolite or the product of the interaction between the xenobiotic agent and the measurable target molecule or cell within the body. Whilst a biomarker of effect is any biochemical, physiological, or other alteration, measurable within the body that could lead to an effect on health and subsequently to clinical diseases (WHO Citation2015). Since exposure and effect biomarkers often reflect exposure and subclinical changes before the onset of disease, they are a valuable tool for preventing exposure and anticipating the potential adverse effects of NMs.
The characteristics of a valid biomarkers are: (i) for a biomarker of exposure: consistently linked with exposure at relevant levels of exposure with confounding and background exposures assessed; (ii) for a biomarker of effect: consistently linked with increased risk with confounding and modifiable factors assessed. The characteristics of a good biomarker – such as reliability, sensitivity and specificity, but also practicality, accessibility, and the availability of an appropriate and validated analytical technique for detection – are difficult to find in the case of MNMs. In fact, for the development of biomarkers for MNMs the criticalities are actually higher due to the lack of knowledge of metabolism and toxicokinetic, the lack of reference and limit values, the high cost of analytical instrumentation and the need for highly experienced team to quantitatively and/or qualitatively determine whether the MNMs are present in biological media and will pose a risk (OECD Citation2022).
Examples of sources of error in biomarker measurements are the followings: (i) the laboratory method: may not measure all sources of biological exposure; may measure other exposures that are not the true exposure of interest; methods may be influenced by subject characteristics that the researcher can’t manipulate; (ii) the sampling protocol: failure to specify the timing and method of samples collection, handling, storage, and preparation; (iii) the analytical procedure: failure to standardize the instrument, between-batch variation, systematic and random errors (White Citation2011).
6.1. Biomarkers of exposure
describes the literature studies that used biomarkers of exposure in subjects exposed to MNMs. The first article on biomarkers of exposure was published in 2012 on two workers in MNMs production exposed at Ag nanoparticles (Lee et al. Citation2012). Since then, a very limited number of surveys (no. 8) were carried out to assess workers exposure to MNMs. The sampled population is often small, except for the Marie-Desvergne’s study in 2016 where 458 people (exposed and controls) were recruited from airport staff (Marie-Desvergne et al. Citation2016). Most of these studies involved single MNM exposure (e.g. Ag, TiO2, In), one study concerned both Cr and Ni nanoparticles, and four studies involved multiple MNMs exposure. Blood and urine specimens were frequently analyzed, but recently the EBC testing, as single or together with other matrices, has been privileged. Indeed EBC analysis is completely noninvasive and has sufficient sensitivity to assess the inhalation exposure to MNMs. Bocca, Leso, et al. (Citation2023) on a cohort of welders observed Cr2O3 and NiO nanoparticles at high amounts in EBC, whilst a very low number of particles were found in plasma, and no particles were observed in urine. Moreover, two male workers involved in the manufacturing of Ag NMs showed detectable levels of Ag in blood, but not in urine (Lee et al. Citation2012). Similarly, Pelclova et al. (Citation2015) did not detect Ti in the urine of TiO2 nanomaterial production workers.
Table 3. Biomarkers of exposure to metal-based nanomaterials (MNMs).
Nevertheless this, the link between air nanoparticles and particles in biological matrices is not clear. Some studies associated the origin of particles in the EBC of workers to inhaled MNMs during the work shift, whilst other surveys did not find differences in particle counting between the exposed and control group. In the paper of Marie-Desvergne et al. (Citation2016) a significantly higher level of Cd nanoparticles was found in airport workers compared to administrative workers, with the workers being exposed to even smaller nanoparticles than the administrative group. In stainless-steel welders, the EBC showed Cr2O3 particles concentration significantly higher at post-shift at the end of week than at pre-shift at the beginning of week (Bocca, Leso, et al. Citation2023), and also in tungsten inert gas (TIG) welders both EBC and urinary Cr concentrations were higher at the end of week on Friday, respect to following Monday morning (Riccelli et al. Citation2018).
Contrarily, urinary Ag and blood Au and TiO2 nanoparticles were detected at comparable concentration in both workers involved in NM-activities and control subjects (Bocca, Battistini, et al. Citation2023). These results might support the role of extra-professional sources of exposure to MNMs considering that they are used in cosmetics, textiles, health-care products, and in the food industry with their possible release and absorption by human. In other occupational settings, the comparable presence of MNMs in workers and controls might suggest the effectiveness of the preventive and protection measures adopted in the workplace. On the basis of the findings of Methner (Citation2010), worker inhalation exposure to TiO2 nanoparticles may be effectively reduced by a properly designed local exhaust ventilation (LEV) together with health practices at work (Santonen et al. Citation2023).
6.2. Biomarkers of effect
Biomarkers of effect in subjects exposed to MNMs have been scarcely explored as reported in . The first articles (no. 3) concerning the measurement of biomarkers of effect in workers exposed to MNMs were published in 2012, and after then other 15 articles were published. Most of the studies (no. 5) determined effect biomarkers linked to the exposure to TiO2 nanoparticles only, 2 studies concern effects due to exposures to In or SiO2 individually, whilst the others (11 studies) examined effects related to mixtures of different MNMs (such as Ag, Au, TiO2, Cr, Ni, SiO2, Fe2O3). In the field of effect biomarkers analysis, the sample size is usually higher (> 100 individuals in 9 surveys) than in exposure biomarkers studies.
Table 4. Biomarkers of effect to metal-based nanomaterials (MNMs).
Most of the studies determined biomarkers of oxidative stress and lipid peroxidation in EBC, plasma and urine – hydrogen peroxide (H2O2), malondialdehyde (MDA), 8-isoprostane (8-iso), 4-hydroxy-nonenal (HNE) and aldehydes C6–C12 – and antioxidant enzyme activities – glutathione peroxidase (GPX) and copper–zinc superoxide dismutase (SOD) – to assess the relationships between exposure to MNMs and any imbalance between the generation of reactive oxygen species (ROS) and anti-oxidative defenses.
Also some studies examined the DNA and RNA oxidation biomarkers in EBC, plasma and urine, including 8-hydroxy-2′-deoxyguanosine (8-OHdG) and 5-hydroxymethyl uracil (5-OHMeU) (both are DNA damage biomarkers) and 8-hydroxy-guanosine (8-OHG) as RNA damage biomarker. The results by Liou et al. (Citation2016) and Liao et al. (Citation2014) showed lower SOD and GPX and higher 8-OHdG in the plasma of workers exposed to TiO2, SiO2, and indium tin oxide (ITO) than controls. A significant elevation of MDA, HNE, 8-OHdG in EBC was found in post-shift samples in comparison to pre-shift samples in workers exposed to TiO2 aerosol (Pelclova et al. Citation2012). Significant increases in the concentration of plasma and urine H2O2 levels and urine 8-OHdG levels were found at 3 h post exposure in tungsten inert gas (TIG) welders (Graczyk et al. Citation2015). Riccelli et al. (Citation2018) in TIG welders observed that H2O2 EBC levels were strictly related to shift work, whereas MDA EBC levels were influenced by weekly exposure.
A few studies found a positive correlation between biomarkers of effect and biomarkers of exposure. In multi-walled carbon nanotubes (MWCNTs) workers, the MDA and n-hexanal levels measured in EBC were significantly correlated with the blood Mo concentration, suggesting MDA, n-hexanal and Mo as useful biomarkers of exposure and effect to MWCNTs (Lee et al. Citation2015). In addition, increased levels of all markers of lipid peroxidation (e.g. MDA, HNE, 8-iso and aldehydes C6-C12) were found elevated in workers exposed to nano TiO2 in EBC (but not in urine), and significant dose-dependent association was found between exposure to TiO2 and the measured biomarkers (Pelclova et al. Citation2016a, Citation2016b, Citation2017). Workers exposed to SiO2 and TiO2 nanoparticles in NMs manufacturing and/or handling factories showed induced oxidative damages measured by urinary 8-OHdG and EBC 8-iso levels, and a significant positive correlation was observed between urinary 8-OHdG and EBC 8-iso levels (Liou et al. Citation2017). Interestingly, 8-OHdG appeared to be the best biomarker of chronic exposure to MNMs since it was significantly elevated in a follow-up study performed over three years in a nanomaterials industry; while MDA appeared to be the most sensitive marker for acute exposure as it was significantly elevated in post-shifts than in pre-shifts (Pelclova et al. Citation2020). A recent review reported that several studies found 8-OHdG levels increased in the EBC of NMs exposed workers and estimated a preliminary reference value for 8-OHdG (Hemmendinger et al. Citation2020). But the same study reported that this biomarker has not been validated in large cohort studies and can be influenced by the analytical method or by confounding factors as smoking (Hemmendinger et al. Citation2020).
Oxidation products of proteins may also serve as biomarkers of oxidative damage; for example, o-tyrosine (o-Tyr), 3-chlorotyrosine (3-ClTyr) and 3-nitrotyrosine (3-NOTy), were observed higher in workers exposed to TiO2 dust than in controls, which was in agreement with Ti levels measured in EBC samples (Pelclova et al. Citation2016a).
The release of inflammatory cytokines, such as interleukin 6 (IL-6), interleukin 8 (IL-8), and tumor necrosis factor a (TNF-a) in plasma may represent a good marker of early inflammatory response, and higher levels of cytokines were observed in workers exposed to nano-TiO2 (Zhao et al. Citation2018). Also leukotrienes (LTs), which are lipid mediators of inflammation were found higher (LTs B4, C4, and E4) in EBC samples (whilst not in urine) of workers exposed to TiO2 respect to controls, and LTs were correlated with Ti levels in EBC (Pelclova et al. Citation2012, Citation2016b).
The fractional exhaled nitric oxide (FeNO) measured in EBC and the surfactant proteins in serum (as surfactant protein A (SP-A) and surfactant protein D (SP-D), and Clara cell protein 16 (CC16) in serum were used as biomarkers of airway inflammation and lung disease. The CC16 and FeNO were significantly associated with the handling of TiO2 NMs after adjusting for some confounders (age, gender, smoking habit, history of respiratory disease and dusty environment) (Liao et al. Citation2014). In a follow-up study, on workers exposed to nano-TiO2, nano-SiO2 and nano-Ag, the increase in FeNO values in EBC was observed only in workers exposed to nano-TiO2 (Wu et al. Citation2014). This study suggested that a FeNO value greater than 35 ppb was predictive of airways inflammation.
This last study found that gender, cigarette smoking, asthma, and allergic rhinitis were significant variables for FeNO. Liu et al. (Citation2012) found a positive association between serum SP-A and SP-D and the levels of serum In in ITO manufacturing workers.
The cardiovascular diseases biomarkers commonly analyzed in serum were the intercellular cell adhesion molecule (ICAM) and vascular cell adhesion molecule (VCAM) levels, but also fibrinogen, myeloperoxidase (MPO), and heart rate variability. Results showed that ICAM, VCAM, low frequency of heart rate variability, and fibrinogen levels were found associated with occupational exposure to MNMs (as TiO2, SiO2, Ag, Au) (Liao et al. Citation2014; Liou et al. Citation2012; Zhao et al. Citation2018), whilst no significant changes were found for MPO levels in exposed workers (Liao et al. Citation2014; Wu et al. Citation2019). Other cardiovascular disease markers like low-density lipoprotein (LDL) and total cholesterol (TC) determined in blood were found associated with occupational exposure to nano-TiO2 (Zhao et al. Citation2018).
To date, a few studies has investigated DNA damage and methylation alterations in human. With regard to DNA damage, the micronucleus (MN) assay in buccal cells, tail comet parameters in lymphocytes, and oxidized DNA bases (8-oxoGua, 8-oxoGuo, and 8-oxodGuo) measurement in urine were able to detect the higher direct DNA damage in workers producing SiO2 nanoparticles (Ursini et al. Citation2021). Rossnerova et al. (Citation2020) investigated DNA methylation profiles in blood and observed significant differences between the exposed and control groups as a consequence of long-term exposure to Fe, Mn and Si based-NMs, whilst no effects were observed after short-term acute exposure. In the study of Liou et al. (Citation2017) when workers were stratified by MNMs exposure (TiO2, SiO2, and ITO) global DNA methylation levels in white blood cells were significantly lower in each exposed group than in the non-exposed group, except for the TiO2 exposed group. represents how a panel of biomarkers in different matrices can be used to assess the health effect associated to exposure to MNMs.
Figure 3. Biomarkers of effects to metal-based nanomaterials (MNMs) to recognize changes or toxicity occurring in the body.
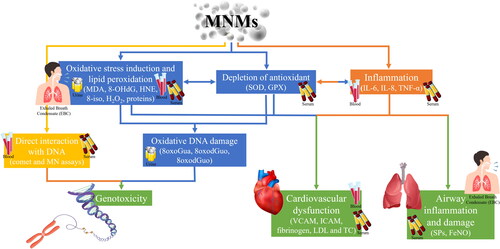
It should be noted that the correlation between MNMs exposure and all the aforementioned biomarkers remains controversial. For example, the studies of Wu et al. (Citation2019) and Liou et al. (Citation2012) did not find changes in inflammation, lung damage and cardiovascular dysfunction biomarkers in workers exposed to Ag, Au, Fe2O3, SiO2, TiO2 nanoparticles respect to controls. Similarly, no difference in biomarkers of oxidative stress and inflammation were found by Ursini et al. (Citation2021) in workers exposed to SiO2 NMs compared to controls. In addition, there were no significant differences in changes between the exposed and control workers for genotoxicity markers (Liao et al. Citation2014; Liou et al. Citation2012; Wu et al. Citation2019).
7. Practical scheme for performing the ‘nanobiomonitoring’
A nanobiomonitoring campaign needs to: (i) recruit the population sample correctly; (ii) choose the most suitable biological matrix on which to perform the measurement; (iii) identify the most appropriate biomarkers reflecting internal dose or effects; (iv) use of sensitive and specific analytical methods; (v) recognize and respect the ethical implications; (vi) communicate the results clearly and appropriately. The designing and undertaking a harmonized human biomonitoring study for chemicals such as MNMs should be a priority objective to support the general population, including the vulnerable groups, and workers (Galea et al. Citation2021). There is a clear need to adapt the human biomonitoring methodologies developed for bulk chemicals and to elaborate the strategies proposed in other studies (Alimonti et al. Citation2011).
Human biomonitoring activities can be divided in health surveillance programmes and research projects with the scope to find the causal links between exposure and health (). Health surveillance consists of hazard surveillance and medical surveillance and can occur at the workplace or at population level. Exposure surveillance is the monitoring of the population for the presence of an environmental NM or its clinically unapparent (for example, subclinical or preclinical) effects; whilst medical surveillance may include clinical examination as well as noting exposures higher than specified levels that determine subsequent actions (including removing the worker from further exposure) (Jones Citation2020).
A nanobiomonitoring study in the context of MNMs can be performed in general population settings, in occupational settings or in targeted surveys to provide enough valid and harmonized information and data for linking exposure to health. It can be structured in various phases () including the selection of biomarkers and related analytical methods and matrices; the integration of ad-hoc questionnaires; the standardization of procedures and materials for collecting biological samples; the development of an analysis plan in collaboration (interlaboratory trials) to verify the quality of the data obtained; and the consideration of ethics and privacy issues (Santonen et al. Citation2022).
Compulsory actions in performing a nanobiomonitoring campaign are (Manno, Sito, and Licciardi Citation2014): (i) select a population potentially exposed to MNMs that have detectable internal dose of MNMs (e.g. nanotechnology companies, welders, etc.) and a control group, preferably which has the same characteristics as those exposed but is not exposed (administrative workers in the company itself) to evaluate the background; (ii) administer questionnaires to all subjects of interest (e.g. workers and company managers) including various questions (e.g. in workplaces: materials used, job description, duties, risk management measures, use of PPE and co-exposure from other sources external to work such as eating habits, food supplements, smoking, dental implants and fillings); (iii) knowledge of the type of MNMs to which subjects are potentially exposed (e.g. synthesized nanoparticles, nanoparticles generated by manufacturing processes, nanoparticles intentionally added in consumer products); (iv) select the matrix on which to carry out the measurements that must be as accessible as possible, available in sufficient quantities, sampling of which is less invasive as possible and does not present any health risks to subjects. The choice of the matrix also depends on the type of NM and its characteristics (e.g. half-life and toxicokinetics).
Various compartments as EBC, blood, serum and urine may represent indicators of exposure (Iavicoli et al. Citation2014). In particular, EBC appears as a noninvasively collected biological matrix able to detect both biomarkers of exposure and effect (Lee et al. Citation2015; Leese et al. Citation2023; Pelclova et al. Citation2015; Marie-Desvergne et al. Citation2016; Wu et al. Citation2014). Blood usually represented an ideal matrix for many chemicals due to its interaction with the organism and its chemical equilibrium with organs and tissues. Blood-based biomarkers may prove useful when more specific information on systemic and intracellular bioavailability is necessary (Ndaw et al. Citation2022). However, blood has an important disadvantage of requiring an invasive sampling and being challenging to use in occupational or environmental settings. In addition it has been demonstrated that only the smallest MNMs (ca. 30 nm) have a translocation potential through the bloodstream, thus generally fewer particles were detected in blood and/or serum than those found in EBC or organs as lung, intestine, or skin (Bocca, Leso, et al. Citation2023; Balasubramanian et al. Citation2013; Bachler et al. Citation2015; Miller et al. Citation2017). Urine is also a noninvasive matrix and requires reduced sample manipulation and a reduced risk of pre-analytical artifacts compared to blood. But concerning urine, it was demonstrated that the kidney glomerular filtration is highly dependent on the size of particles: a diameter <6 nm is typically filtered and excreted via the renal system, while those >8 nm are not normally capable of glomerular filtration and are eliminated via the hepatobiliary system (Iavicoli, Leso, and Schulte Citation2016; Longmire, Choyke, and Kobayashi Citation2008). Moreover, some oxidative stress biomarkers (e.g. HNE and other aldehydes) are intermediary oxidation products and their overall concentrations in urine may be not high to detect biomarkers changes in this matrix (Luo et al. Citation2022).
The half-life and toxicokinetics of a biomarker in a given matrix is fundamental to select the appropriate sampling time, and is essential for interpreting the human biomonitoring data. The longer half-lives allow detection of exposures in the past or cumulative exposures. In occupational settings, for chemicals half-life between 2 and 10 h, the sampling should be performed after work or the day after; for half-life between 10 and 100 h, the sampling is better at the end of the week; for half-life >100 h, the sampling time is not critical (Vorkamp et al. Citation2021). With reference to biomarkers of effect, the half-lives of oxidative stress biomarkers in plasma were found to be longer than that in urine, which may result in their low levels in urinary samples (Brunmark et al. Citation1995). Zhang et al. (Citation2022) found that o-Tyr and 5-OHMeU in urine were not statistically elevated in the longest sampling time points, whilst urinary 8-OHG and HNE had a slower urinary excretion kinetics and can be used to assess both the acute and chronic exposure in NMs exposed workers (Zhang et al. Citation2022). From previous results within the EU human biomonitoring initiative (HBM4EU), the complementary role of different types of biomarkers clearly emerged, especially in the case of the limited toxicokinetic information on MNMs (Bocca, Leso, et al. Citation2023; Bocca, Battistini, et al. Citation2023; Ndaw et al. Citation2022).
Moreover, a nanobiomonitoring program has ethical and social implications as human samples are collected and analyzed and personal data that may involve sensitive sections of the population (children) are used (Manno, Sito, and Licciardi Citation2014). Research must be undertaken in accordance with standards of good ethical and social practice to best protect participants. The principle of voluntary participation requires that people are not forced to participate in research. Closely related to the notion of voluntary participation is the requirement of informed consent. In essence, potential research participants need to be fully informed about the procedures and risks. Ethical standards also require that researchers do not put participants in a situation where they could be at risk of harm due to their participation. Participants are assured their privacy is respected, therefore the identification information is not made available and the samples are anonymous and shared (Tolonen et al. Citation2022). It is also important to consider the additional ethical complications in interventions to protect individuals who are found to present higher exposure without causing discrimination, especially at work.
Finally, the communication of the risk of nanobiomonitoring results must take place in a structured and systematic way for a clear and widespread understanding of the results of scientific research, without creating alarmism. This is an intentional exchange of information between the stakeholders on different aspects including levels and significance of risk to health or the environment, as well as decisions and actions aimed at their management or control (Laux et al. Citation2018). Some communication issues to follows are: (i) set out the communication agreement up front; (ii) communicate as agreed; (iii) communicate fully, openly and in a timely fashion; (iv) communicate simply, using two-way tools (there are no stupid questions); (v) follow-up; get back with answers; (vi) involve all levels within community and institutions.
8. Characterization of MNMs in human samples
To be useful for the aforementioned purposes, the nanobiomonitoring and biomarkers assessment should be based on reproducible sample preparations and analytical protocols adapted to the specific characteristic of particles (size, shape, agglomeration, surface charge, etc.) and concentration range of interest and using available and rapid tools. Another important prerequisite for conducting reliable and robust biomarker assessments is the availability of well-developed guidance documents and SOPs. The SOPs have so far been done only in few cases. The NanoDefine project (https://cordis.europa.eu/project/id/604347) aimed to develop an integrated approach consisting of a continuous performance evaluation of relevant measurement techniques, improvement of available software and instruments, and of proper sample preparation methods. The NANoREG project included deliverables on SOPs for nanoparticles dispersion and characterization (e.g. for the quantitative size and shape analysis) (https://www.bmuv.de/fileadmin/Daten_BMU/Download_PDF/Nanotechnologie/nanoreg_uebersicht_en_bf.pdf). One fundamental activity of the QualityNano project is the establishment of quality control and quality assurance conditions for NMs safety and assessment (https://cordis.europa.eu/project/id/262163). At the global level, the OECD published a guidance document on sample preparation and dosimetry but as stated by OECD it is does not aim to be conclusive due to the diversity of types of NMs (OECD Citation2012).
8.1. Measurement techniques
For a correct execution of biomarkers analysis, the samples must be collected in such a way as to preserve their quality and reduce the external contamination to the minimum. Many aspects of sample collection are not nano-specific, e.g. metal-free containers can be used like a typical total trace metal analysis in a biological sample. Sources of external contamination include the laboratory equipment and tools used and the common procedure of the acid-washing may be sufficient for experiments with MNMs (Laycock et al. Citation2022). Appropriate storage is an important aspect for preservation of biological samples to ensure the original MNMs will not change through dissolution, chemical transformation and agglomeration/aggregation. The effect of storage conditions on MNMs in biological matrices has not been thoroughly investigated. Loeschner et al. (Citation2013) observed that Ag NMs in chicken meat could not be recovered after long-term (10 months) storage at −80 °C. The container used for storage the biological samples may influence the recovery of MNMs. It was demonstrated that storage in a plastic container resulted in nearly a two-fold better mass recovery for TiO2 NMs compared to glassware (Shaw et al. Citation2013).
Also the sample preparation method () should preserve the integrity of the nanoform. The preparation method should not create conditions whereby particle aggregation/agglomeration occurs or indeed promote any chemical reaction that might cause particle formation leading to false positives (Laycock et al. Citation2022). The ISO 14887:2007 outlines procedures for the preparation of good dispersions from various powder/liquid combinations for particle size analysis of substances in general (ISO Citation2007). Suggested dispersion procedures for a range of nanomaterials are also emerging in the scientific literature e.g. in Hartmann et al. (Citation2015). In addition, in order to start with relevant sample preparation the OECD Guidance on Sample Preparation and Dosimetry for the Safety Testing of Manufactured Nanomaterials should be considered (OECD Citation2012). In literature it was found that the Inductively Coupled Plasma Mass Spectrometry (ICP-MS) analysis of MNMs in urine, serum and EBC analysis required a simple dilution of the sample with ultrapure deionized water (Bocca, Battistini, and Petrucci Citation2020; Badalova et al. Citation2019; Huang, Tsz-Shan Lum, and Sze-Yin Leung Citation2020; Salou et al. Citation2020). After each dilution step, vortexing for 1 minute and ultrasonic sonication for 10 min of the samples ensures the homogeneous dispersion and prevents agglomeration of particles (Shaw et al. Citation2013). For more complex biological tissues as blood, organs and skin it is necessary to develop digestion or extraction procedures. The use of an acid solution allows the digestion of the organic component of the matrix and the subsequent ICP-MS analysis provided the total metal quantification including the dissolved nanoforms (Laycock et al. Citation2022). Frequently the metal ion form is indeed used as indicative of MNMs exposure, after qualitative identification of nanosized particles with microscopic techniques (Gulson et al. Citation2010; Heringa et al. Citation2018; Pele et al. Citation2015; Peters et al. Citation2020). Blood and tissue samples were extracted with an aqueous solution of tetramethylammonium hydroxide (TMAH) and Triton-X in several cases (Bocca, Battistini, and Petrucci Citation2020; Neubauer and Chady Citation2014; Roman et al. Citation2016; Witzler, Küllmer, and Günther Citation2018). To complete the extraction process, Bocca et al. (Citation2020) sonicated the solution and left it for 24 h at room temperature; while Roman et al. (Citation2016) added a NH4OH solution. The extraction protocols based on the use of enzymes, such as proteinase K or pancreatic added with lipase, were applied especially for tissues (Laycock et al. Citation2022). Despite this, the enzymatic approach has some limitations as the long times of incubation, the specificity of the enzyme for the tissue, e.g. proteinase K may be not appropriate for liver extraction (Clark et al. Citation2019) and the possible lower mass recoveries respect to alkaline extraction (Loeschner et al. Citation2013; Sung et al. 2018). Regarding the selection of the eluent in pre-separation systems as the field-flow fractionation (FFF), the chosen carrier solution should mimic the matrix in which the MNMs are suspended (i.e. pH, ionic strength, etc.) in order to avoid altering NMs properties, such as surface charge, double layer thickness, particle aggregation/disaggregation and dissolution (Gigault et al. Citation2014). The application of ultrasound or probe sonication is often required to break up agglomerates present in the suspension, but the sonication characteristics and parameters selected can influence the physico-chemical properties of dispersed MNMs (Cohen et al. Citation2013; Ghomrasni et al. Citation2020). Vidmar et al. (Citation2016) observed that the use of a sonication probe resulted in the most favorable stabilizing conditions when water was used as a dispersion medium. Recently it was found that adding to blood and urine the polyvinylpyrrolidone (PVP) at a nominal concentration of 2 wt% and sonicating the suspension in pulse mode with a probe obviated TiO2 nanoparticles agglomeration and aggregation, and minimized the risk of overestimation of size, for at least 72 h (Salou et al. Citation2020). But an uncontrolled sonication method can induce processes of re-agglomeration of nanoparticles (Mandzy, Grulke, and Druffel Citation2005). For example, in the case of Cu, Al, Mn, ZnO particles the probe sonication led to an extensive agglomeration and a rapid sedimentation with dose alteration of 30-80% (Pradhan et al. Citation2016). This last paper reported that the addition of a stabilizing agent like the bovine serum albumin (BSA) had an accelerating effect on the release of metals in sonicated solutions. Other means of dispersion such as ultrasonic bath, vortexing, and manual shaking were also investigated (Bocca, Battistini, and Petrucci Citation2020). In general, the harmonization and standardization of dispersion methods applied in MNMs testing are needed to ensure a comparable data quality and to minimize test artifacts produced by modifications of MNMs during the dispersion preparation process (Hartmann et al. Citation2015).
Table 5. Current methods for metal-based nanomaterials (MNMs) measurements.
Advantages and limitations of the available techniques the determination and characterization of MNMs in biological matrices are reported in . Characterization in biological matrices requires chemical (i.e. composition and concentration) and physical (i.e. size, shape, aggregation, etc.) information, which cannot be achieved by the use of a single analytical method but by a combination of analytical techniques. The European Chemicals Agency (ECHA) stressed that the use of several analytical techniques for characterizing nanoforms (multi-method approach) was favored, and bias from one technique could be minimized by the use of multiple techniques, providing new and more detailed information (ECHA Citation2013). In the case of nanobiomonitoring studies it is fundamental to develop high-sensitivity and low-background analytical techniques considering the low levels of MNMs in biological matrices. Thus, among the challenges of nanoanalytics there is the need to measure at the trace level, the limited availability of standards and certified reference materials (CRMs) for the nanoforms, and the poor participation to interlaboratory circuits for the reliability of MNM measurements. The National Institute of Standards and Technology (NIST) produced reference materials for MNMs (https://shop.nist.gov/ccrz__ProductList?cartID=&portalUser=&store=&cclcl=en_US&operation=quickSearch&searchText=nanoparticles) as well as the Joint Research Center (JRC) provided a list of MNMs hosted in the JRC Repository and their main physico-chemical characteristics (https://joint-research-center.ec.europa.eu/system/files/2016-06/JRC%2520Nanomaterials%2520Repository-List%2520of%2520Representative%2520Nanomaterials-201606.pdf). However, the availability of test materials that mimic MNMs in real human biological matrix, including different metals, broad size ranges, different concentrations and different shapes is still lacking. As stated by the OECD, test materials should be adequately characterized, should represents a realistically conservative form of the material to which an individual or organism has been exposed, and should be adequately representative of the test material used in exposure and effects testing, and, in addition, sufficient dose ranges are needed to adequately characterize the dose-response relationship (OECD Citation2022).
Regarding the interlaboratory trials, one recent interlaboratory study explored the transport efficiency of Au nanoparticle suspensions in seven European and US expert laboratories using different ICP-MS instruments (Geiss et al. Citation2022). Another interlaboratory study investigated the reproducibility on modal particle size using nanoparticle tracking analysis (NTA) on a range of particle sizes and in several different media (Hole et al. Citation2013). The characterization of Si nanoparticles mixture relied on an interlaboratory comparison study in which 30 laboratories of demonstrated competence participated with a variety of techniques for particle size analysis (Kestens et al. Citation2016). In addition, within the NanoValid project a consortium of six partners jointly investigated the hazard of Ag nanoparticles paying special attention to methodical aspects (Jemec et al. Citation2016). This last study concluded that harmonized procedures should consider the measuring of Ag nanoparticles properties like hydrodynamic size and the presence of metal ions species in test medium at a relevant range of concentrations.
Among the analytical techniques, microscopy is an established method for determining the size and morphology of MNMs. The size and particle size distribution as determined by microscopy methods like TEM are the most reliable and most similar to the real physical size (diameter equivalent to the area or Feret diameter) (Mast, Verleysen, and De Temmerman Citation2015). However, the analyses are for dry samples and the particle behaviors in solution may be different, removing the solvent may indeed increase their aggregation and agglomeration in the original samples. For the analysis of morphology and surface properties the SEM-EDX (scanning electron microscope-energy dispersive X-ray spectroscopy) was applied by Locci et al. (Citation2019). The results showed the presence of MNMs aggregates in human kidney samples with a very low resolution (<0.6 nm) (Locci et al. Citation2019). Light scattering is one of the most common methods for determining the mean and/or median particle size and the particle size distribution (PSD) in dispersions with a relatively fast measurement, and with the automation of measurements is not necessary an extensive experience for this kind of analysis. The DLS and NTA permitted the evaluation of the hydrodynamic radius (Rh) of particles whilst MALS the gyration radius (Rg) and the ratio Rg/Rh allowed to evaluate the shape of particles. The value of the ratio Rg/Rh increases with rising anisomery and for spheres the value is 0.778 (Kratochvíl and Netopilík Citation2017). However, there are some limitations when applied to complex samples. A major drawback of light scattering methods is that the results are strongly biased by the presence of larger particles or aggregates that can mask the presence of smaller nanoscale particles (Moore and Cerasoli Citation2017). Compared to DLS, the detection limit for NTA for particle size is generally higher but it can better handle polydisperse samples (Filipe, Hawe, and Jiskoot Citation2010). The atomic force microscope method (AFM) is potentially promising for MNMs that do not readily aggregate, and it is used to determine the shape, size and/or size distribution of MNMs, such as Al2O3, TiO2, ZnO (Rao et al. Citation2007).
Zeta potential measurement, combined with DLS, can be used to explore the stability of nanoparticle dispersions and for the qualitative understanding of the agglomeration process (Bhattacharjee Citation2016). The agglomeration or aggregation state is also determined by measuring light scattering properties or by electron microscopy (De Temmerman et al. Citation2012).
The single particle-ICP-MS (SP-ICP-MS) system allows us to distinguish between dissolved metal signals and nonforms and to simultaneously determine both the number concentration and the size distribution of particles. Thanks to its speed (a few seconds) and the high sensitivity it allows the determination of Ag, Au, Cr2O3, Mn3O4, NiO particles in biological tissues at low concentration level (Bocca, Leso, et al. Citation2023; Bocca, Battistini, and Petrucci Citation2020; Badalova et al. Citation2019; Gray et al. Citation2013; Ishizaka et al. Citation2019). The main limitations of SP ICP-MS method is that the size detection limit is element specific and influenced by the presence of background dissolved ions and it cannot distinguish aggregates from large-size particles. Also, the assumptions about particle composition, shape and size made during the calculation may not justify the use of SP-ICP-MS analysis alone, requiring further confirmation from other characterization methods like TEM (Gao and Lowry Citation2018). In a recent survey, SP-ICP-MS analysis showed accurate (76–122%) and precise (<5.2%) particle measurements for Ag 20, 60 and 100 nm and for Au 5, 20, 40 and 60 nm in urine, serum and blood samples (Bocca, Battistini, and Petrucci Citation2020). The SP-ICP-MS determination of oxide nanoparticles of Cr2O3 (60 nm), Mn3O4 (30 nm) and NiO (18 nm) provided recoveries between 98–129%, 104–149% and 100–160% and precisions between 5.8–8.6%, 4.0–9.4% and 3.9–8.6% in EBC, plasma and urine, respectively (Bocca, Leso, et al. Citation2023).
The pre-separation step prior to detection by FFF or size-exclusion chromatography (SEC) is an effective tool for separating complex mixtures of MNMs of different sizes and in highly polydispersity samples. The SEC is a column liquid chromatographic technique based on exclusion of the molecules from the pores of the stationary phase. The larger molecules are elute before the smaller ones because larger molecules either enter fewer pores (Pitkänen and Striegel Citation2016). In FFF the separation is achieved in a flat channel by means of an externally generated cross-flow applied in a perpendicular direction across a flat semi-permeable membrane (ISO Citation2018a). The cross-flow allows the smaller particles to be eluted at shorter times, as they will move faster along the channel, compared to the larger (Bocca, Battistini, and Petrucci Citation2020). Both the SEC and the FFF system are separation techniques and therefore must be coupled with other detectors that perform sizing as DLS or MALS, and detection and quantification as ultraviolet-visible (UV-Vis) spectroscopy and ICP-MS. The UV-Vis has been used coupled on-line with the SEC for the analysis of Au, CdS, CdSe, CeO2, Pd, ZnS NMs (Pitkänen and Striegel Citation2016) and with FFF for the characterization of Au and Ag NMs in urine and blood (Bocca, Battistini, and Petrucci Citation2020) but the applicability is limited by the low sensitivity and the absence of any specificity of the UV detector. The FFF-MALS-ICP-MS coupling instead was specific for metals and represents a quantitative method allowing the detection of Al, Ag, Au, Cu, SiO2, TiO2, ZnO NMs in the range from μg/L to ng/L and from 5 nm to 100 nm (Bocca et al. Citation2017; Bocca et al. Citation2018; Bocca, Battistini, and Petrucci Citation2020; Heroult et al. Citation2014). On the other hand, the concentration detection limit of the FFF technique can be a limiting factor, probably due to the high dilution in the FFF channel during separations. A study that applied FFF separations observed a partial recovery of Au and Ag nanoparticles (i.e. 30%–50%) spiked in blood, serum and urine, the lengthy of the separation process (40–50 min), and the need for extra cost and specialized personnel (Bocca, Battistini, and Petrucci Citation2020). Many studies reported that the optimization of FFF analysis is very critical as the parameters to be controlled are numerous (selection of eluent, membrane and spacer channel height, cross flow gradient, etc.), specific for each metal and still poorly understood (Baalousha, Stolpe, and Lead Citation2011; Bocca et al. Citation2018; Bocca et al. Citation2017; Mitrano et al. Citation2012).
8.2. Method validation
The validation of any method in matrix is an important part of the quality assurance (QA) in ‘nanobiomonitoring’ including the detailed documentation and reporting of all relevant steps in the MNMs measurement process. In addition, well-maintained instruments and trained staff are other prerequisites for any NMs measurement result to be meaningful. Specific guidance on QA for MNMs are lacking, but the ISO/IEC 17025 ‘General requirements for the competence of testing and calibration laboratories’ developed by ISO (ISO Citation2018b) and the Principles of Good Manufacturing Practice (GMP) (EC Citation2003, Citation2014) and Good Laboratory Practice (GLP) developed under OECD (OECD Citation1998) can be utilized.
According to the ISO/IEC 17025 (ISO Citation2018b) the following parameters should be at least determined: (i) precision: it identifies the similarities of replicate measurements results made on a similar sample; (ii) the working range: represents the range of concentrations that can be measured reliably. It also covers the limit of detection (LoD) and quantification (LoQ); (iii) selectivity/specificity: it confirms whether there any interferences and defines whether laboratories are measuring what they think they are measuring; (iv) trueness/recovery: it identifies how close are the results to the correct value; (v) ruggedness/robustness: it provides the capacity of the method to remain unaffected by small and deliberate variations in method parameters. Few papers report these parameters for MNMs in human biological matrices. These parameters were recently validated for Ag and Au NPs in human biological fluid samples. The SP ICP-MS method allowed to obtain a rapid dimensional screening of MNMs in biological samples with a LoD in size of ca. 20 nm and a LoD in concentration of ca.103 particles/mL (Bocca, Battistini, and Petrucci Citation2020; Badalova et al. Citation2019; Mitrano et al. Citation2012). Acceptable concentrations recoveries from spike tests for MNMs in urine, serum, blood and biological tissue are 100 ± 25% with a RSD% in particle counting < 5% (Bocca, Battistini, and Petrucci Citation2020; Gray et al. Citation2013). Also the accuracy on particle sizes was 100 ± 25%, but for the smaller MNMs (proximally to size LoD) a shift to larger diameters was possible especially in a context of biological matrices rich in salts, proteins, lipids, etc (Bocca Battistini, and Petrucci Citation2020; Liu et al. Citation2017).
The ISO/TS 21362:2018 identified parameters and conditions necessary to develop and validate methods for the application of the FFF technique to the analysis of NMs in aqueous media (ISO Citation2018a). Accordingly to the ISO 21362:2018, method performances criteria, other than precision, trueness and selectivity, should be determined as the void peak retention time, retention time of nanoparticles, and the resolution factor (defined as the ratio of the difference in retention time to the average of the peak widths measured as the full width at half maximum for two adjacent eluting analytes). In the paper of Battistini, Petrucci, and Bocca (Citation2021) the high selectivity of the FFF analysis of polystyrene nanoplastics was reflected by the large changes in retention time values with small variations in particle size. Thanks to the FFF analysis it was possible to extend the analysis to particles < 5 nm improving the dimensional detection limits (Baalousha and Lead Citation2007; Bocca, Battistini, and Petrucci Citation2020). The LoD in concentration with FFF-ICP-MS was ca.106 particles/mL for Au and Ag NMs in blood, serum and urine (Bocca, Battistini, and Petrucci Citation2020). The recovery was found better for smaller particles, probably due to the tendency of larger particles to remain closer to the FFF channel membrane (Schmidt et al. Citation2011).
9. Present and future challenges
There is increasing public, scientific and governmental interest in the potential adverse health effects of MNMs exposure. Human biomonitoring offers the opportunity to integrate or even supplement epidemiology, environment monitoring and in vitro and animal models achieving a more accurate and confident risk assessment to MNMs in general and occupational settings. Indeed, a biomarker of exposure gives an assessment of the absorbed dose of MNMs in human, whilst a biomarker of effect gives an assessment of the adverse early and reversible health effects (subclinical or preclinical changes). Thus, the combination of exposure and effect biomarkers improves the knowledge of current or past human exposure to MNMs and postulates pathways and causes of the observed adverse health effects, playing an important part in primary prevention (e.g. by limiting exposure to the dangerous MNMs and anticipating the late adverse effects).
Physicochemical properties and toxicokinetic of MNMs significantly influence the levels of biomarkers in the matrix and the sampling time window. Thus, biomarkers of exposure and effect have similar developmental requirements including the knowledge of size, shape, surface area, charge, etc. of the MNM, and its tissue penetration, biodistribution, circulation half-life, and accumulation at target sites.
A combination of biomarkers - the most relevant being oxidative stress/antioxidant capacity indicators, inflammatory mediators, cardiovascular disease markers, as well as oxidized DNA bases – are currently used to associate exposure to MNMs and health effects. The EBC matrix appears an extremely promising matrix for the noninvasiveness of sampling and biomarkers in EBC (as MDA, 8-OHdG, 8-iso, HNE) may directly reflect airway inflammation and lung oxidative stress. In addition, biomarkers in blood/serum (as SOD, GPX, cytokines, proteins, ICAM, VCAM) or in urine (8-OHdG, 8-oxoGua, 8-oxoGuo and 8-oxodGuo) may predict various damaged conditions (oxidation, DNA damage, inflammation, cardiovascular dysfunction). Apparently, biomarkers of MNMs measured in EBC and blood samples yield more significant outcomes as compare to biomarkers in urine, but validation and confirmation with studies designed on a larger population are needed.
Notably, very few studies examined the relationship between exposure and effect biomarkers to provide valuable understanding of the actual MNMs exposure and health relevance of such exposure, and findings are often contradictory. This fact can be considered associated to reasons as: (i) the limited number of individuals collected; (ii) measuring biomarkers and their physico-chemical properties is not an easy task; (iii) procedures including considering the sampling time windows and analytical methods were not standardized; (iv) there is a limited consistency in how the results are reported; (v) biomarkers concentration are not always corrected for possible confounding and background exposures; (vi) the reference range for biomarkers, essential for a meaningful interpretation of data, are scarcely explored.
Therefore, future efforts are needed to involve higher number of individuals (exposed and controls), design harmonized procedures at every step (including interlaboratory trials and certified reference materials), and develop reference limits, in order to be able to produce reliable and comparable human biomonitoring data. Importantly, the observed changes in biomarkers level along with their health significance should be assessed in long-term perspective studies, through analytical techniques sensitive enough to identify trace levels of biomarkers, predictive and applicable to subclinical or preclinical testing.
Disclosure statement
No potential conflict of interest was reported by the author(s).
Additional information
Funding
References
- Abbott, L. C., and A. D. Maynard. 2010. “Exposure Assessment Approaches for Engineered Nanomaterials: Perspective.” Risk Analysis 30 (11): 1634–1644. https://doi.org/10.1111/j.1539-6924.2010.01446.x
- Ahmad, J., M. A. Siddiqui, M. J. Akhtar, H. A. Alhadlaq, A. Alshamsan, S. T. Khan, R. Wahab, et al. 2018. “Copper Doping Enhanced the Oxidative Stress-Mediated Cytotoxicity of TiO2 Nanoparticles in A549 Cells.” Human & Experimental Toxicology 37 (5): 496–507. https://doi.org/10.1177/09603271177140
- Alam, M., T. Alshehri, J. Wang, S. A. Singerling, C. N. Alpers, and M. Baalousha. 2023. “Identification and Quantification of Cr, Cu, and as Incidental Nanomaterials Derived from CCA-Treated Wood in Wildland-Urban Interface Fire Ashes.” Journal of Hazardous Materials 445: 130608. https://doi.org/10.1016/j.jhazmat.2022.130608
- Alimonti, A., B. Bocca, D. Mattei, and A. Pino. 2011. “Programme for Biomonitoring the Italian Population Exposure (PROBE): Internal Dose of Metals.” Rapporti ISTISAN 11(9): 1–85.
- Arenas-Vivo, A., G. Amariei, S. Aguado, R. Rosal, and P. Horcajada. 2019. “An Ag-Loaded Photoactive Nano-Metal Organic Framework as a Promising Biofilm Treatment.” Acta Biomaterialia 97: 490–500. https://doi.org/10.1016/j.actbio.2019.08.011
- Augustine, R., A. Hasan, R. Primavera, R. J. Wilson, A. S. Thakor, and B. D. Kevadiya. 2020. “Cellular Uptake and Retention of Nanoparticles: Insights on Particle Properties and Interaction with Cellular Components.” Materials Today Communications 25: 101692. https://doi.org/10.1016/j.mtcomm.2020.101692
- Baalousha, M., and J. R. Lead. 2007. “Characterization of Natural Aquatic Colloids (<5 nm) by Flow-Field Flow Fractionation and Atomic Force Microscopy.” Environmental Science & Technology 41 (4): 1111–1117. https://doi.org/10.1021/es061766n
- Baalousha, M., B. Stolpe, and J. R. Lead. 2011. “Flow Field-Flow Fractionation for the Analysis and Characterization of Natural Colloids and Manufactured Nanoparticles in Environmental Systems: A Critical Review.” Journal of Chromatography. A 1218 (27): 4078–4103. https://doi.org/10.1016/j.chroma.2011.04.063
- Bachler, G., S. Losert, Y. Umehara, N. von Goetz, L. Rodriguez-Lorenzo, A. Petri-Fink, B. Rothen-Rutishauser, and K. Hungerbuehler. 2015. “Translocation of Gold Nanoparticles across the Lung Epithelial Tissue Barrier: Combining in Vitro and in Silico Methods to Substitute in Vivo Experiments.” Particle and Fibre Toxicology 12 (1): 18. https://doi.org/10.1186/s12989-015-0090-8
- Badalova, K., P. Herbello-Hermelo, P. Bermejo-Barrera, and A. Moreda-Piñeiro. 2019. “Possibilities of Single particle-ICP-MS for Determining/Characterizing Titanium Dioxide and Silver Nanoparticles in Human Urine.” Journal of Trace Elements in Medicine and Biology 54: 55–61. https://doi.org/10.1016/j.jtemb.2019.04.003
- Balasubramanian, S. K., K.-W. Poh, C.-N. Ong, W. G. Kreyling, W.-Y. Ong, and L. E. Yu. 2013. “The Effect of Primary Particle Size on Biodistribution of Inhaled Gold Nano-Agglomerates.” Biomaterials 34 (22): 5439–5452. https://doi.org/10.1016/j.biomaterials.2013.03.080
- Battistini, B., F. Petrucci, and B. Bocca. 2021. “In-House Validation of AF4-MALS-UV for Polystyrene Nanoplastic Analysis.” Analytical and Bioanalytical Chemistry 413 (11): 3027–3039. https://doi.org/10.1007/s00216-021-03238-2
- Battistini, B., F. Petrucci, I. De Angelis, C. M. Failla, and B. Bocca. 2020. “Quantitative Analysis of Metals and Metal-Based Nano- and Submicron-Particles in Tattoo Inks.” Chemosphere 245: 125667. https://doi.org/10.1016/j.chemosphere.2019.125667
- Bergamaschi, E., V. Bellisario, M. Macrì, M. Buglisi, G. Garzaro, G. Squillacioti, F. Ghelli, et al. 2022. “A Biomonitoring Pilot Study in Workers from a Paints Production Plant Exposed to Pigment-Grade Titanium Dioxide (TiO2).” Toxics 10 (4): 171. https://doi.org/10.3390/toxics10040171
- Bergamaschi, E., M. Gulumian, J. Kanno, and K. Savolainen. 2019. “Engineered Nanomaterials: Biomarkers of Exposure and Effect, in: Biomarkers in Toxicology.” Biomarkers in Toxicology 41: 735–755. https://doi.org/10.1016/B978-0-12-814655-2.00041-4
- Bergamaschi, E., C. Poland, I. Guseva Canu, and A. Prina-Mello. 2015. “The Role of Biological Monitoring in Nano-Safety.” Nano Today.10 (3): 274–277. https://doi.org/10.1016/j.nantod.2015.02.001
- Bhattacharjee, S. 2016. “DLS and Zeta Potential – What They Are and What They Are Not?” Journal of Controlled Release 235: 337–351. https://doi.org/10.1016/j.jconrel.2016.06.017
- Bhattacharjee, S., L. H. de Haan, N. M. Evers, X. Jiang, A. T. Marcelis, H. Zuilhof, I. M. Rietjens, and G. M. Alink. 2010. “Role of Surface Charge and Oxidative Stress in Cytotoxicity of Organic Monolayer-Coated Silicon Nanoparticles towards Macrophage NR8383 Cells.” Particle and Fibre Toxicology 7 (1): 25. https://doi.org/10.1186/1743-8977-7-25
- Bocca, B., V. Leso, B. Battistini, S. Caimi, M. Senofonte, M. Fedele, D. M. Cavallo, A. Cattaneo, P. Lovreglio, and I. Iavicoli. 2023. “Human Biomonitoring and Personal Air Monitoring. An Integrated Approach to Assess Exposure of Stainless-Steel Welders to Metal-Oxide Nanoparticles.” Environmental Research 216 (Pt 3): 114736. https://doi.org/10.1016/j.envres.2022.114736
- Bocca, B., B. Battistini, V. Leso, L. Fontana, S. Caimi, M. Fedele, and I. Iavicoli. 2023. “Occupational Exposure to Metal Engineered Nanoparticles: A Human Biomonitoring Pilot Study Involving Italian Nanomaterial Workers.” Toxics 11 (2): 120. https://doi.org/10.3390/toxics11020120
- Bocca, B., F. Barone, F. Petrucci, F. Benetti, V. Picardo, V. Prota, and G. Amendola. 2020. “Nanopesticides: Physico-Chemical Characterization by a Combination of Advanced Analytical Techniques.” Food and Chemical Toxicology 146: 111816. https://doi.org/10.1016/j.fct.2020.111816
- Bocca, B., B. Battistini, and F. Petrucci. 2020. “Silver and Gold Nanoparticles Characterization by SP-ICP-MS and AF4-FFF-MALS-UV-ICP-MS in Human Samples Used for Biomonitoring.” Talanta 220: 121404. https://doi.org/10.1016/j.talanta.2020.121404
- Bocca, B., S. Caimi, O. Senofonte, A. Alimonti, and F. Petrucci. 2018. “ICP-MS Based Methods to Characterize Nanoparticles of TiO2 and ZnO in Sunscreens with Focus on Regulatory and Safety Issues.” The Science of the Total Environment 630: 922–930. https://doi.org/10.1016/j.scitotenv.2018.02.166
- Bocca, B., E. Sabbioni, I. Mičetić, A. Alimonti, and F. Petrucci. 2017. “Size and Metal Composition Characterization of Nano- and Microparticles in Tattoo Inks by a Combination of Analytical Techniques.” Journal of Analytical Atomic Spectrometry 32 (3): 616–628. https://doi.org/10.1039/C6JA00210B
- Borak, B., P. Biernat, A. Prescha, A. Baszczuk, and J. Pluta. 2012. “In Vivo Study on the Biodistribution of Silica Particles in the Bodies of Rats.” Advances in Clinical and Experimental Medicine 21: 13–18.
- Braakhuis, H. M., M. V. Park, I. Gosens, W. H. De Jong, and F. R. Cassee. 2014. “Physicochemical Characteristics of Nanomaterials That Affect Pulmonary Inflammation.” Particle and Fibre Toxicology 11 (1): 18. https://doi.org/10.1186/1743-8977-11-18
- Breza, M., and P. Šimon. 2020. “On Shape Dependence of the Toxicity of Rutile Nanoparticles.” Journal of Nanoparticle Research 22 (3): 58. https://doi.org/10.1007/s11051-020-4773-1
- Brunmark, P., M. Bruze, S. Skerfving, and G. Skarping. 1995. “Biomonitoring of 4,4’-Methylene Dianiline by Measurement in Hydrolysed Urine and Plasma after Epicutaneous Exposure in Humans.” International Archives of Occupational and Environmental Health 67 (2): 95–100. https://doi.org/10.1007/BF00572232
- Calatayud, M. P., B. Sanz, V. Raffa, C. Riggio, M. R. Ibarra, and G. F. Goya. 2014. “The Effect of Surface Charge of Functionalized Fe3O4 Nanoparticles on Protein Adsorption and Cell Uptake.” Biomaterials 35 (24): 6389–6399. https://doi.org/10.1016/j.biomaterials.2014.04.009
- Centers for Disease Control and Prevention (CDC). 2005. “Third National Report on Human Exposure to Environmental Chemicals.” Atlanta, Georgia: CDC, National Center for Environmental Health.
- Cheng, T.-H., F.-C. Ko, J.-L. Chang, and K.-A. Wu. 2012. “Bronchiolitis Obliterans Organizing Pneumonia Due to Titanium Nanoparticles in Paint.” The Annals of Thoracic Surgery 93 (2): 666–669. https://doi.org/10.1016/j.athoracsur.2011.07.062
- Choi, H. S., Y. Ashitate, J. H. Lee, S. H. Kim, A. Matsui, N. Insin, M. G. Bawendi, M. Semmler-Behnke, J. V. Frangioni, and A. Tsuda. 2010. “Rapid Translocation of Nanoparticles from the Lung Airspaces to the Body.” Nature Biotechnology 28 (12): 1300–1303. https://doi.org/10.1038/nbt.1696
- Clark, N. J., R. Clough, D. Boyle, and R. D. Handy. 2019. “Development of a Suitable Detection Method for Silver Nanoparticles in Fish Tissue Using Single Particle ICP-MS.” Environmental Science: Nano 6 (11): 3388–3400. https://doi.org/10.1039/C9EN00547A
- Cohen, J., G. DeLoid, G. Pyrgiotakis, and P. Demokritou. 2013. “Interactions of Engineered Nanomaterials in Physiological Media and Implications for in Vitro Dosimetry.” Nanotoxicology 7 (4): 417–431. https://doi.org/10.3109/17435390.2012.666576
- Colvin, V. L. 2003. “The Potential Environmental Impact of Engineered Nanomaterials.” Nature Biotechnology 21 (10): 1166–1170. https://doi.org/10.1038/nbt875
- De Temmerman, P.-J., E. Van Doren, E. Verleysen, Y. Van Der Stede, M. A. D. Francisco, and J. Mast. 2012. “Quantitative Characterization of Agglomerates and Aggregates of Pyrogenic and Precipitated Amorphous Silica Nanomaterials by Transmission Electron Microscopy.” Journal of Nanobiotechnology 10 (1): 24. https://doi.org/10.1186/1477-3155-10-24
- Dhar, S., W. L. Daniel, D. A. Giljohann, C. A. Mirkin, and S. J. Lippard. 2009. “Polyvalent Oligonucleotide Gold Nanoparticle Conjugates as Delivery Vehicles for Platinum(IV) Warheads.” Journal of the American Chemical Society 131 (41): 14652–14653. https://doi.org/10.1021/ja9071282
- Dong, L., S. Tang, F. Deng, Y. Gong, K. Zhao, J. Zhou, D. Liang, et al. 2019. “Shape-Dependent Toxicity of Alumina Nanoparticles in Rat Astrocytes.” The Science of the Total Environment 690: 158–166. https://doi.org/10.1016/j.scitotenv.2019.06.532
- Dong, X., Z. Wu, X. Li, L. Xiao, M. Yang, Y. Li, J. Duan, and Z. Sun. 2020. “The Size-Dependent Cytotoxicity of Amorphous Silica Nanoparticles: A Systematic Review of in Vitro Studies.” International Journal of Nanomedicine 15: 9089–9113. https://doi.org/10.2147/IJN.S276105
- Du Preez, S., A. Johnson, R. F. LeBouf, S. J. L. Linde, A. B. Stefaniak, and J. Du Plessis. 2018. “Exposures during Industrial 3-D Printing and Post-Processing Tasks.” Rapid Prototyping Journal 24 (5): 865–871. https://doi.org/10.1108/RPJ-03-2017-0050
- Dziendzikowska, K., J. Gromadzka-Ostrowska, A. Lankoff, M. Oczkowski, A. Krawczyńska, J. Chwastowska, M. Sadowska-Bratek, et al. 2012. “Time-Dependent Biodistribution and Excretion of Silver Nanoparticles in Male Wistar Rats: Biodistribution of Nanosilver in Rats.” Journal of Applied Toxicology: JAT 32 (11): 920–928. https://doi.org/10.1002/jat.2758
- European Commission (EC). 2003. “Commission Directive 2003/94/EC laying down the principles and guidelines of good manufacturing practice in respect of medicinal products for human use and investigational medicinal products for human use”. Official Journal L 262.
- European Commission (EC). 2009. “Regulation (Ec) No 1223/2009 of the European Parliament and of the Council of 30 November 2009 on cosmetic products”. Official Journal of the European Union L 342/59.
- European Commission (EC). 2011. “Commission Recommendation of 18 October 2011 on the definition of nanomaterial”. Official Journal of the European Union L 275/38.
- European Commission (EC). 2014. “Commission Delegated Regulation No. 1252/2014 of 28 May 2014 supplementing Directive 2001/83/EC of the European Parliament and of the Council with regard to principles and guidelines of good manufacturing practice for active substances for medicinal products for human use”. Official Journal of the European Union L 337/1.
- European Commission (EC). 2022. “Commission Recommendation of 10 June 2022 on the definition of nanomaterial”. Official Journal of the European Union C 229/1.
- European Chemicals Agency (ECHA). 2016. “Guidance on information requirements and chemical safety assessment. Appendix R7-1 Recommendations for nanomaterials applicable to: Chapter R7a Endpoint specific guidance”. Helsinki, Finland: ECHA.
- European Chemicals Agency (ECHA). 2013. “Assessing human health and environmental hazards of nanomaterials. Best practice for REACH Registrants”. Second GAARN meeting. Helsinki, Finland: ECHA.
- European Food Safety Authority (EFSA). 2016. “Scientific Opinion Recent Developments in the risk Assessment of Chemicals in Food and Their Potential Impact on the Safety Assessment of Substances Used in Food Contact Materials”. EFSA Journal 14(1): 4357. https://doi.org/10.2903/j.efsa.2016.4357
- European Food Safety Authority (EFSA). 2011. “Guidance on the risk assessment of the application of nanoscience and nanotechnologies in the food and feed chain”.EFSA Journal 9(5): 2140. https://doi.org/10.2903/j.efsa.2011.2140
- Ermolin, M. S., A. I. Ivaneev, N. N. Fedyunina, and P. S. Fedotov. 2021. “Nanospeciation of Metals and Metalloids in Volcanic Ash Using Single Particle Inductively Coupled Plasma Mass Spectrometry.” Chemosphere 281: 130950. https://doi.org/10.1016/j.chemosphere.2021.130950
- Filipe, V., A. Hawe, and W. Jiskoot. 2010. “Critical Evaluation of Nanoparticle Tracking Analysis (NTA) by NanoSight for the Measurement of Nanoparticles and Protein Aggregates.” Pharmaceutical Research 27 (5): 796–810. https://doi.org/10.1007/s11095-010-0073-2
- Forest, V., L. Leclerc, J.-F. Hochepied, A. Trouvé, G. Sarry, and J. Pourchez. 2017. “Impact of Cerium Oxide Nanoparticles Shape on Their in Vitro Cellular Toxicity.” Toxicology in Vitro: An International Journal Published in Association with BIBRA 38: 136–141. https://doi.org/10.1016/j.tiv.2016.09.022
- Forest, V., and J. Pourchez. 2023. “Human Biological Monitoring of Nanoparticles, a New Way to Investigate Potential Causal Links between Exposure to Nanoparticles and Lung Diseases?” Pulmonology 29 (1): 4–5. https://doi.org/10.1016/j.pulmoe.2022.08.005
- Frazzoli, C., F. Ruggieri, B. Battistini, O. E. Orisakwe, J. K. Igbo, and B. Bocca. 2022. “E-WASTE Threatens Health: The Scientific Solution Adopts the One Health Strategy.” Environmental Research 212 (Pt A): 113227. https://doi.org/10.1016/j.envres.2022.113227
- Frazzoli, C., B. Bocca, and A. Mantovani. 2015. “The One Health Perspective in Trace Elements Biomonitoring.” Journal of Toxicology and Environmental Health. Part B, Critical Reviews 18 (7-8): 344–370. https://doi.org/10.1080/10937404.2015.1085473
- Fubini, B., M. Ghiazza, and I. Fenoglio. 2010. “Physico-Chemical Features of Engineered Nanoparticles Relevant to Their Toxicity.” Nanotoxicology 4 (4): 347–363. https://doi.org/10.3109/17435390.2010.509519
- Fukui, H., M. Horie, S. Endoh, H. Kato, K. Fujita, K. Nishio, L. K. Komaba, et al. 2012. “Association of Zinc Ion Release and Oxidative Stress Induced by Intratracheal Instillation of ZnO Nanoparticles to Rat Lung.” Chemico-Biological Interactions 198 (1-3): 29–37. https://doi.org/10.1016/j.cbi.2012.04.007
- Galea, K. S., S. P. Porras, S. Viegas, B. Bocca, R. Bousoumah, R. C. Duca, L. Godderis, et al. 2021. “HBM4EU Chromates study - Reflection and Lessons Learnt from Designing and Undertaking a Collaborative European Biomonitoring Study on Occupational Exposure to Hexavalent Chromium.” International Journal of Hygiene and Environmental Health 248: 114099. https://doi.org/10.1016/j.ijheh.2022.114099
- Gao, X., and G. V. Lowry. 2018. “Progress towards Standardized and Validated Characterizations for Measuring Physicochemical Properties of Manufactured Nanomaterials Relevant to Nano Health and Safety Risks.” NanoImpact 9: 14–30. https://doi.org/10.1016/j.impact.2017.09.002
- Gautam, A., D. Singh, and R. Vijayaraghavan. 2011. “Dermal Exposure of Nanoparticles: An Understanding.” Journal of Cell and Tissue Research 11: 2703–2708.
- Geiss, O., I. Bianchi, G. Bucher, E. Verleysen, F. Brassinne, J. Mast, K. Loeschner, et al. 2022. “Determination of the Transport Efficiency in spICP-MS Analysis Using Conventional Sample Introduction Systems: An Interlaboratory Comparison Study.” Nanomaterials 12 (4): 725. https://doi.org/10.3390/nano12040725
- Ghomrasni, N. B., C. Chivas-Joly, L. Devoille, J.-F. Hochepied, and N. Feltin. 2020. “Challenges in Sample Preparation for Measuring Nanoparticles Size by Scanning Electron Microscopy from Suspensions, Powder Form and Complex Media.” Powder Technology 359: 226–237. https://doi.org/10.1016/j.powtec.2019.10.022
- Gigault, J., J. M. Pettibone, C. Schmitt, and V. A. Hackley. 2014. “Rational Strategy for Characterization of Nanoscale Particles by Asymmetric-Flow Field Flow Fractionation: A Tutorial.” Analytica Chimica Acta 809: 9–24. https://doi.org/10.1016/j.aca.2013.11.021
- Graczyk, H., N. Lewinski, J. Zhao, J.-J. Sauvain, G. Suarez, P. Wild, B. Danuser, and M. Riediker. 2015. “Increase in Oxidative Stress Levels following Welding Fume Inhalation: A Controlled Human Exposure Study.” Particle and Fibre Toxicology 13 (1): 31. https://doi.org/10.1186/s12989-016-0143-7
- Graf, C., D. Nordmeyer, C. Sengstock, S. Ahlberg, J. Diendorf, J. Raabe, M. Epple, et al. 2018. “Shape-Dependent Dissolution and Cellular Uptake of Silver Nanoparticles.” Langmuir: The ACS Journal of Surfaces and Colloids 34 (4): 1506–1519. https://doi.org/10.1021/acs.langmuir.7b03126
- Gray, E. P., J. G. Coleman, A. J. Bednar, A. J. Kennedy, J. F. Ranville, and C. P. Higgins. 2013. “Extraction and Analysis of Silver and Gold Nanoparticles from Biological Tissues Using Single Particle Inductively Coupled Plasma Mass Spectrometry.” Environmental Science & Technology 47 (24): 14315–14323. https://doi.org/10.1021/es403558c
- Gulson, B., M. McCall, M. Korsch, L. Gomez, P. Casey, Y. Oytam, A. Taylor, et al. 2010. “Small Amounts of Zinc from Zinc Oxide Particles in Sunscreens Applied Outdoors Are Absorbed through Human Skin.” Toxicological Sciences: An Official Journal of the Society of Toxicology 118 (1): 140–149. https://doi.org/10.1093/toxsci/kfq243
- Gulumian, M., J. Verbeek, C. Andraos, N. Sanabria, and P. de Jager. 2016. “Systematic Review of Screening and Surveillance Programs to Protect Workers from Nanomaterials.” PloS One 11 (11): e0166071. https://doi.org/10.1371/journal.pone.0166071
- Hadrup, N., K. Aimonen, M. Ilves, H. Lindberg, R. Atluri, N. M. Sahlgren, N. R. Jacobsen, et al. 2021. “Pulmonary Toxicity of Synthetic Amorphous Silica - Effects of Porosity and Copper Oxide Doping.” Nanotoxicology 15 (1): 96–113. https://doi.org/10.1080/17435390.2020.1842932
- Hartmann, N. B., Jensen, K. A., Baun, A., Rasmussen, K., Rauscher, H., Tantra, R., Cupi, D., Gilliland, D., Pianella, F., and Riego Sintes J. M. 2015. “Techniques and Protocols for Dispersing Nanoparticle Powders in Aqueous Media—is There a Rationale for Harmonization?” Journal of Toxicology and Environmental Health. Part B, Critical Reviews 18 (6): 299–326. https://doi.org/10.1080/10937404.2015.1074969
- Hemmendinger, M., G. Squillacioti, T. Charreau, G. Garzaro, F. Ghelli, R. Bono, J. J. Sauvain, et al. 2023. “Occupational Exposure to Nanomaterials and Biomarkers in Exhaled Air and Urine: Insights from the NanoExplore International Cohort.” Environment International 179: 108157. https://doi.org/10.1016/j.envint.2023.108157
- Hemmendinger, M., P. Wild, Y. Shoman, M. Graille, E. Bergamaschi, N. Hopf, and I. Guseva Canu. 2020. “Reference Ranges of Oxidative Stress Biomarkers Selected for Non-Invasive Biological Surveillance of Nanotechnology Workers: Study Protocol and Meta-Analysis Results for 8-OHdG in Exhaled Breath Condensate.” Toxicology Letters 327: 41–47. https://doi.org/10.1016/j.toxlet.2020.03.021
- Heringa, M. B., R. J. B. Peters, R. L. A. W. Bleys, M. K. van der Lee, P. C. Tromp, P. C. E. van Kesteren, J. C. H. van Eijkeren, A. K. Undas, A. G. Oomen, and H. Bouwmeester. 2018. “Detection of Titanium Particles in Human Liver and Spleen and Possible Health Implications.” Particle and Fibre Toxicology 15 (1): 15. https://doi.org/10.1186/s12989-018-0251-7
- Heroult, J., V. Nischwitz, D. Bartczak, and H. Goenaga-Infante. 2014. “The Potential of Asymmetric Flow Field-Flow Fractionation Hyphenated to Multiple Detectors for the Quantification and Size Estimation of Silica Nanoparticles in a Food Matrix.” Analytical and Bioanalytical Chemistry 406 (16): 3919–3927. https://doi.org/10.1007/s00216-014-7831-7
- Hole, P., K. Sillence, C. Hannell, C. M. Maguire, M. Roesslein, G. Suarez, S. Capracotta, et al. 2013. “Interlaboratory Comparison of Size Measurements on Nanoparticles Using Nanoparticle Tracking Analysis (NTA).” Journal of Nanoparticle Research 15 (12): 2101. https://doi.org/10.1007/s11051-013-2101-8
- Hsiao, I.-L., and Y.-J. Huang. 2011. “Effects of Various Physicochemical Characteristics on the Toxicities of ZnO and TiO2 Nanoparticles toward Human Lung Epithelial Cells.” The Science of the Total Environment 409 (7): 1219–1228. https://doi.org/10.1016/j.scitotenv.2010.12.033
- Huang, Y., J. Tsz-Shan Lum, and K. Sze-Yin Leung. 2020. “Single Particle ICP-MS Combined with Internal Standardization for Accurate Characterization of Polydisperse Nanoparticles in Complex Matrices.” Journal of Analytical Atomic Spectrometry 35 (10): 2148–2155. https://doi.org/10.1039/D0JA00180E
- Iavicoli, I., V. Leso, L. Fontana, and E. Calabrese. 2018. “Nanoparticle Exposure and Hormetic Dose–Responses: An Update.” International Journal of Molecular Sciences 19 (3): 805. https://doi.org/10.3390/ijms19030805
- Iavicoli, I., V. Leso, and P. A. Schulte. 2016. “Biomarkers of Susceptibility: State of the Art and Implications for Occupational Exposure to Engineered Nanomaterials.” Toxicology and Applied Pharmacology 299: 112–124. https://doi.org/10.1016/j.taap.2015.12.018
- Iavicoli, I., V. Leso, M. Manno, and P. A. Schulte. 2014. “Biomarkers of Nanomaterial Exposure and Effect: current Status.” Journal of Nanoparticle Research 16 (3): 2302. https://doi.org/10.1007/s11051-014-2302-9
- International Commission on Radiological Protection (ICRP). 1994. “Human Respiratory Tract Model for Radiological Protection”. International Commission on Radiological Protection Publication 66, Tarrytown, NY.
- Ishizaka, T., K. Nagano, I. Tasaki, H. Tao, J.-Q. Gao, K. Harada, K. Hirata, et al. 2019. “Optimization and Evaluation of Pretreatment Method for sp-ICP-MS to Reveal the Distribution of Silver Nanoparticles in the Body.” Nanoscale Research Letters 14 (1): 180. https://doi.org/10.1186/s11671-019-3016-9
- International Organization for Standardization (ISO). 2007. “Particulate materials - Sampling and sample splitting for the determination of particulate properties”. ISO 14488:2007.
- International Organization for Standardization (ISO). 2018a. Nanotechnologies - Analysis of nano-objects using asymmetrical-flow and centrifugal field-flow fractionation. ISO/TS 21362:2018.
- International Organization for Standardization (ISO). 2018b. “General requirements for the competence of testing and calibration laboratories”. UNI CEI EN ISO/IEC 17025:2018.
- Jemec, A., A. Kahru, A. Potthoff, D. Drobne, M. Heinlaan, S. Böhme, M. Geppert, et al. 2016. “An Interlaboratory Comparison of Nanosilver Characterisation and Hazard Identification: Harmonising Techniques for High Quality Data.” Environment International 87: 20–32. https://doi.org/10.1016/j.envint.2015.10.014
- Jones, K. 2020. “Human Biomonitoring in Occupational Health for Exposure Assessment.” Portuguese Journal of Public Health 38 (1): 2–5. https://doi.org/10.1159/000509480
- Kaminski, H., M. Beyer, H. Fissan, C. Asbach, and T. A. Kuhlbusch. 2015. “Measurements of Nanoscale TiO2 and Al2O3 in Industrial Workplace Environments - Methodology and Results.” Aerosol and Air Quality Research 15 (1): 129–141. https://doi.org/10.4209/aaqr.2014.03.0065
- Kang, B., M. A. Mackey, and M. A. El-Sayed. 2010. “Nuclear Targeting of Gold Nanoparticles in Cancer Cells Induces DNA Damage, Causing Cytokinesis Arrest and Apoptosis.” Journal of the American Chemical Society 132 (5): 1517–1519. https://doi.org/10.1021/ja9102698
- Kestens, V., G. Roebben, J. Herrmann, Å. Jämting, V. Coleman, C. Minelli, C. Clifford, et al. 2016. “Challenges in the Size Analysis of a Silica Nanoparticle Mixture as Candidate Certified Reference Material.” Journal of Nanoparticle Research 18 (6): 171. https://doi.org/10.1007/s11051-016-3474-2
- Kim, S.-H., J. H. Lee, K. Jung, J.-Y. Yang, H.-S. Shin, J. P. Lee, J. Jeong, J.-H. Oh, and J. K. Lee. 2021. “Copper and Cobalt Ions Released from Metal Oxide Nanoparticles Trigger Skin Sensitization.” Frontiers in Pharmacology 12: 627781. https://doi.org/10.3389/fphar.2021.627781
- Kratochvíl, P., and M. Netopilík. 2017. “The Effect of Nanoparticle Nonuniformity on the Ratio of Gyration and Hydrodynamic Radiuses.” International Journal of Polymer Analysis and Characterization 22 (2): 112–117. https://doi.org/10.1080/1023666X.2016.1252872
- Krug, H. F. 2014. “Nanosafety Research-Are We on the Right Track?” Angewandte Chemie (International ed. in English) 53 (46): 12304–12319. https://doi.org/10.1002/anie.201403367
- Kuhlbusch, T. A. J., S. W. P. Wijnhoven, and A. Haase. 2018. “Nanomaterial Exposures for Worker, Consumer and the General Public.” NanoImpact 10: 11–25. https://doi.org/10.1016/j.impact.2017.11.003
- Kurjane, N., T. Zvagule, J. Reste, Z. Martinsone, I. Pavlovska, I. Martinsone, and I. Vanadzins. 2017. “The Effect of Different Workplace Nanoparticles on the Immune Systems of Employee.” Journal of Nanoparticle Research 19 (9): 320. https://doi.org/10.1007/s11051-017-4004-6
- Laux, P., J. Tentschert, C. Riebeling, A. Braeuning, O. Creutzenberg, A. Epp, V. Fessard, et al. 2018. “Nanomaterials: certain Aspects of Application, Risk Assessment and Risk Communication.” Archives of Toxicology 92 (1): 121–141. https://doi.org/10.1007/s00204-017-2144-1
- Laycock, A., N. J. Clark, R. Clough, R. Smith, and R. D. Handy. 2022. “Determination of Metallic Nanoparticles in Biological Samples by Single Particle ICP-MS: A Systematic Review from Sample Collection to Analysis.” Environmental Science Nano 9 (2): 420–453. https://doi.org/10.1039/D1EN00680K
- Lebedová, J., Y. S. Hedberg, I. Odnevall Wallinder, and H. L. Karlsson. 2018. “Size-Dependent Genotoxicity of Silver, Gold and Platinum Nanoparticles Studied Using the Mini-Gel Comet Assay and Micronucleus Scoring with Flow Cytometry.” Mutagenesis 33 (1): 77–85. https://doi.org/10.1093/mutage/gex027
- Lee, J. S., Y. C. Choi, J. H. Shin, J. H. Lee, Y. Lee, S. Y. Park, J. E. Baek, J. D. Park, K. Ahn, and I. J. Yu. 2015. “Health Surveillance Study of Workers Who Manufacture Multi-Walled Carbon Nanotubes.” Nanotoxicology 9 (6): 802–811. https://doi.org/10.3109/17435390.2014.978404
- Lee, J. H., J. E. Ju, B. I. Kim, P. J. Pak, E.-K. Choi, H.-S. Lee, and N. Chung. 2014. “Rod-Shaped Iron Oxide Nanoparticles Are More Toxic than Sphere-Shaped Nanoparticles to Murine Macrophage Cells: Toxicity of Rod and Sphere Iron Oxide Nanoparticles.” Environmental Toxicology and Chemistry 33 (12): 2759–2766. https://doi.org/10.1002/etc.2735
- Lee, O., S. H. Jeong, W. U. Shin, G. Lee, C. Oh, and S. W. Son. 2013. “Influence of Surface Charge of Gold Nanorods on Skin Penetration.” Skin Research and Technology 19 (1): e390–e396. https://doi.org/10.1111/j.1600-0846.2012.00656.x
- Lee, J. H., J. Mun, J. D. Park, and I. J. Yu. 2012. “A Health Surveillance Case Study on Workers Who Manufacture Silver Nanomaterials.” Nanotoxicology 6 (6): 667–669. https://doi.org/10.3109/17435390.2011.600840
- Leese, E., K. Jones, B. Bocca, R. Bousoumah, A. Castaño, K. S. Galea, I. Iavicoli, et al. 2023. “HBM4EU Chromates Study - the Measurement of Hexavalent and Trivalent Chromium in Exhaled Breath Condensate Samples from Occupationally Exposed Workers across Europe.” Toxicology Letters 15375: 59–68. https://doi.org/10.1016/j.toxlet.2022.12.009
- Liati, A., S. S. Pandurangi, K. Boulouchos, D. Schreiber, and Y. Arroyo Rojas Dasilva. 2015. “Metal Nanoparticles in Diesel Exhaust Derived by in-Cylinder Melting of Detached Engine Fragments.” Atmospheric Environment 101: 34–40. https://doi.org/10.1016/j.atmosenv.2014.11.014
- Liao, H.-Y., Y.-T. Chung, C.-H. Lai, S.-L. Wang, H.-C. Chiang, L.-A. Li, T.-C. Tsou, et al. 2014. “Six-Month Follow-up Study of Health Markers of Nanomaterials among Workers Handling Engineered Nanomaterials.” Nanotoxicology 8 (Suppl 1): 100–110. https://doi.org/10.3109/17435390.2013.858793
- Liou, S.-H., W.-T. Wu, H.-Y. Liao, C.-Y. Chen, C.-Y. Tsai, W.-T. Jung, and H.-L. Lee. 2017. “Global DNA Methylation and Oxidative Stress Biomarkers in Workers Exposed to Metal Oxide Nanoparticles.” Journal of Hazardous Materials 331: 329–335. https://doi.org/10.1016/j.jhazmat.2017.02.042
- Liou, S.-H., Y.-C. Chen, H.-Y. Liao, C.-J. Wang, J.-S. Chen, and H.-L. Lee. 2016. “Increased Levels of Oxidative Stress Biomarkers in Metal Oxides Nanomaterial-Handling Workers.” Biomarkers 21 (7): 600–606. https://doi.org/10.3109/1354750X.2016.1160432
- Liou, S.-H., T.-C. Tsou, S.-L. Wang, L.-A. Li, H.-C. Chiang, W.-F. Li, P.-P. Lin, et al. 2012. “Epidemiological Study of Health Hazards among Workers Handling Engineered Nanomaterials.” Journal of Nanoparticle Research 14 (8): 878. https://doi.org/10.1007/s11051-012-0878-5
- Lischkova, L., D. Pelclova, J. Hlusicka, T. Navratil, S. Vlckova, Z. Fenclova, S. Dvorackova, et al. 2019. “Detection and Identification of Engineered Nanoparticles in Exhaled Breath Condensate, Blood Serum, and Urine of Occupationally Exposed Subjects.” Monatshefte Für Chemie - Chemical Monthly 150 (3): 511–523. https://doi.org/10.1007/s00706-019-2379-z
- Liu, J., K. E. Murphy, M. R. Winchester, and V. A. Hackley. 2017. “Overcoming Challenges in Single Particle Inductively Coupled Plasma Mass Spectrometry Measurement of Silver Nanoparticles.” Analytical and Bioanalytical Chemistry 409 (25): 6027–6039. https://doi.org/10.1007/s00216-017-0530-4
- Liu, H.-H., C.-Y. Chen, G.-I. Chen, L.-H. Lee, and H.-L. Chen. 2012. “Relationship between Indium Exposure and Oxidative Damage in Workers in Indium Tin Oxide Production Plants.” International Archives of Occupational and Environmental Health 85 (4): 447–453. https://doi.org/10.1007/s00420-011-0688-6
- Locci, E., I. Pilia, R. Piras, S. Pili, G. Marcias, P. Cocco, F. De Giorgio, et al. 2019. “Particle Background Levels in Human Tissues-PABALIHT Project. Part I: A Nanometallomic Study of Metal-Based Micro- and Nanoparticles in Liver and Kidney in an Italian Population Group.” Journal of Nanoparticle Research 21 (3): 45. https://doi.org/10.1007/s11051-019-4480-y
- Loeschner, K., J. Navratilova, C. Købler, K. Mølhave, S. Wagner, F. von der Kammer, and E. H. Larsen. 2013. “Detection and Characterization of Silver Nanoparticles in Chicken Meat by Asymmetric Flow Field Flow Fractionation with Detection by Conventional or Single Particle ICP-MS.” Analytical and Bioanalytical Chemistry 405 (25): 8185–8195. https://doi.org/10.1007/s00216-013-7228-z
- Longmire, M., P. L. Choyke, and H. Kobayashi. 2008. “Clearance Properties of Nano-Sized Particles and Molecules as Imaging Agents: Considerations and Caveats.” Nanomedicine 3 (5): 703–717. https://doi.org/10.2217/17435889.3.5.703
- Luo, X., D. Xie, J. Hu, J. Su, and Z. Xue. 2022. “Oxidative Stress and Inflammatory Biomarkers for Populations with Occupational Exposure to Nanomaterials: A Systematic Review and Meta-Analysis.” Antioxidants 11 (11): 2182. https://doi.org/10.3390/antiox11112182
- Mandzy, N., E. Grulke, and T. Druffel. 2005. “Breakage of TiO2 Agglomerates in Electrostatically Stabilized Aqueous Dispersions.” Powder Technology 160 (2): 121–126. https://doi.org/10.1016/j.powtec.2005.08.020
- Manno, M., F. Sito, and L. Licciardi. 2014. “Ethics in Biomonitoring for Occupational Health.” Toxicology Letters 231 (2): 111–121. https://doi.org/10.1016/j.toxlet.2014.10.004
- Marie-Desvergne, C., M. Dubosson, L. Touri, E. Zimmermann, M. Gaude-Môme, L. Leclerc, C. Durand, et al. 2016. “Assessment of Nanoparticles and Metal Exposure of Airport Workers Using Exhaled Breath Condensate.” Journal of Breath Research 10 (3): 036006. https://doi.org/10.1088/1752-7155/10/3/036006
- Mast, J., E. Verleysen, and P.-J. De Temmerman. 2015. “Physical Characterization of Nanomaterials in Dispersion by Transmission Electron Microscopy in a Regulatory Framework.” In: Deepak, F., Mayoral, A., Arenal, R. (eds) Advanced Transmission Electron Microscopy (pp. 249–270). Cham: Springer. https://doi.org/10.1007/978-3-319-15177-9_8
- Menon, D., and S. Chakraborty. 2023. “How Safe Are Nanoscale Metal-Organic Frameworks?” Frontiers in Toxicology 5: 1233854. https://doi.org/10.3389/ftox.2023.1233854
- Methner, M. M. 2010. “Effectiveness of a Custom-Fitted Flange and Local Exhaust Ventilation (LEV) System in Controlling the Release of Nanoscale Metal Oxide Particulates during Reactor Cleanout Operations.” International Journal of Occupational and Environmental Health 16 (4): 475–487. https://doi.org/10.1179/oeh.2010.16.4.475
- Miller, M. R., J. B. Raftis, J. P. Langrish, S. G. McLean, P. Samutrtai, S. P. Connell, S. Wilson, et al. 2017. “Inhaled Nanoparticles Accumulate at Sites of Vascular Disease.” ACS Nano 11 (5): 4542–4552. https://doi.org/10.1021/acsnano.6b08551
- Mitrano, D. M., A. Barber, A. Bednar, P. Westerhoff, C. P. Higgins, and J. F. Ranville. 2012. “Silver Nanoparticle Characterization Using Single Particle ICP-MS (SP-ICP-MS) and Asymmetrical Flow Field Flow Fractionation ICP-MS (AF4-ICP-MS).” Journal of Analytical Atomic Spectrometry 27 (7): 1131. https://doi.org/10.1039/c2ja30021d
- Moore, J., and E. Cerasoli. 2017. “Particle Light Scattering Methods and Applications". In: Lindon, J C., Tranter, G. E., Koppenaal D. W. (eds) Encyclopedia of Spectroscopy and Spectrometry (Third Edition): 543–553. Academic Press. https://doi.org/10.1016/B978-0-12-803224-4.00040-6
- Naess, E. M., A. Hofgaard, V. Skaug, M. Gulbrandsen, T. E. Danielsen, S. Grahnstedt, A. Skogstad, and J.-Ø. Holm. 2016. “Titanium Dioxide Nanoparticles in Sunscreen Penetrate the Skin into Viable Layers of the Epidermis: A Clinical Approach.” Photodermatology, Photoimmunology & Photomedicine 32 (1): 48–51. https://doi.org/10.1111/phpp.12217
- Ndaw, S., V. Leso, R. Bousoumah, A. Rémy, B. Bocca, R. C. Duca, L. Godderis, I., et al. 2022. “HBM4EU Chromates study – Usefulness of Measurement of Blood Chromium Levels in the Assessment of Occupational Cr(VI) Exposure.” Environmental Research 214 (Pt 1): 113758. https://doi.org/10.1016/j.envres.2022.113758
- Neubauer, K., and S. Chady. 2014. “Determination of Gold and Silver Nanoparticles in. Blood Using Single Particle ICP-MS.” Perkin Elmer Application
- National Institute for Occupational Safety & Health (NIOSH). 2011. Occupational Exposure to Titanium Dioxide. Washington DC: Centers for Disease Control and Prevention.
- Organisation for Economic Co-operation and Development (OECD). 2022. “Important Issues on Risk Assessment of Manufactured Nanomaterials”. Series on the Safety of Manufactured Nanomaterials No. 103. ENV/CBC/MONO(2022).
- Organisation for Economic Co-operation and Development (OECD). 2012. Guidance on Sample Preparation and Dosimetry for the Safety Testing of Manufactured Nanomaterials”. Series on the Safety of Manufactured Nanomaterials No. 36. ENV/JM/MONO(2012)40.
- OECD (Organisation for Economic Co-operation and Development). 1998. “OECD series on principles of good laboratory practice and compliance monitoring Number 11. Advisory document of the panel on good laboratory practice. The role and responsibilities of the sponsor in the application of the principles of GLP”. ENV/MC/CHEM(9816.
- Okuda-Shimazaki, J., S. Takaku, K. Kanehira, S. Sonezaki, and A. Taniguchi. 2010. “Effects of Titanium Dioxide Nanoparticle Aggregate Size on Gene Expression.” International Journal of Molecular Sciences 11 (6): 2383–2392. https://doi.org/10.3390/ijms11062383
- Pelclova, D., V. Zdimal, M. Komarc, J. Schwarz, J. Ondracek, L. Ondrackova, M. Kostejn, et al. 2020. “Three-Year Study of Markers of Oxidative Stress in Exhaled Breath Condensate in Workers Producing Nanocomposites, Extended by Plasma and Urine Analysis in Last Two Years.” Nanomaterials 10 (12): 2440. https://doi.org/10.3390/nano10122440
- Pelclova, D., T. Navratil, T. Kacerova, B. Zamostna, Z. Fenclova, S. Vlckova, and P. Kacer. 2019. “NanoTiO2 Sunscreen Does Not Prevent Systemic Oxidative Stress Caused by UV Radiation and a Minor Amount of NanoTiO2 is Absorbed in Humans.” Nanomaterials 9 (6): 888. https://doi.org/10.3390/nano9060888
- Pelclova, D., V. Zdimal, J. Schwarz, S. Dvorackova, M. Komarc, J. Ondracek, M. Kostejn, et al. 2018. “Markers of Oxidative Stress in the Exhaled Breath Condensate of Workers Handling Nanocomposites.” Nanomaterials 8 (8): 611. https://doi.org/10.3390/nano8080611
- Pelclova, D., V. Zdimal, P. Kacer, N. Zikova, M. Komarc, Z. Fenclova, S. Vlckova, et al. 2017. “Markers of Lipid Oxidative Damage in the Exhaled Breath Condensate of Nano TiO2 Production Workers.” Nanotoxicology 11 (1): 52–63. https://doi.org/10.1080/17435390.2016.1262921
- Pelclova, D., V. Zdimal, Z. Fenclova, S. Vlckova, F. Turci, I. Corazzari, P. Kacer, et al. 2016a. “Markers of Oxidative Damage of Nucleic Acids and Proteins among Workers Exposed to TiO2 (Nano) Particles.” Occupational and Environmental Medicine 73 (2): 110–118. https://doi.org/10.1136/oemed-2015-103161
- Pelclova, D., V. Zdimal, P. Kacer, Z. Fenclova, S. Vlckova, M. Komarc, T. Navratil, et al. 2016b. “Leukotrienes in Exhaled Breath Condensate and Fractional Exhaled Nitric Oxide in Workers Exposed to TiO2 Nanoparticles.” Journal of Breath Research 10 (3): 036004. https://doi.org/10.1088/1752-7155/10/3/036004
- Pelclova, D., H. Barosova, J. Kukutschova, V. Zdimal, T. Navratil, Z. Fenclova, S. Vlckova, et al. 2015. “Raman Microspectroscopy of Exhaled Breath Condensate and Urine in Workers Exposed to Fine and Nano TiO2 Particles: A Cross-Sectional Study.” Journal of Breath Research 9 (3): 036008. https://doi.org/10.1088/1752-7155/9/3/036008
- Pelclova, D., V. Zdimal, Z. Fenclova, V. Stepanka, J. Schwarz, J. Pusman, N. Zikova, et al. 2012. “Markers of Oxidative Stress Are Elevated in Workers Exposed to Nanoparticles.” Conference Proceedings, NANOCON 2012, 658. https://doi.org/10.13140/2.1.4208.1282
- Pele, L. C., V. Thoree, S. F. A. Bruggraber, D. Koller, R. P. H. Thompson, M. C. Lomer, and J. J. Powell. 2015. “Pharmaceutical/Food Grade Titanium Dioxide Particles Are Absorbed into the Bloodstream of Human Volunteers.” Particle and Fibre Toxicology 12 (1): 26. https://doi.org/10.1186/s12989-015-0101-9
- Peters, R. J. B., A. G. Oomen, G. van Bemmel, L. van Vliet, A. K. Undas, S. Munniks, R. L. A. W. Bleys, P. C. Tromp, W. Brand, and M. van der Lee. 2020. “Silicon Dioxide and Titanium Dioxide Particles Found in Human Tissues.” Nanotoxicology 14 (3): 420–432. https://doi.org/10.1080/17435390.2020.1718232
- Phillips, J. I., F. Y. Green, J. C. A. Davies, and J. Murray. 2010. “Pulmonary and Systemic Toxicity following Exposure to Nickel Nanoparticles.” American Journal of Industrial Medicine 53 (8): 763–767. https://doi.org/10.1002/ajim.20855
- Pietroiusti, A., and A. Magrini. 2014. “Engineered Nanoparticles at the Workplace: Current Knowledge about Workers’ Risk.” Occupational Medicine 64 (5): 319–330. https://doi.org/10.1093/occmed/kqu051
- Pirela, S. V., G. A. Sotiriou, D. Bello, M. Shafer, K. L. Bunker, V. Castranova, T. Thomas, and P. Demokritou. 2015. “Consumer Exposures to Laser Printer-Emitted Engineered Nanoparticles: A Case Study of Life-Cycle Implications from Nano-Enabled Products.” Nanotoxicology 9 (6): 760–768. https://doi.org/10.3109/17435390.2014.976602
- Pitkänen, L., and A. M. Striegel. 2016. “Size-Exclusion Chromatography of Metal Nanoparticles and Quantum Dots.” Trends in Analytical Chemistry 80: 311–320. https://doi.org/10.1016/j.trac.2015.06.013
- Poland, C. A., Read, S. A. K., Varet, J., Carse, G.,Christensen, F. M., and Hankin, S. M. 2013. “Dermal Absorption of Nanomaterials.” Copenhagen, Denmark: The Danish Environmental Protection Agency, 1–167. ISBN No. 978-87-93026-50-6
- Pradhan, S., J. Hedberg, E. Blomberg, S. Wold, and I. Odnevall Wallinder. 2016. “Effect of Sonication on Particle Dispersion, Administered Dose and Metal Release of Non-Functionalized, Non-Inert Metal Nanoparticles.” Journal of Nanoparticle Research 18 (9): 285. https://doi.org/10.1007/s11051-016-3597-5
- Rao, A., M. Schoenenberger, E. Gnecco, T. Glatzel, E. Meyer, D. Brändlin, and L. Scandella. 2007. “Characterization of Nanoparticles Using Atomic Force Microscopy.” Journal of Physics: Conference Series 61: 971–976. https://doi.org/10.1088/1742-6596/61/1/192
- Rasmussen, K., H. Rauscher, P. Kearns, M. González, and J. Riego Sintes. 2019. “Developing OECD Test Guidelines for Regulatory Testing of Nanomaterials to Ensure Mutual Acceptance of Test Data.” Regulatory Toxicology and Pharmacology 104: 74–83. https://doi.org/10.1016/j.yrtph.2019.02.008
- Riccelli, M. G., M. Goldoni, R. Andreoli, P. Mozzoni, S. Pinelli, R. Alinovi, L. Selis, A. Mutti, and M. Corradi. 2018. “Biomarkers of Exposure to Stainless Steel Tungsten Inert Gas Welding Fumes and the Effect of Exposure on Exhaled Breath Condensate.” Toxicology Letters 292: 108–114. https://doi.org/10.1016/j.toxlet.2018.04.032
- Roco, M. C. 2011. “The Long View of Nanotechnology Development: The National Nanotechnology Initiative at 10 Years.” Journal of Nanoparticle Research 13 (2): 427–445. https://doi.org/10.1007/s11051-010-0192-z
- Rodríguez-Ibarra, C., A. Déciga-Alcaraz, O. Ispanixtlahuatl-Meráz, E. I. Medina-Reyes, N. L. Delgado-Buenrostro, and Y. I. Chirino. 2020. “International Landscape of Limits and Recommendations for Occupational Exposure to Engineered Nanomaterials.” Toxicology Letters 322: 111–119. https://doi.org/10.1016/j.toxlet.2020.01.016
- Roman, M., C. Rigo, H. Castillo-Michel, I. Munivrana, V. Vindigni, I. Mičetić, F. Benetti, L. Manodori, and W. R. L. Cairns. 2016. “Hydrodynamic Chromatography Coupled to Single-Particle ICP-MS for the Simultaneous Characterization of AgNPs and Determination of Dissolved Ag in Plasma and Blood of Burn Patients.” Analytical and Bioanalytical Chemistry 408 (19): 5109–5124. https://doi.org/10.1007/s00216-015-9014-6
- Rossnerova, A., K. Honkova, D. Pelclova, V. Zdimal, J. A. Hubacek, I. Chvojkova, K. Vrbova, et al. 2020. “DNA Methylation Profiles in a Group of Workers Occupationally Exposed to Nanoparticles.” International Journal of Molecular Sciences 21 (7): 2420. https://doi.org/10.3390/ijms21072420
- Saleem, H., and S. Zaidi. 2020. “Sustainable Use of Nanomaterials in Textiles and Their Environmental Impact.” Materials 13 (22): 5134. https://doi.org/10.3390/ma13225134
- Salou, S., C.-M. Cirtiu, D. Larivière, and N. Fleury. 2020. “Assessment of Strategies for the Formation of Stable Suspensions of Titanium Dioxide Nanoparticles in Aqueous Media Suitable for the Analysis of Biological Fluids.” Analytical and Bioanalytical Chemistry 412 (7): 1469–1481. https://doi.org/10.1007/s00216-020-02412-2
- Santonen, T., B. Bocca, R. Bousoumah, R. C. Duca, K. S. Galea, L. Godderis, T. Göen, J., et al. 2022. “HBM4EU Chromates Study – Overall Results and Recommendations for the Biomonitoring of Occupational Exposure to Hexavalent Chromium.” Environmental Research 204 (Pt A): 111984. https://doi.org/10.1016/j.envres.2021.111984
- Santonen, T., H. Louro, B. Bocca, R. Bousoumah, R. C. Duca, A. Fucic, K. S. Galea, et al. 2023. “The HBM4EU Chromates Study – Outcomes and Impacts on EU Policies and Occupational Health Practices.” International Journal of Hygiene and Environmental Health 248: 114099. https://doi.org/10.1016/j.ijheh.2022.114099
- Scientific Committee on Emerging and Newly Identified Health Risks (SCENIHR). 2006. “SCENIHR modified Opinion (after public consultation) on the appropriateness of existing methodologies to assess the potential risks associated with engineered and adventitious products of nanotechnologies”.
- Schaeublin, N. M., L. K. Braydich-Stolle, A. M. Schrand, J. M. Miller, J. Hutchison, J. J. Schlager, and S. M. Hussain. 2011. “Surface Charge of Gold Nanoparticles Mediates Mechanism of Toxicity.” Nanoscale 3 (2): 410–420. https://doi.org/10.1039/c0nr00478b
- Schaller, K. H., J. Angerer, and H. Drexler. 2002. “Quality Assurance of Biological Monitoring in Occupational and Environmental Medicine.” Journal of Chromatography B 778 (1-2): 403–417. https://doi.org/10.1016/S1570-0232(02)00171-X
- Schleh, C., M. Semmler-Behnke, J. Lipka, A. Wenk, S. Hirn, M. Schäffler, G. Schmid, U. Simon, and W. G. Kreyling. 2012. “Size and Surface Charge of Gold Nanoparticles Determine Absorption across Intestinal Barriers and Accumulation in Secondary Target Organs after Oral Administration.” Nanotoxicology 6 (1): 36–46. https://doi.org/10.3109/17435390.2011.552811
- Schmidt, B., K. Loeschner, N. Hadrup, A. Mortensen, J. J. Sloth, C. Bender Koch, and E. H. Larsen. 2011. “Quantitative Characterization of Gold Nanoparticles by Field-Flow Fractionation Coupled Online with Light Scattering Detection and Inductively Coupled Plasma Mass Spectrometry.” Analytical Chemistry 83 (7): 2461–2468. https://doi.org/10.1021/ac102545e
- Schulte, P., V. Leso, M. Niang, and I. Iavicoli. 2018a. “Biological Monitoring of Workers Exposed to Engineered Nanomaterials.” Toxicology Letters 298: 112–124. https://doi.org/10.1016/j.toxlet.2018.06.003
- Sharma, V., A. Kumar, and A. Dhawan. 2012. “Nanomaterials: Exposure, Effects and Toxicity Assessment.” Proceedings of the National Academy of Sciences B 82 (S1): 3–11. https://doi.org/10.1007/s40011-012-0072-7
- Shaw, B. J., C. S. Ramsden, A. Turner, and R. D. Handy. 2013. “A Simplified Method for Determining Titanium from TiO2 Nanoparticles in Fish Tissue with a Concomitant Multi-Element Analysis.” Chemosphere 92 (9): 1136–1144. https://doi.org/10.1016/j.chemosphere.2013.01.065
- Shukla, S., A. Jadaun, V. Arora, R. K. Sinha, N. Biyani, and V. K. Jain. 2015. “In Vitro Toxicity Assessment of Chitosan Oligosaccharide Coated Iron Oxide Nanoparticles.” Toxicology Reports 2: 27–39. https://doi.org/10.1016/j.toxrep.2014.11.002
- Song, Y., X. Li, L. Wang, Y. Rojanasakul, V. Castranova, H. Li, and J. Ma. 2011. “Nanomaterials in Humans: Identification, Characteristics, and Potential Damage.” Toxicologic Pathology 39 (5): 841–849. https://doi.org/10.1177/0192623311413787
- Steckiewicz, K. P., E. Barcinska, A. Malankowska, A. Zauszkiewicz–Pawlak, G. Nowaczyk, A. Zaleska-Medynska, and I. Inkielewicz-Stepniak. 2019. “Impact of Gold Nanoparticles Shape on Their Cytotoxicity against Human Osteoblast and Osteosarcoma in in Vitro Model. Evaluation of the Safety of Use and anti-Cancer Potential.” Journal of Materials Science: Materials in Medicine 30 (2): 22. https://doi.org/10.1007/s10856-019-6221-2
- Suman, T. Y., and D.-S. Pei. 2022. “Nanomaterial Waste Management". In Nanomaterials Recycling, edited by Rai, M., Nguyen T. A. 21–36. Elsevier. https://doi.org/10.1016/B978-0-323-90982-2.00002-0
- Tak, Y. K., S. Pal, P. K. Naoghare, S. Rangasamy, and J. M. Song. 2015. “Shape-Dependent Skin Penetration of Silver Nanoparticles: Does It Really Matter?” Scientific Reports 5 (1): 16908. https://doi.org/10.1038/srep16908
- Tolonen, H., S. Moore, D. Lermen, A. Virgolino, L. E. Knudsen, A.-M. Andersson, L. Rambaud, C. Ancona, and M. Kolossa-Gehring. 2022. “What is Required to Combine Human Biomonitoring and Health Surveys?” International Journal of Hygiene and Environmental Health 242: 113964. https://doi.org/10.1016/j.ijheh.2022.113964
- Tsoli, M., H. Kuhn, W. Brandau, H. Esche, and G. Schmid. 2005. “Cellular Uptake and Toxicity of Au55 Clusters.” Small 1 (8-9): 841–844. https://doi.org/10.1002/smll.200500104
- Ursini, C. L., A. M. Fresegna, A. Ciervo, R. Maiello, V. Del Frate, G. Folesani, M. Galetti, et al. 2021. “Occupational Exposure to Graphene and Silica Nanoparticles. Part II: pilot Study to Identify a Panel of Sensitive Biomarkers of Genotoxic, Oxidative and Inflammatory Effects on Suitable Biological Matrices.” Nanotoxicology 15 (2): 223–237. https://doi.org/10.1080/17435390.2020.1850903
- Vena, M. P., M. Jobbágy, and S. A. Bilmes. 2016. “Microorganism Mediated Biosynthesis of Metal Chalcogenides; a Powerful Tool to Transform Toxic Effluents into Functional Nanomaterials.” The Science of the Total Environment 565: 804–810. https://doi.org/10.1016/j.scitotenv.2016.04.019
- Vidmar, J., R. Milačič, V. Golja, S. Novak, and J. Ščančar. 2016. “Optimization of the Procedure for Efficient Dispersion of Titanium Dioxide Nanoparticles in Aqueous Samples.” Analytical Methods 8 (5): 1194–1201. https://doi.org/10.1039/C5AY03305E
- Viegas, S., Zare Jeddi, M. B., Hopf, N., Bessems, J., Palmen, N., Galea, S., Jones, K., et al. 2020. “Biomonitoring as an Underused Exposure Assessment Tool in Occupational Safety and Health Context-Challenges and Way Forward.” International Journal of Environmental Research and Public Health 17 (16): 5884. https://doi.org/10.3390/ijerph17165884
- Vladitsi, M., C. Nikolaou, N. P. Kalogiouri, and V. F. Samanidou. 2022. “Analytical Methods for Nanomaterial Determination in Biological Matrices.” Methods and Protocols 5 (4): 61. https://doi.org/10.3390/mps5040061
- Vorkamp, K., A. Castaño, J.-P. Antignac, L. D. Boada, E. Cequier, A. Covaci, M. Esteban López, et al. 2021. “Biomarkers, Matrices and Analytical Methods Targeting Human Exposure to Chemicals Selected for a European Human Biomonitoring Initiative.” Environment International 146: 106082. https://doi.org/10.1016/j.envint.2020.106082
- White, E. 2011. “Measurement Error in Biomarkers: sources, Assessment, and Impact on Studies.” IARC Scientific Publications 163: 143–161.
- World Health Organization (WHO). 2015. Human Biomonitoring: facts and Figures. Copenhagen: WHO Regional Office for Europe.
- Williams, R. M., J. Shah, B. D. Ng, D. R. Minton, L. J. Gudas, C. Y. Park, and D. A. Heller. 2015. “Mesoscale Nanoparticles Selectively Target the Renal Proximal Tubule Epithelium.” Nano Letters 15 (4): 2358–2364. https://doi.org/10.1021/nl504610d
- Wiśniewska, P., J. Haponiuk, M. R. Saeb, N. Rabiee, and S. A. Bencherif. 2023. “Mitigating Metal-Organic Framework (MOF) Toxicity for Biomedical Applications.” Chemical Engineering Journal 471: 144400. https://doi.org/10.1016/j.cej.2023.144400
- Witzler, M., F. Küllmer, and K. Günther. 2018. “Validating a Single-Particle ICP-MS Method to Measure Nanoparticles in Human Whole Blood for Nanotoxicology.” Analytical Letters 51 (4): 587–599. https://doi.org/10.1080/00032719.2017.1327538
- Worthington, K. L. S., A. Adamcakova-Dodd, A. Wongrakpanich, I. A. Mudunkotuwa, K. A. Mapuskar, V. B. Joshi, C. Allan Guymon, et al. 2013. “Chitosan Coating of Copper Nanoparticles Reduces in Vitro Toxicity and Increases Inflammation in the Lung.” Nanotechnology 24 (39): 395101. https://doi.org/10.1088/0957-4484/24/39/395101
- Wu, W.-T., L.-A. Li, T.-C. Tsou, S.-L. Wang, H.-L. Lee, T.-S. Shih, and S.-H. Liou. 2019. “Longitudinal Follow-up of Health Effects among Workers Handling Engineered Nanomaterials: A Panel Study.” Environmental Health 18 (1): 107. https://doi.org/10.1186/s12940-019-0542-y
- Wu, W.-T., H.-Y. Liao, Y.-T. Chung, W.-F. Li, T.-C. Tsou, L.-A. Li, M.-H. Lin, J.-J. Ho, T.-N. Wu, and S.-H. Liou. 2014. “Effect of Nanoparticles Exposure on Fractional Exhaled Nitric Oxide (FENO) in Workers Exposed to Nanomaterials.” International Journal of Molecular Sciences 15 (1): 878–894. https://doi.org/10.3390/ijms15010878
- Xie, X., J. Liao, X. Shao, Q. Li, and Y. Lin. 2017. “The Effect of Shape on Cellular Uptake of Gold Nanoparticles in the Forms of Stars, Rods, and Triangles.” Scientific Reports 7 (1): 3827. https://doi.org/10.1038/s41598-017-04229-z
- Yaqoob, A. A., Ahmad, H., Parveen, T., Ahmad, A., Oves, M., Ismail, I. M. I., Qari, H. A., Umar, K., and Mohamad Ibrahim, M. N. 2020. “Recent Advances in Metal Decorated Nanomaterials and Their Various Biological Applications: A Review.” Frontiers in Chemistry 8: 341. https://doi.org/10.3389/fchem.2020.00341
- Yildirimer, L., Thanh, N. T. K., Loizidou, M, and Seifalian, A. M. 2011. “Toxicology and Clinical Potential of Nanoparticles.” Nano Today 6 (6): 585–607. https://doi.org/10.1016/j.nantod.2011.10.001
- Yu, M., S. Huang, K. J. Yu, and A. M. Clyne. 2012a. “Dextran and Polymer Polyethylene Glycol (PEG) Coating Reduce Both 5 and 30 nm Iron Oxide Nanoparticle Cytotoxicity in 2D and 3D Cell Culture.” International Journal of Molecular Sciences 13 (5): 5554–5570. https://doi.org/10.3390/ijms13055554
- Yu, T., K. Greish, L. D. McGill, A. Ray, and H. Ghandehari. 2012b. “Influence of Geometry, Porosity, and Surface Characteristics of Silica Nanoparticles on Acute Toxicity: Their Vasculature Effect and Tolerance Threshold.” ACS Nano 6 (3): 2289–2301. https://doi.org/10.1021/nn2043803
- Zhang, Y., A. Bello, D. K. Ryan, P. Demokritou, and D. Bello. 2022. “Elevated Urinary Biomarkers of Oxidative Damage in Photocopier Operators following Acute and Chronic Exposures.” Nanomaterials 12 (4): 715. https://doi.org/10.3390/nano12040715
- Zhao, L., Y. Zhu, Z. Chen, H. Xu, J. Zhou, S. Tang, Z. Xu, et al. 2018. “Cardiopulmonary Effects Induced by Occupational Exposure to Titanium Dioxide Nanoparticles.” Nanotoxicology 12 (2): 169–184. https://doi.org/10.1080/17435390.2018.1425502
- Zoroddu, M., S. Medici, A. Ledda, V. Nurchi, J. Lachowicz, and M. Peana. 2014. “Toxicity of Nanoparticles.” Current Medicinal Chemistry 21 (33): 3837–3853. https://doi.org/10.2174/0929867321666140601162314