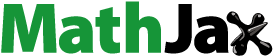
ABSTRACT
In this research, the effects of Selective Laser Melting (SLM) process parameters comprising laser power, scan speed, hatch space and scan pattern angle on the formation of porosity and subsequently density have been analysed. To improve the mechanical properties, post-processes (heat treatment) must be performed. Therefore, heat treatment was added to the design of experiment to analyse the effect of this process coupling with SLM process parameters on the value of density. A comprehensive design set with five levels for each parameter was selected so Taguchi L25 was used as the design of experiment. The significance of each parameter on obtained results was examined using statistical analysis (F-Test) and numerical model (interrogator analysis). The correlation between two process parameters was discussed by using 3D analytical and contour plots and the mechanisms behind these were discussed in depth. The contribution of this paper is a deep investigation of the relation of process parameters and heat treatment on density based on the Artificial Neural Network model. Results showed that better density is obtained with lower scan speed, laser power and scanning pattern angle. Meanwhile, for heat treatment and hatch space, the best density was obtained in their optimum range.
1. Introduction
Laser Powder Bed Fusion (LPBF) is one of the most common techniques of metal additive manufacturing (AM). This method has been successfully applied to a wide range of metals including steels, titanium alloys, aluminium alloys and nickel-based alloys with high flexibility in dimension and shape of the products. In particular, Ti-6Al-4 V alloy has been extensively used and practiced by the SLM technique for different applications (Gibson, Kvan, and Wai Ming Citation2002; Gibson, Rosen, and Stucker Citation2015; Yadroitsev and Yadroitsava Citation2015; Khorasani, Gibson, Asadnia et al. Citation2018; Sillars et al. Citation2018). Like other manufacturing techniques, the desire is to achieve optimum properties of the manufactured part through the correct adjustment of the processing parameters.
Although SLM is highly capable of manufacturing materials with high relative density, printing material without any porosity at all still is a challenge. Formation of the pores during the SLM process seems to be highly dependent on the flow of the melt, melting pool and its solidification rate (Qiu et al. Citation2015; Du Plessis et al. Citation2018) so the processing parameters that affect the size, depth and temperature of the melting pool are the key elements in controlling porosity.
It has been reported that for any specific power, the variation range of scan speed is limited due to balling effect (Averyanova, Bertrand, and Verquin Citation2011; Qiu et al. Citation2015). Unsuitable selection of laser power and scan speed causes instability of the melt flow that results in the formation of pores. It is believed that Marangoni convection and recoil pressure are the root causes of the instability of melt flow and pool (Khorasani, Gibson, and Ghaderi Citation2018). Investigating the influence of a wide range of processing parameters on the formation of pores, relative density and mechanical properties of SLM Ti-6Al-4 V alloy showed that mechanical properties are highly dependent on the printing orientation (Simonelli, Tse, and Tuck Citation2014; Sutton et al. Citation2017). Teng et al. (Citation2017) investigated porosity and cracking related defects as well as lack of fusion. The temperature gradient causes some metal to convert into the vapour state, which is trapped and forms a bubble, also known as a keyhole. One study showed that the keyhole defects are highly dependent on the speed of the laser and emphasizes that higher scan speeds reduce keyhole defects. This is related to the reduction of energy density and subsequently melting pool temperature, which are the source of vapor and keyhole (Teng et al. Citation2017).
Analysis of microstructure, layer-by-layer, showed major anisotropy of Young’s modulus and modest anisotropy of other mechanical properties (Jhabvala et al. Citation2010; Dadbakhsh, Hao, and Sewell Citation2012; Long et al. Citation2018). This can be related to the irregular orientation of the grains in different directions. Part layout directly affects the main variables, which are temperature distribution and cooling rate. These two factors, along with the gas flow, greatly affect the occurrence of defects such as porosity in SLM parts. Delamination is directly proportional to temperature distribution and gas flow. To reduce the chance of delamination, the temperature distribution should be kept relatively constant. It was observed that there is a stronger bond and fewer pores between layers when the gas flow is perpendicular compared to when the gas flow is parallel to the part. However, this is highly related to the shape of the sample.
Laser scanning speed and preheating temperature both have a significant effect on the depth of the melting pool. Preheating affects the height of the melt pool whereas scanning speed controls the width of the track. The preheating controls the contact angle and depth of the melt pool because adhesion between solid and molten metal is the cohesive force causing molten metal to spread out and forms the pores (Yadroitsev et al. Citation2013).
The density of powder metal with three different particle sizes was studied by Spierings, Herres, and Levy (Citation2011). Finer particles can be melted quickly, resulting in higher density. On the other hand, larger particles have a benefit of a greater breaking elongation, which is equivalent to the conventionally processed material. The scan velocity is typically determined according to the particle size distribution since it requires higher scan speed to melt the finer powder and slower speed to melt coarser particles. However, if the particle size is coarse without finer particles filling the gaps, the overall density of the part decreases and the surface roughness will increase.
To minimize the occurrence of porosity, the process parameters such as laser power, scan speed, hatch space, etc. are required to be optimized based on the geometry of the part, mechanical properties and chemical composition of the material. Moreover, the process parameters should be selected in proportion to each other.
Lack of comprehensive investigation on density was found in the literature that ties process parameters to density by discussing the rheology of the melting pool. Most of the literature revolved around small data sets which suffer from Gaussian errors and therefore an extensive study is needed to show the relationship between process parameters and the density of printed parts.
In this research, based on Taguchi L25 Design of Experiment (DoE), 25 samples with five repetitions were printed and evaluated for density measurement to show the effect of process parameters on the density. To improve mechanical properties, different standard heat treatments such as mill annealing, stress relieving, alpha-beta and beta annealing, were carried out. The effect of heat treatment coupled with process parameters produced interesting results, which are statistically explained. In the next step, the whole process was modelled using artificial neural networks (ANNs) and the interaction of each two process parameters on density is discussed through both ANN and statistical methods.
2. Experimental set-up
2.1. Powder material and SLM operation
Based on ASTM E8 and E8M, samples (using the smallest size) were printed using an SLM 125HL (SLM Solutions GmbH, Lubeck, Germany), equipped with YLR-Fiber-Laser. Parameters which are constant during the build process are shown in . shows powder morphology and as-built samples. Samples were printed in X-Y direction. Layer thickness was selected as 30 µm, min wall thickness 120 µm and operational beam focus was 100 µm.
Table 1. Process parameters and levels.
shows the SLM parameters and specifications.
2.2. Design of experiment
In the case of the full factorial DoE, the total test number is impossible to be performed in terms of cost and time. To reduce the test number, Taguchi DoE was used. Taguchi L25 DoE was used containing five factors and five levels for each. To analyze each factor independently, an orthogonal array was chosen, and shows the factors and levels.
2.3. Post-processing (Heat treatment)
During SLM, large thermal gradients, associated with cooling and heating processes, lead to the accumulation of residual stress and generation of martensitic and the results are lower ductility and higher tensile strength. To enhance mechanical properties and machinability, annealing is recommended (Yasa et al. Citation2010; Khorasani et al. Citation2016; Khorasani, Gibson, Goldberg, Littlefair Citation2017). shows the heat treatment conditions that were used in this experiment. The heating and resident time were each fixed at two hours, with the heating gradient gradually increasing from ambient to the set temperature at 4.8–8.6°C/min. To remove the cooling effect, the cooling rate was kept fixed at 5°C/min across all samples (Welsch, Boyer, and Collings Citation1993).
Table 2. Heat treatment condition (20 means no heat treatment).
2.4. Density measurement
To measure the value of density, we used electronic Archimedes densimeter SD-200L with density resolution of 0.0001 g/cm3 with solid volume and specific gravity of solid and liquid measurement in one unit. The scale capacity was 0.01–200 g (https://www.worldoftest.com/densimeter-sd-200l 2019). We used L25 DOE with five repetitions so there was a total of 125 samples and two measurements on each sample. In total, we analyzed 250 measurements to generate a comprehensive model.
3. Results
3.1. Interrogator analysis
Density is related to the overall quality of the printed parts. In other words, any problem within the process leads to the generation of defects such as trapped gas, unstable melting pool, lack of fusion, volcano effect, cracks, balling, etc. These defects, in general, are called porosity and affect density. Therefore, analyzing the process parameters for density can be helpful to characterize the SLM process and finding the optimum process window. The results were obtained according to our previous research into the relation of density, hardness, tensile strength and surface quality of as-built parts (Khorasani et al. Citation2019).
To identify the effect of each process parameter on the value of density, interrogator analysis was carried out. In order to serve this need, Multivariate Analysis of Variance (MANOVA) was implemented on the results. Also, ANN, which is normally used for modelling, was utilized to show the interrogator analysis. Qnet software with the capability of showing the effect of each input (process parameters) on outputs (Relative Density) was used (Vanloocke Citation1995).
In interrogator analysis, first, an ANN should be designed, trained and tested. Therefore, according to the condition of our test, a network with two hidden layers 5 × 4 × 3 × 1 was selected. shows the outputs of the network in both train and test, which proves that the accuracy of the designed network is in an acceptable range. The chosen number of samples in train, test and recall/validation were 15, 5 and 5 respectively with 4 × 106 iterations. Analytical results and graphs were used to verify the proposed ANN model (Khorasani et al. Citation2019).
Figure 2. Predictions results of designed network (The number of samples for train, test and recall were 15, 5 and 5 respectively).
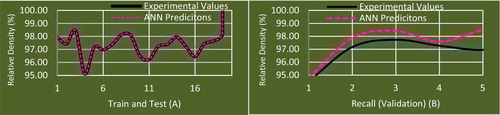
shows the interrogator analyses for five parameters. Also, MANOVA shows that of all the parameters interrogated, heat treatment had the highest influence on the value of density. Some other parameters could also be seen to influence density, thus highlighting the challenges of modelling. Analyzing F-tests in (B) shows that the difference in variance for heat treatment is significant, which confirms the ANN interrogator analysis is correct. The secondary influential factors were seen to be hatch space and scan speed, which showed similar impact in both F-Test and interrogator. Finally, laser power and scan pattern angle were found to be the least influential on the value of density. The comprehensive parametric mapping of relative density in SLMed Ti alloy based on the experiment and proposed model is shown in the Appendix.
4. Discussions
4.1. The effect of laser power versus other parameters on density using analytical and numerical analysis
We collected analytical graphs from the previous study (Khorasani et al. Citation2019) and discuss and compare them with the results of the proposed ANN. shows the analytical graphs for laser power versus other process parameters. These graphs were obtained from MANOVA. Similarly, shows the effect of laser versus other parameters based on ANN interrogator analysis. A similar trend in each sub-figure is observed and validates both analyses.
Figure 4. Interaction of laser power versus other parameters (analytical graphs) (Khorasani et al. Citation2019).
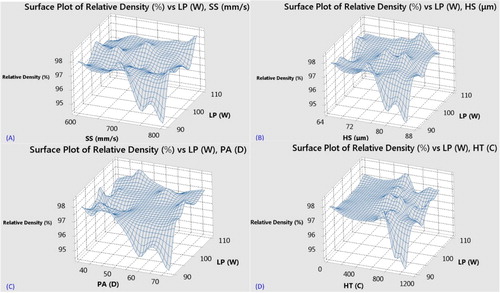
Figure 5. Interaction of laser power versus other parameters (numerical graphs) (RD is Relative density).
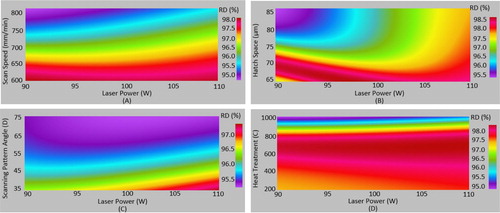
and (A) show that with high scan speed, less density was obtained. This is related to wettability and Rayleigh instability. According to Khorasani, Gibson, and Ghaderi (Citation2018), melting pool temperature is obtained through Equation (1).(1)
(1) Where C1 and C2 are Planck distribution constants and
is wavelength. In higher scan speed, according to Equation (1), melting pool temperature decreases and causes increasing surface tension based on Equation (2). This phenomenon is called thermocapillary effect (Berthier Citation2008; Malkin and Isayev Citation2017).
(2a)
(2a)
(2b)
(2b) Where
is constant for each liquid, TC is critical temperature, T0 is a reference value for temperature
are Plank distribution constants, SS is scan speed, HS is hatch space, LP is laser power and
is wavelength. (The indexes of S, L and G are representative of solid, liquid and gas interfaces). Therefore, the value of
becomes bigger and according to Equation (3), the chance of generation of droplets increases (Berthier Citation2008; Malkin and Isayev Citation2017; Khorasani, Gibson, and Ghaderi Citation2018). Surface tension is defined for triple contact line on the surface during printing process based on different interfaces including
. When the temperature of melting pool decreases, the surface tension in contact line with liquid becomes bigger; so, the value of
increase compared to surface tension in solid and gas interface (
). This is the condition of formation of droplets and is the driving factor of Rayleigh instability.
(3)
(3) The results are low wettability and a higher chance of balling, which is known to be a common defect in metal AM and increases pores and reduces density (Tian et al. Citation2017; Khorasani, Gibson, and Ghaderi Citation2018). (A) shows that with lower laser power, less density is obtained. However, this figure has some fluctuations, which in turn causes fluctuations in density. (A) is more linear in terms of variations and shows that increasing laser power improves density. Laser power and scan speed play important roles in energy density and in some areas of this diagram by increasing both factors the energy density reduces. Therefore, due to lack of fusion, lower density was obtained. (C) and (D) have less fluctuations due to the lower effect of scanning pattern angle and absence of heat treatment below 600°C.
When using lower laser power according to Equation (1), less energy is transferred to the samples and the melting pool temperature decreases. The results are big pores that are often called lack of fusion (), and density decreases (Kok et al. Citation2015; Calignano Citation2018). (A) proved that the scan speed is more influential on the density.
and (B) illustrate that with lower laser power, less density was obtained down to 95%, which is also explained as a lack of fusion and energy. With hatch space, the density trend is different. Small hatch space starts with lower density associated with high overlap. By increasing hatch space, density improves to an optimum value and then decreases. In the overlap area, the material is mushy and the value of micro-flow motion due to high viscosity is zero. Therefore, no Marangoni’s convection is happening and heat penetration increases with the chance of formation of keyholes. shows large pores due to lack of energy density in the bulk of the sample.
Lower density for high hatch space can be related to higher energy dispersion in a wider area that leads to lower remelting of the subsequent layer. and (C) shows that the pattern angle affects the density. Increasing pattern angle leads to decreasing density. The reason is related to higher overlap between subsequent layers. In other words, lower pattern angles experience higher thermal penetration of subsequent layers. More re-melting therefore happens and keyholes that were generated on overlap areas will disappear or become smaller. The result is increasing density and improvement in the quality of printed parts.
and (D) shows the small variation of density before 600°C (mill annealing), which is related to the selected temperatures for heat treatment (there was no heat treatment between 20°C and 600°C). These figures show that with heat treatment up to 700°C, or without heat treatment, the best density was obtained. This can be related to the formation of due to the high cooling rate (Murr et al. Citation2009; Khorasani et al. Citation2016; Khorasani, Gibson, Goldberg, and Littlefair Citation2017; Khorasani, Gibson, and Ghaderi Citation2018). This phase disappears in mill annealing and stress relieving. Furthermore, due to the lower mass of
, density improves. Over this range, the density shows to have a reduction specifically for temperature above 1000°C. At this temperature, as shown in , micro flow might be occurring on the surface that is associated with temperature concentration and lower mass due to balling and residual particles (Khorasani et al. Citation2016; Khorasani, Gibson, Goldberg, Masoud Movahedi et al. Citation2017; Khorasani, Gibson, Goldberg, and Littlefair Citation2017).
SLM is operated under controlled conditions (first vacuum, then argon). However, small amounts of nitrogen and oxygen were reported in the bulk of material so the solution of these elements in titanium may result in decreasing melting point and is another reason for increasing micro-flow during heat treatment on 1050°C (Baufeld, Van der Biest, and Gault Citation2010; Gorny et al. Citation2011). Previous research (Khorasani, Gibson, and Ghaderi Citation2018) showed that in 1050°C heat treatment, the value of average surface ‘Sa’ greatly increased.
4.2. The effect of scan speed versus other parameters on density
and (A) show that lower density was obtained for higher scan speed and hatch space. Lack of wettability and energy in this region results in increasing pores and reduction of density to 95%. (A) shows more linear behaviour due to the numerical solution of the ANN method. It illustrates that with lower scan speed and when hatch space increases, density has an initially raising and then decreasing trend. With higher scan speed and increasing hatch space density, there is only a decreasing trend. With the lowest scan speed and hatch spacing the pressure in the droplet, according to Equation (4), increases and due to thermocapillary effect, the surface tension also increases and more bursting happens, which is a fertile ground for porosity in the subsequent layers (Khorasani, Gibson, and Ghaderi Citation2018).(4)
(4) When increasing hatch space, the energy density is moved to the optimum area and less overlap leads to higher density. Above this optimum area, energy density decreases, and wider hatch spacing leads to less laser penetration and re-melting, and so lower density was obtained.
Figure 8. Interaction of scan speed versus other parameters (analytical graphs) (Khorasani et al. Citation2019).
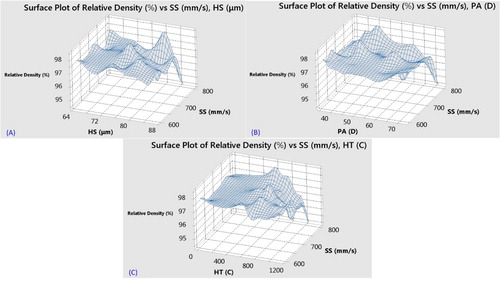
Figure 9. Interaction of scan speed versus other parameters (numerical graphs) (RD is Relative density).
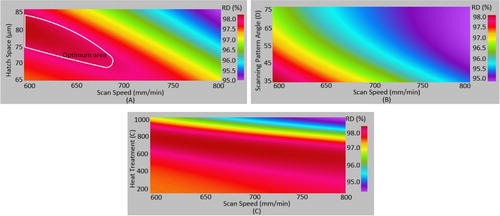
and (B) show a similar trend for scan speed. Besides that, lower density is seen with bigger pattern angles, which is related to the above-mentioned mechanisms. Analytical observation from (B) demonstrates that some density peaks occurred in the cases of high scan speed and low pattern angle. This is related to better coverage of overlaps for former layers that leads to more remelting, which generates better density. This effect seems to compensate the lack of wettability in higher scan speeds. and (C) illustrate that better density was obtained with heat treatment ranging from 600°C to 800°C. The combination of micro-flow at higher temperature (>900°C) and lack of wetting causes decreasing density. Moreover, porosity in higher scan speed duplicates this chance of micro-flow and reduces the density to 95%. (C) shows that the effect of heat treatment on density is stronger than scan speed and this is confirmed by F-Test and interrogator analysis.
4.3. The effect of hatch space versus other parameters on density
and (A) illustrate that the best density was obtained with hatch space around 70 µm and low pattern angle. This figure proves that hatch space is a more influential parameter on density. Another reason for higher density with lower hatch spacing is related to the work of adhesion. In solid–liquid interfaces like melting pool and solidified layer, Young’s law leads to Young-Dupre's relation and Equation (5) shows that work adhesion is directly related to surface tension.(5)
(5) where θ is the angle of the tangent to meniscus curve relative to normal line to the surface of the melt pool. Based on Planck’s distribution and thermocapillary effect when selecting small hatch space, the surface tension and work adhesion in hatch to hatch decreases and this phenomenon leads to decreasing initial adhesion between solidified layer to melting pool. Furthermore, due to laser pulses, the melt pool becomes unstable and the gradient of fluid flow has non-zero values (Equation (6)):
(6)
(6) Where
is the speed of fluid. This leads to increasing waves and incorporation of unmelted powder particles which may form porosity in subsequent layers.
Figure 10. Interaction of scan speed versus other parameters (analytical graphs) (Khorasani et al. Citation2019).
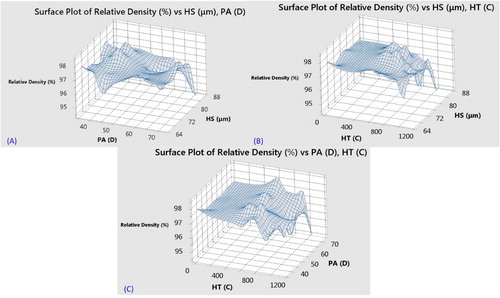
Figure 11. Interaction of scan speed versus other parameters (numerical graphs) (RD is Relative density).
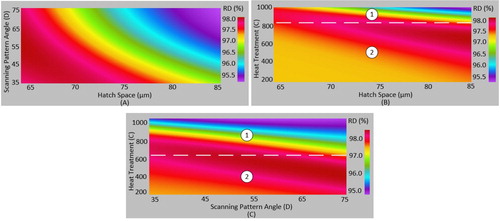
and (B) show a similar trend of density for heat treatment versus other process parameters. Mill annealing gives the best density and in higher temperature and hatch the density and strength radically reduces (Facchini et al. Citation2010; Simonelli, Tse, and Tuck Citation2014; Cao et al. Citation2018). Also, this figure shows that increasing hatch space up to a certain point (region 2 (B)) improves density. Larger hatch space makes greater contact between the melting pool and solidified layer. Therefore, according to the following equations, surface energy and subsequently work adhesion increases.(7)
(7)
(8)
(8) Where SC is contact area of melting pool with a solidified layer, E1 is melting pool energy and E2 is Energy of solidified layer.
The result is a more stable melting pool and higher density. Higher than a certain amount (region 1 (B)) due to a stronger effect of micro-fluid flow, increasing hatch space generates less density (Welsch, Boyer, and Collings Citation1993; Jovanović et al. Citation2006; Sieniawski et al. Citation2013; Li et al. Citation2015; Khorasani, Gibson, and Ghaderi Citation2018). Larger overlap area is obtained in smaller hatch space and it is reported that highly overlapping areas absorb much energy. For less overlap, most of the absorbed heat during heat treatment is spent on the center of the tracks and leads to greater microflow and reduction of density towards 95%.
and (C) show that heat treatment had the greatest impact on density compared to scan pattern angle. A similar trend is seen when comparing this figure with the interaction of hatch space and pattern angle. However, the area of reduction of density (region 1) is wider compared to (C). This is related to the higher contribution of hatch space on density () compared to pattern angle.
5. Conclusions
In this work, the effect of SLM process parameters including laser power, scan speed, hatch space and scan pattern angle on melting pool, porosity and relative density of Ti-6Al-4V parts was investigated. To improve mechanical properties, heat treatment is necessary for SLM parts. Thus, different heat treatments such as mill annealing, stress relieving, alpha-beta annealing and beta annealing were carried out and the effect of heat treatment on density was also analyzed. The results are summarized as below:
The most effective parameter on porosity is heat treatment that is confirmed by F-test and interrogator analysis. Scan speed and hatch space have almost the same impact on porosity and the lowest impact is related to laser power and pattern angle.
Due to low wettability and Rayleigh instability, when increasing scan speed, the porosity increases.
When using lower laser power, the energy density is smaller and the melting pool temperature decreases. Large pores that are referred to as lack of fusion is a result of this phenomenon.
Smaller hatch space generates lower density and is related to higher overlap area and by increasing hatch space density subsequently increases to an optimum value and then decreases from there. This behaviour is related to mushy material in the overlap area and zero gradient of micro-flow motion due to higher viscosity. Therefore, the absence of Marangoni’s convection leads to higher temperature and penetration and the chance for the formation of keyholes increases. The area for lower density in the highest hatch space is associated with higher energy dispersion in wider hatch space and less remelting of the subsequent layers.
Increasing scan pattern angle causes decreasing density that is related to lower overlap covered by bigger pattern angles.
For samples with no heat treatment or up to 700°C, the best density was achieved. In beta annealing, micro flow might be occurring on the surface that is associated with temperature concentration and lower localized mass due to balling and residual particles.
Disclosure statement
No potential conflict of interest was reported by the authors.
Notes on contributors
Amir Mahyar Khorasani received his PhD in Additive manufacturing of metal parts from Deakin University in 2017. He is working on the process and post process of AM parts. Currently, he is Alfred Deakin Postdoctoral Research Fellow at Deakin University.
Ian Gibson is a professor of Additive manufacturing and works in the University of Twente and he is the head of Fraunhofer Production Center in the Netherlands. He is also working at Deakin University Australia. Ian works on different research topics including metal and polymer AM products.
AmirHossein Ghasemi is a Master of Engineering in Manufacturing and metal cutting. He is working as a trainer and lecturer in University of Applied Sciences.
Alireza Ghaderi received his PhD in Materials Engineering from Deakin University in 2011. He is a former research engineer at Deakin University where he worked on different research topics including plastic deformation, thermomechanical processing, and additive manufacturing of metal alloys as well as tribology and wear resistance of steels. He currently works at 3M in St Paul, USA as a surface scientist.
References
- Averyanova, M., P. Bertrand, and B. Verquin. 2011. “Studying the Influence of Initial Powder Characteristics on the Properties of Final Parts Manufactured by the Selective Laser Melting Technology: A Detailed Study on the Influence of the Initial Properties of Various Martensitic Stainless Steel Powders on the Final Microstructures and Mechanical Properties of Parts Manufactured Using an Optimized SLM Process is Reported in this Paper.” Virtual and Physical Prototyping 6 (4): 215–223. doi: 10.1080/17452759.2011.594645
- Baufeld, B., O. Van der Biest, and R. Gault. 2010. “Additive Manufacturing of Ti–6Al–4 V Components by Shaped Metal Deposition: Microstructure and Mechanical Properties.” Materials & Design 31: S106–S111. doi: 10.1016/j.matdes.2009.11.032
- Berthier, J. 2008. Micro-drops and Digital Microfluidics. Norwich, NY: William Andrew.
- Calignano, F. 2018. “Investigation of the Accuracy and Roughness in the Laser Powder Bed Fusion Process.” Virtual and Physical Prototyping 13 (2): 97–104. doi: 10.1080/17452759.2018.1426368
- Cao, S., Ruikun Chu, Xigen Zhou, Kun Yang, Qingbo Jia, Chao Voon Samuel Lim, Aijun Huang, and Xinhua Wu. 2018. “Role of Martensite Decomposition in Tensile Properties of Selective Laser Melted Ti-6Al-4V.” Journal of Alloys and Compounds 744: 357–363. doi: 10.1016/j.jallcom.2018.02.111
- Dadbakhsh, S., L. Hao, and N. Sewell. 2012. “Effect of Selective Laser Melting Layout on the Quality of Stainless Steel Parts.” Rapid Prototyping Journal 18 (3): 241–249. doi: 10.1108/13552541211218216
- Du Plessis, A., I. Yadroitsava, I. Yadroitsev, S. G. le Roux, and D. C. Blaine. 2018. “Numerical Comparison of Lattice Unit Cell Designs for Medical Implants by Additive Manufacturing.” Virtual and Physical Prototyping 13 (4): 266–281. doi: 10.1080/17452759.2018.1491713
- Facchini, L., Emanuele Magalini, Pierfrancesco Robotti, Alberto Molinari, Simon Höges, and Konrad Wissenbach. 2010. “Ductility of a Ti-6Al-4V Alloy Produced by Selective Laser Melting of Prealloyed Powders.” Rapid Prototyping Journal 16 (6): 450–459. doi: 10.1108/13552541011083371
- Gibson, I., T. Kvan, and L. Wai Ming. 2002. “Rapid Prototyping for Architectural Models.” Rapid Prototyping Journal 8 (2): 91–95. doi: 10.1108/13552540210420961
- Gibson, I., D. W. Rosen, and B. Stucker. 2015. Additive Manufacturing Technologies. New York: Springer.
- Gorny, B., T. Niendorf, J. Lackmann, M. Thoene, T. Troester, and H. J. Maier. 2011. “In situ Characterization of the Deformation and Failure Behavior of Non-stochastic Porous Structures Processed by Selective Laser Melting.” Materials Science and Engineering: A 528 (27): 7962–7967. doi: 10.1016/j.msea.2011.07.026
- Jhabvala, J., Eric Boillat, Thibaud Antignac, and Rémy Glardon. 2010. “On the Effect of Scanning Strategies in the Selective Laser Melting Process.” Virtual and Physical Prototyping 5 (2): 99–109. doi: 10.1080/17452751003688368
- Jovanović, M. T., S. Tadić, S. Zec, Z. Mišković, and I. Bobić. 2006. “The Effect of Annealing Temperatures and Cooling Rates on Microstructure and Mechanical Properties of Investment Cast Ti–6Al–4V Alloy.” Materials & Design 27 (3): 192–199. doi: 10.1016/j.matdes.2004.10.017
- Khorasani, A. M., Ian Gibson, Mohsen Asadnia, and William O’Neill. 2018. “Mass Transfer and Flow in Additive Manufacturing of a Spherical Component.” The International Journal of Advanced Manufacturing Technology 96 (9–12): 3711–3718. doi: 10.1007/s00170-017-1483-7
- Khorasani, A. M., Ian Gibson, Umar Shafique Awan, and Alireza Ghaderi. 2019. “The Effect of SLM Process Parameters on Density, Hardness, Tensile Strength and Surface Quality of Ti-6Al-4V.” Additive Manufacturing 25: 176–186. doi: 10.1016/j.addma.2018.09.002
- Khorasani, A. M., I. Gibson, and A. R. Ghaderi. 2018. “Rheological Characterization of Process Parameters Influence on Surface Quality of Ti-6Al-4V Parts Manufactured by Selective Laser Melting.” The International Journal of Advanced Manufacturing Technology 97 (9–12): 3761–3775.
- Khorasani, A. M., Ian Gibson, Moshe Goldberg, and Guy Littlefair. 2016. “A Survey on Mechanisms and Critical Parameters on Solidification of Selective Laser Melting During Fabrication of Ti-6Al-4V Prosthetic Acetabular Cup.” Materials & Design 103: 348–355. doi: 10.1016/j.matdes.2016.04.074
- Khorasani, A. M., Ian Gibson, Moshe Goldberg, and Guy Littlefair. 2017. “Production of Ti-6Al-4 V Acetabular Shell Using Selective Laser Melting: Possible Limitations in Fabrication.” Rapid Prototyping Journal 23 (1): 110–121. doi: 10.1108/RPJ-11-2015-0159
- Khorasani, A. M., Ian Gibson, Moshe Goldberg, Mohammad Masoud Movahedi, and Guy Littlefair. 2017. “Thermal Stress Flow Analysis in Fabrication of Acetabular Shells using SLM.” KnE Engineering 2 (2): 297–307. doi: 10.18502/keg.v2i2.629
- Kok, Y., Xipeng Tan, Shu Beng Tor, and Chee Kai Chua. 2015. “Fabrication and Microstructural Characterisation of Additive Manufactured Ti-6Al-4 V Parts by Electron Beam Melting: This Paper Reports that the Microstructure and Micro-hardness of an EMB Part is Thickness Dependent.” Virtual and Physical Prototyping 10 (1): 13–21. doi: 10.1080/17452759.2015.1008643
- Li, X. P., M. Roberts, Y. J. Liu, C. W. Kang, H. Huang, and T. B. Sercombe. 2015. “Effect of Substrate Temperature on the Interface Bond Between Support and Substrate During Selective Laser Melting of Al–Ni–Y–Co–La Metallic Glass.” Materials & Design (1980–2015) 65: 1–6. doi: 10.1016/j.matdes.2014.08.065
- Long, T., Xiaohong Zhang, Qianli Huang, Ling Liu, Yong Liu, Junye Ren, Yong Yin, Dengke Wu, and Hong Wu. 2018. “Novel Mg-based Alloys by Selective Laser Melting for Biomedical Applications: Microstructure Evolution, Microhardness and in vitro Degradation Behaviour.” Virtual and Physical Prototyping 13 (2): 71–81. doi: 10.1080/17452759.2017.1411662
- Malkin, A. Y., and A. I. Isayev. 2017. Rheology: Concepts, Methods, and Applications. ChemTec Publishing, Elsevier.
- Murr, L., S. A. Quinones, S. M. Gaytan, M. I. Lopez, A. Rodela, E. Y. Martinez, D. H. Hernandez, E. Martinez, F. Medina, and R.B. Wicker. 2009. “Microstructure and Mechanical Behavior of Ti–6Al–4 V Produced by Rapid-layer Manufacturing, for Biomedical Applications.” Journal of the Mechanical Behavior of Biomedical Materials 2 (1): 20–32. doi: 10.1016/j.jmbbm.2008.05.004
- Qiu, C., Chinnapat Panwisawas, Mark Ward, Hector C. Basoalto, Jeffery W. Brooks, and Moataz M. Attallah. 2015. “On the Role of Melt Flow into the Surface Structure and Porosity Development During Selective Laser Melting.” Acta Materialia 96: 72–79. doi: 10.1016/j.actamat.2015.06.004
- Sieniawski, J., W. Ziaja, K. Kubiak, and M. Motyka. 2013. Microstructure and Mechanical Properties of High Strength Two-phase Titanium Alloys, in Titanium Alloys-advances in Properties Control. InTech.
- Sillars, S. A., C. J. Sutcliffe, A. M. Philo, S. G. R. Brown, J. Sienz, and N. P. Lavery. 2018. “The Three-prong Method: A Novel Assessment of Residual Stress in Laser Powder Bed Fusion.” Virtual and Physical Prototyping 13 (1): 20–25. doi: 10.1080/17452759.2017.1392682
- Simonelli, M., Y. Y. Tse, and C. Tuck. 2014. “Effect of the Build Orientation on the Mechanical Properties and Fracture Modes of SLM Ti–6Al–4 V.” Materials Science and Engineering: A 616: 1–11. doi: 10.1016/j.msea.2014.07.086
- Spierings, A. B., N. Herres, and G. Levy. 2011. “Influence of the particle size distribution on surface quality and mechanical properties in AM steel parts.” Rapid Prototyping Journal 17 (3): 195–202. doi: 10.1108/13552541111124770
- Sutton, A. T., Caitlin S. Kriewall, Ming C. Leu, and Joseph W. Newkirk. 2017. “Powder Characterisation Techniques and Effects of Powder Characteristics on Part Properties in Powder-bed Fusion Processes.” Virtual and Physical Prototyping 12 (1): 3–29. doi: 10.1080/17452759.2016.1250605
- Teng, C., Deepankar Pal, Haijun Gong, Kai Zeng, Kevin Briggs, Nachiket Patil, and Brent Stucker. 2017. “A Review of Defect Modeling in Laser Material Processing.” Additive Manufacturing 14: 137–147. doi: 10.1016/j.addma.2016.10.009
- Tian, Y., Dacian Tomus, Paul Rometsch, and Xinhua Wu. 2017. “Influences of Processing Parameters on Surface Roughness of Hastelloy X Produced by Selective Laser Melting.” Additive Manufacturing 13: 103–112. doi: 10.1016/j.addma.2016.10.010
- Vanloocke, P. R. 1995. “Qnet-A Quantum-mechanical Neural-network-A New Connectionist Architecture and Its Relevance for Variable Binding and Constraint Satisfaction Problems.” Cybernetica 38 (1): 85–106.
- Welsch, G., R. Boyer, and E. Collings. 1993. Materials Properties Handbook: Titanium Alloys. ASM international.
- Yadroitsev, I., P. Krakhmalev, I. Yadroitsava, S. Johansson, and I. Smurov. 2013. “Energy Input Effect on Morphology and Microstructure of Selective Laser Melting Single Track from Metallic Powder.” Journal of Materials Processing Technology 213 (4): 606–613. doi: 10.1016/j.jmatprotec.2012.11.014
- Yadroitsev, I., and I. Yadroitsava. 2015. “Evaluation of Residual Stress in Stainless Steel 316L and Ti6Al4 V Samples Produced by Selective Laser Melting.” Virtual and Physical Prototyping 10 (2): 67–76. doi: 10.1080/17452759.2015.1026045
- Yasa, E., Jan Deckers, Jean-Pierre Kruth, Marleen Rombouts, and Jan Luyten. 2010. “Charpy Impact Testing of Metallic Selective Laser Melting Parts.” Virtual and Physical Prototyping 5 (2): 89–98. doi: 10.1080/17452751003703894